Abstract
Free full text

Reprogramming the genetic code: The emerging role of ribosomal frameshifting in regulating cellular gene expression
Abstract
Reading frame maintenance is a critical property of ribosomes. However, a number of genetic elements have been described that can induce ribosomes to shift on mRNAs, the most well understood of which are a class that directs ribosomal slippage by one base in 5' (‐1) direction. This is referred to as programmed ‐1 ribosomal frameshifting (‐1 PRF). Recently, a new ‐1 PRF promoting element was serendipitously discovered in a study examining the effects of stretches of adenosines in the coding sequences of mRNAs. Here, we discuss this finding, recent studies describing how ‐1 PRF is used to control gene expression in eukaryotes, and how ‐1 PRF is itself regulated. The implications of dysregulation of ‐1 PRF on human health are examined, as are possible new areas in which novel ‐1 PRF promoting elements might be discovered.
Also watch the https://youtu.be/1mPXIINCRcY.
Abstract
A) Traditional ‐1 PRF: ribosomes paused by mRNA structures shift on slippery sites. Regulated my miRNAs. B) polyA mediated ‐1 PRF: poly‐lysine may make ribosomes pause, where they slip back on polyA tracts. Regulation by polyA binding protein? C) Shifted ribosomes encounter premature termination codons, triggering mRNA degradation through NMD.
Abbreviations
- ‐1 PRF
- programmed ‐1 ribosomal frameshifting
- NMD
- nonsense‐mediated mRNA decay
- NSD
- “non‐stop” decay
- PTC
- premature termination codon
Introduction
We are living in a golden age of scientific discovery, from the detection of exoplanets and the near certainty of extraterrestrial life, to the visualization of atomic scale molecular machines in action. While new findings tend to be consistent with generally accepted theories, they also reveal interesting exceptions to the general rules. In so doing, they both further illuminate these rules, and help to reveal the deeper mysteries of the natural world. In this essay we discuss how the discovery of cis‐acting mRNA elements that subvert normal mRNA decoding is adding a new dimension to our understanding of how cellular gene expression is regulated.
A quick tutorial on protein synthesis
mRNAs are decoded by ribosomes as groups of three contiguous nucleotides (codons) on messenger RNAs (mRNAs). Each codon specifies either an amino acid or, in three cases, instructions to stop protein synthesis. Ribosomes are programmed to identify the right place to start (initiation, usually at an AUG codon encoding methionine in eukaryotes and archaea and formyl‐methionine in prokaryotes), and then proceed to decode the genetic information in the mRNA by marching (elongation) down the mRNA in the 5′ to 3′ direction 1 codon at a time until they reach one of the stop codons (termination). Central to this is that ribosomes must maintain the reading frame as defined by the initiation codon, in order to properly decode the information contained in the mRNA. Translational reading frame maintenance is an under‐appreciated area of research as compared to a large body of literature on e.g. mechanisms underlying tRNA charging by aminoacyl‐tRNA synthetases, or the accurate decoding of codons 1. From this, it follows that bad things must happen when ribosomes fail to maintain reading frame. And indeed, this is true … with some exceptions.
PolyA tracts and mRNA decay
To explore these exceptions, we begin with a recent paper by Arthur and co‐workers 2 describing why consecutive runs of A residues rarely occur in the protein coding regions of mRNAs, and their surprising elucidation of how such polyA tracts are used to regulate gene expression. Prior studies in nucleated (eukaryotic) cells had shown that if ribosomes bypass a normal stop codon they becomes stalled on the mRNA's polyA tail. This is presumably due to the fact that the AAA codon encodes the basic amino acid lysine, and the demonstrated ability of poly‐basic polypeptides such as poly‐lysine promote ribosome stalling 3, 4, presumably through their interactions with the negatively charged ribosomal exit tunnel 5. The stalled ribosomes are recognized by a complex of proteins that remove the ribosome for recycling 6. During this process, the complex also recruits an endonuclease which cleaves the mRNA and initiates its degradation. This is called “non‐stop” decay (NSD) because the failure of ribosomes to stop where they are supposed to results in destruction of the mRNA (reviewed in 7). While on the subject of mRNA decay, two additional mechanisms merit discussion. A second is initiated when ribosome stalling occurs in the protein coding sequence, typically due to strong mRNA structures that are difficult to unwind. Here, the same (or similar) machinery is recruited to save the ribosome and kill the message: this is called “no‐go” decay (NGD) 8, 9. Thirdly, if a ribosome encounters a stop codon in the wrong context e.g. far away from a polyA tail (called a premature termination codon, or PTC), mRNA degradation proceeds through an independent process called the nonsense‐mediated mRNA decay pathway (NMD) (recently reviewed in 10). Note that although the ribosome is at fault in these cases, it is the messenger that pays the price.
Arthur and co‐workers mined sequence data to find that runs of polybasic amino acids are universally underrepresented in the protein coding regions of mRNAs. This engendered the hypothesis that these might be used as regulatory elements by acting as “translational attenuators” akin to the NSD process. Surprisingly however, only runs of polyA, but not repeated AAG codons (which also encodes poly‐lysine) or repeated CGA or AGG (encoding poly‐arginine), conferred strong translational attenuation effects. Thus, something other than basic amino acid mediated ribosome stalling had to be operating.
Slip sliding away
Thirty years ago, the HIV/AIDS epidemic was dominating the headlines and the virus was just beginning to be characterized. Elucidation of retroviral genomic sequences by many different groups revealed a novel feature: overlapping and mutually out of frame open reading frames. This soon led to the discovery of special “slippery” sequences able to program elongating ribosomes to slip from one reading frame to another in a process that is called programmed ‐1 ribosomal frameshifting (‐1 PRF) 11. In the intervening years, we and others have characterized the nature of these ‐1 PRF signals, demonstrating the importance of ‐1 PRF on virus propagation by ensuring synthesis of the correct stoichiometries of viral proteins 12, 13, 14. More recently, it has been recognized that ribosomal frameshifting and other forms of translational recoding are widely used in all three domains of life 15, 16, 17, 18. Here, we focus on ‐1 PRF in eukaryotes, where computational searches for “classic” ‐1 PRF signals suggested that up to 10% of genes may be regulated by this mechanism 19. Counterintuitively, unlike viruses in which ‐1 PRF is used to synthesize two (or more) proteins from a single mRNA (Fig. (Fig.1A),1A), more than 99% of ‐1 PRF events were predicted to direct elongating ribosomes to PTCs (Fig. (Fig.1B).1B). Follow up studies revealed that these “genomic” ‐1 PRF signals function as mRNA destabilizing elements through NMD from yeast to humans 20, 21, 22. Thus, while ‐1 PRF generally serves to expand the genomic coding content of viruses, in eukaryotes it appears to be primarily employed as a post‐transcriptional regulatory mechanism. However, while classic ‐1 PRF signals require slippery stretches of nucleotides, slippage is greatly stimulated by strong downstream RNA structural elements that induce elongating ribosomes to pause at the slippery sequences. Interestingly, a strong RNA secondary structural element can also promote significant rates of ‐1 PRF even in absence of an upstream canonical slippery site, albeit to a lesser extent 22. Whether the stimulatory elements actively help ribosomes to slip, or passively enhance kinetic partitioning between reading frames remains to be determined. We and others have also recently shown that ‐1 PRF can be regulated in a sequence‐specific manner through interaction of ‐1 PRF signals with trans‐acting nucleic acids e.g. naturally occurring miRNAs and synthetic oligonucleotides 21, 23, 24, 25. However, while polyA is one of the allowable “slippery” sequences within the coding region of an mRNA, the surprising finding was that it can direct efficient ‐1 PRF in the absence of any other stimulating element. Indeed, Arthur and colleagues demonstrated that as few as 9 A's in a row were able to promote a significant fraction of ribosomes to shift reading frame. In the context of naturally occurring polyA sequences, these were shown to direct ribosomes to PTCs, destabilizing mRNAs through NMD, thus limiting protein expression. The ability of these short polyA sequences to promote frameshifting at rates of ~10% in the absence of a downstream stimulatory structural element is rather surprising. While not discussed by the authors, we suggest that the presence of poly‐lysine in the ribosome exit tunnel may cause ribosomes to pause over the slippery polyA sequence, thus enhancing their ability to kinetically partition into the ‐1 frame. If so, this suggests that at least two different ‐1 PRF mechanisms convergently evolved as mRNA destabilizing elements to control gene expression in eukaryotic cells. Additionally, Gene Ontogeny analysis of polyA track containing messages identified by Arthur et al. reveals that approximately 12% are located in mRNAs encoding trans‐acting regulatory factors involved in stress response and apoptosis. While this suggests a regulatory role for these sequences, no suggestion for how such regulation may be effected has been presented. In addition, the fact that polyA sequences do not promote slippage into the +1 frame is also interesting: while not discussed by the authors, perhaps this observation is instructive about the fundamental nature of how ribosomes naturally maintain reading frame.
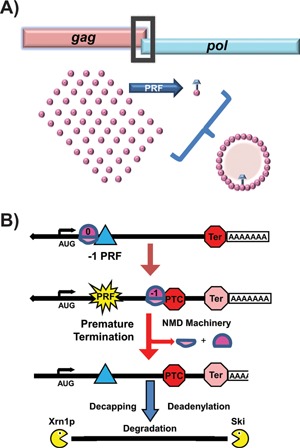
Frameshifting on viral compared to cellular mRNAs. A: In viruses, PRF events result in synthesis of C‐terminally extended fusion proteins. In many virus families (e.g. Retroviridae, Totiviridae), rates of ‐1 PRF determine the stoichiometric ratios of capsid (pink Gag) to replicase (pink+
blue Gag‐pol) proteins. Correct ratios are critical for viral particle assembly. B: Canonical “genomic” ‐1 PRF signals or poly(A) tracks (blue triangle) can direct an elongating ribosome to a ‐1 frame premature termination codon (PTC). The recognition of the PTC by the ribosome results in activation of nonsense mediated mRNA decay (NMD) pathway and subsequent degradation of the transcript through a process of decapping and deadenylation followed by exonucleolytic degradation by Xrn1p (5′→3′) and the Ski complex (3′→5′).
Regulation of gene expression by ‐1 PRF
In our studies, we have validated ‐1 PRF signals in mRNAs encoding proteins involved in numerous cellular processes, including telomere maintenance 22 and the immune response 21. Critically, the availability of a set of sequences that direct ‐1 PRF at rates ranging from 1 to 70% enabled the relationship between rates of ‐1 PRF and mRNA abundance to be determined:
where x=
‐1 PRF efficiency and mRNA abundance is a function (f) of x (Fig. (Fig.2).2). Since this is an inverse exponential relationship, small changes in frameshifting can have large effects on gene expression. This suggests that ‐1 PRF is a translational attenuation mechanism that functions to balance gene dosage. Intriguingly, most ‐1 PRF signals promote 1–10% frameshifting: this lies in the linear range of the plot where changes in ‐1 PRF efficiencies are predicted to promote the largest changes in mRNA abundances.
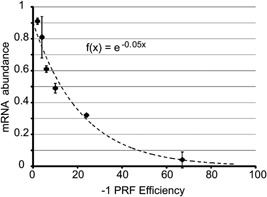
The relationship between frameshift efficiency and mRNA abundance. The mathematical relationship between ‐1 PRF efficiency and mRNA abundance was determined using frameshift signals derived from the yeast EST1, EST2, STN1, and CDC13 mRNAs. These were cloned into a reporter gene and cellular mRNA steady‐state abundances were plotted relative to the abundance of the reporter without frameshift signals. Plotting of these data fit to the logarithmic function f(x)=
e−0.05x
(adapted from 22).
What might be the consequences of perturbing this delicate balance? In elegant studies using B. subtilis, Losick and co‐workers have shown that switching between two morphological cell types is controlled not by the absolute numbers of regulatory molecules, but by their relative ratios 26. This represents an important paradigmatic change: rather the Galilean quantitative numerical emphasis, it returns our understanding of biological regulation to the classical ratiometric Euclidian view. Curiously, included in the first report of yeast mutants in which ‐1 PRF was globally elevated was the observation that many of the mutants had temperature‐dependent cell cycle defects 27. Twenty years later, the basis for this was elucidated: in yeast, telomere maintenance is controlled by ‐1 PRF 22. Global increases in ‐1 PRF imbalances the stoichiometries of key proteins that are either intrinsic to telomerase itself (Est1p and Est2p), or involved in telomerase recruitment to chromosome ends (Stn1p, Cdc13p). We hypothesize changes in the relative ratios of these proteins inhibits telomerase activity and/or recruitment to chromosome ends, triggering “checkpoint arrest” as cells work to repair this particular DNA synthesis defect before committing to cell division. This accounts for the observed cell cycle arrest phenotypes upon global dysregulation of ‐1 PRF. Notably, this phenomenon was also observed upon inhibition of NMD, consistent with the epistatic relationship of NMD to ‐1 PRF. Importantly, this places pressure on cells to select for mutations that bypass this quality control checkpoint, most commonly by “repairing” telomeres using the double stranded break repair apparatus. Unfortunately, this approach requires templating by homologous sequences, i.e. the ends of other chromosomes, which can lead to chromosome fusion, breakage during mitosis, and eventually aneuploidy. Unpublished finding from our laboratory have recently validated ‐1 PRF signals in some human mRNAs encoding telomere maintenance proteins, suggesting the evolutionary conservation of ‐1 PRF in chromosome end maintenance.
Bad ribosomes
As noted above, we estimate that ~10% of nuclear encoded mRNAs harbor classic ‐1 PRF signals, and another ~2% harbor slippery polyA sequences; a respectable number of genes. Thus, it follows that defects in ‐1 PRF due to defective ribosomes may be significant drivers of disease. The importance of ribosomes and human health has emerged over the past decade through the emergence of a class of diseases collectively called Ribosomopathies 28. Originally associated with genetically inherited anemias, it is becoming clear that mutations that affect ribosome biogenesis and/or ribosome function have dire consequences. Interestingly, while patients with classic ribosomopathies such as Shwachman–Bodian–Diamond syndrome and X‐linked dyskeratosis congenita (X‐DC) initially present with hypo‐proliferative cellular disorders e.g. anemias, should they survive this phase of the disease, they are at much higher risk of developing cancers of the same tissue bed, i.e. cellular hyper‐proliferation. This seeming paradox is known in the field of hematology as Dameshek's Riddle 29. Using X‐DC as a model, we showed that rRNA hypopseudouridylation decreases the affinity of ribosomes for at least two classes of RNA ligands, internal ribosomal entry signals (IRES) and tRNAs 30. While this leads to decreased expression of IRES containing mRNAs (emerging as an important driver of cancer 31), this biochemical defect also renders tRNAs more likely to slip at ‐1 PRF signals. The effects of X‐DC associated mutations on ‐1 PRF and gene expression is currently a topic of intense research. Unpublished findings from our laboratory also suggests that mutations associated with other classic ribosomopathies affect various aspects of translational fidelity, including ‐1 PRF, and hence gene expression.
An understanding of how somatically acquired mutations that affect translational reading frame maintenance may also underlie other cancers is beginning to take shape. Exome sequencing initially revealed a conserved mutation in the gene encoding ribosomal protein L10 (also known as uL16 32) in ~10% of T‐cell acute lymphoblastic leukemias 33, and followup studies revealed that this mutation promotes globally increased rates of ‐1 PRF 34, 35. Current efforts are aimed at characterizing how this affects gene expression and drives carcinogenesis. Additionally, while ribosomopathies are currently defined as diseases caused by defective ribosomes, it is not inconceivable that mutations that affect other components of the translational apparatus may also be pathogenic. Case in point: spinocerebellar ataxia 26 (SCA26) is an autosomal dominant disease caused by a mutation in EEF2, encoding the translational translocase eukaryotic elongation factor 2 (eEF2), which promotes increased rates of ‐1 PRF both in yeast 36, and in patient‐derived cells (unpublished). Altered rates of ribosomal slippage may not only affect gene expression by altering mRNA abundances. The increased expression of C‐terminally truncated dead end polypeptides may also burden the cellular protein degradation apparatus. Indeed, yeast cells harboring the EEF2‐SCA26 mutation show a more robust unfolded protein response (UPR) induction in response to antioxidant and heat shock challenges 36. This may be of importance with regard to neurological disease in particular as Purkinje neurons are known to be vulnerable to a wide variety of molecular and cellular insults 37. The links between ‐1 PRF and proteostaic insult represent a critical, underexplored area of investigation. Additionally, unlike the stringent quality control mechanisms governing ribosome biogenesis 38, no such control governing trans‐acting factors like eEF2 are known. Thus, diseases associated with mutations in this class of proteins should be rare and may be expected to present as hypoproliferative only.
Sequence‐specific regulation of frameshifting
If ‐1 PRF is widely used to control gene expression, then it stands to reason that it should be subject to regulation. However, given that global changes in ‐1 PRF appear to be deleterious, regulation of ‐1 PRF would have to be sequence‐specific. microRNAs (miRNAs) and other non‐coding RNAs naturally participate in sequence‐specific interactions with mRNAs, and thus present the logical places to look for trans‐acting regulators of individual ‐1 PRF signals. With this in mind, computational methods were used to identify two miRNAs that interact with and stimulate ‐1 PRF promoted by a sequence element in the mRNA encoding CCR5, a cytokine receptor that is used as a co‐receptor for HIV‐1 21. A series of genetic and biochemical experiments revealed that one of these, miR‐1224, directly interacts with the CCR5 ‐1 PRF stimulating mRNA pseudoknot. Presumably, the interaction stabilizes the pseudoknot, rendering it more difficult for ribosomes to resolve. This would increase ribosome pause times at the slippery site, stimulating kinetic partitioning into the ‐1 frame. Theoretically, miRNAs may also have ‐1 PRF inhibitory activities by being able to destabilize ‐1 PRF promoting mRNA downstream elements. In support of this, siRNA knockdown of the cellular miRNA processing apparatus stimulated ‐1 PRF promoted by some frameshift signals, and inhibited ‐1 PRF promoted by others 21. Non‐coding RNA stimulation of ‐1 PRF at polyA sequences may also be possible. In support of this, hybridization of antisense linked nucleic acids (LNAs) immediately 3′ of heptameric slippery sequences was sufficient to promote efficient ‐1 frameshifting in the absence of any other stimulatory element 39. Natural attenuation of ‐1 PRF by stem‐loop structures immediately 5′ of coronavirus slippery sequences has also been reported 40, a phenomenon that can be replicated by hybridization of oligonucleotides complementary to sequences lying just upstream of slippery sites 41. These studies establish the role of ncRNAs in regulating translational fidelity and suggest that regulation of ‐1 PRF may not be limited to miRNAs. Missing, however, is any hypothesis or model explaining how polyA‐directed ‐1 PRF may be specifically regulated. The demonstration of protein‐induced transactivation of frameshifting in porcine reproductive and respiratory syndrome virus 42 may provide a clue in this respect; perhaps polyA‐mediated ‐1 PRF may be regulated by polyA‐binding proteins.
Conclusion and prospective
Arthur and co‐workers serendipitously discovered that there is more than one way to program ‐1 ribosomal frameshifting. What other ribosomal frameshift promoting elements may be out there, and how might they be identified? An approach for discovering new ‐1 PRF signals that has worked particularly well with viruses and bacteria has been to first detect evolutionarily conserved reading frames and then, using molecular genetics tools, identify and characterize the translational recoding elements 16. Mining of ribosome profiling data to pinpoint frameshifted ribosomes, and from there identifying the elements that made them shift reading frame presents a new and promising approach 21, 43. The recent discovery of modified bases in mRNAs and their regulation 44, 45, raises the question of how these may affect the ability of ribosomes to maintain reading frame. Additionally, although we have known about mRNA editing for quite some time (reviewed in 46), its role in creating or ablating ‐1 PRF signals remains completely unexplored. Furthermore, identifying the trans‐acting factors (miRNAs, other ncRNAs and even proteins) that regulate specific ‐1 PRF signals, characterizing how their expression is regulated, and how dysregulation may be linked to disease is another open research area. Lastly, as outlined in Fig. Fig.3,3, ‐1 PRF and NMD present as therapeutic targets, not only with regard to viral diseases, e.g. HIV/AIDS, but also as a potential modality to fine tune and correct errors in gene expression, either using small molecules that target specific ‐1 PRF signals to correct the expression of specific genes (e.g. using synthetic RNA‐like molecules) or using therapeutics that correct for changes in gene expression due to global defects in ‐1 PRF.
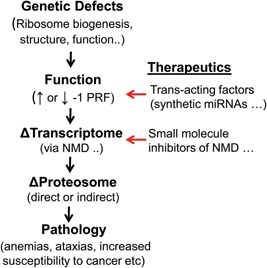
From genes to disease and points of therapeutic intervention. Mutations in genes that participate in translation that alter global rates of ‐1 PRF elicit downstream post‐transcriptional surveillance pathways e.g. NMD that alter the transcriptome. This leads to altered gene expression (proteomic changes) and progression to disease states. Therapeutic approaches may include use of synthetic polynucleotide analogs (miRNAs and related derivitives) targeting specific ‐1 PRF signals designed to fine tune frameshifting rates. Given the epistatic relationship of NMD to ‐1 PRF, targeting this pathway using small molecule inhibitors presents another therapeutic modality.
The authors have declared no conflict of interest.
Acknowledgements
We would like to thank our co‐workers, in particular Susan Baserga, Kim de Keersmaecker, Arlen Johnson, Davide Ruggero, John Woolford, Ian Brierley, John Atkins, and many others in the translational control field for participating in stimulating and creative discussions about the topics covered in this essay in an ongoing discussion over the past two decades. The discussion of Dr. Losick's findings in the context of Galilean versus Euclidian mathematical schemes was inspired by lectures by Drs. Losick and Mark A. Peterson (Department of Mathematics, Mount Holyoke College). We also wish to acknowledge members of the Dinman lab, past and present. This work was supported by grant to JDD from the National Institutes of Health (R01 HL119439, R01 GM117177).
References
Full text links
Read article at publisher's site: https://doi.org/10.1002/bies.201500131
Read article for free, from open access legal sources, via Unpaywall:
https://www.ncbi.nlm.nih.gov/pmc/articles/PMC4749135
Citations & impact
Impact metrics
Article citations
Alternative production of pro-death Bax∆2 protein via ribosomal frameshift in Alzheimer's disease.
Sci Rep, 14(1):27288, 08 Nov 2024
Cited by: 0 articles | PMID: 39516502 | PMCID: PMC11549088
Modular Polymerase Synthesis and Internal Protein Domain Swapping via Dual Opposed Frameshifts in the Ebola Virus L Gene.
Pathogens, 13(10):829, 25 Sep 2024
Cited by: 0 articles | PMID: 39452701 | PMCID: PMC11510084
Possible involvement of three-stemmed pseudoknots in regulating translational initiation in human mRNAs.
PLoS One, 19(7):e0307541, 22 Jul 2024
Cited by: 0 articles | PMID: 39038036 | PMCID: PMC11262651
Ribosome specialization in cancer: a spotlight on ribosomal proteins.
NAR Cancer, 6(3):zcae029, 09 Jul 2024
Cited by: 1 article | PMID: 38989007
Review
The Inhibition of Gag-Pol Expression by the Restriction Factor Shiftless Is Dispensable for the Restriction of HIV-1 Infection.
Viruses, 16(4):583, 10 Apr 2024
Cited by: 2 articles | PMID: 38675925 | PMCID: PMC11055011
Go to all (38) article citations
Other citations
Similar Articles
To arrive at the top five similar articles we use a word-weighted algorithm to compare words from the Title and Abstract of each citation.
Cell cycle control (and more) by programmed -1 ribosomal frameshifting: implications for disease and therapeutics.
Cell Cycle, 14(2):172-178, 01 Jan 2015
Cited by: 20 articles | PMID: 25584829 | PMCID: PMC4615106
Mechanisms and implications of programmed translational frameshifting.
Wiley Interdiscip Rev RNA, 3(5):661-673, 19 Jun 2012
Cited by: 136 articles | PMID: 22715123 | PMCID: PMC3419312
Review Free full text in Europe PMC
Reprogramming eukaryotic translation with ligand-responsive synthetic RNA switches.
Nat Methods, 13(5):453-458, 21 Mar 2016
Cited by: 20 articles | PMID: 26999002 | PMCID: PMC4850110
Ribosome collisions alter frameshifting at translational reprogramming motifs in bacterial mRNAs.
Proc Natl Acad Sci U S A, 116(43):21769-21779, 07 Oct 2019
Cited by: 35 articles | PMID: 31591196 | PMCID: PMC6815119
Funding
Funders who supported this work.
NHLBI NIH HHS (1)
Grant ID: R01 HL119439
NIGMS NIH HHS (1)
Grant ID: R01 GM117177
National Institutes of Health (2)
Grant ID: R01 GM117177
Grant ID: R01 HL119439