Abstract
Free full text

In Vivo Imaging of Human Neuroinflammation
Introduction
The central nervous system (CNS) enacts a series of responses to promote homeostasis, both in pathological (e.g., neurotropic infections, neurodegenerative and autoimmune disorders1–3), and physiological (e.g. aging4) conditions. This series of responses, comprehensively referred to as “neuroinflammation”, encompasses the concerted actions of numerous cellular and molecular mechanisms designed to identify the potentially harmful event, limit its impact, and repair any consequent damage. Neuroinflammatory responses do not occur solely in the presence of a local insult (e.g., a stroke), but also when normal functioning of the CNS is challenged by distally occurring pathological events. For instance, immunocompetent CNS cells can detect pathologically enhanced afferent neuronal activity occurring after peripheral injury. In this case, neuroinflammation is termed ‘neurogenic’5, 6. Just like classical neuroinflammation, neurogenic neuroinflammation can be adaptive. For example, the CNS neuroinflammation induced by peripheral injury often results in increased pain sensitivity (hyperalgesia and allodynia), which motivates the organism to rest, thereby promoting recovery from the original insult7. However, when exaggerated, both types of neuroinflammation can become pathological, and are in fact implicated in the pathophysiology of a growing number of human disorders3, 8–10.
Because of the recent understanding that neuroinflammation is involved in various neuropathologies, interest in the development of novel methods to investigate neuroinflammatory processes, for the purpose of diagnosis and therapy monitoring, as well as to guide development of novel treatments, has surged over the past 15 years. Neuroimaging offers a wide array of non- or minimally-invasive techniques to characterize neuroinflammatory processes in vivo (Figure 1). The intent of this review is to provide a brief overview of some of the currently available methods to image neuroinflammation in the human CNS. Nuclear imaging (positron emission tomography [PET]; single photon emission computed tomography [SPECT]) and magnetic resonance imaging (MRI) technologies afford broad capability to image many different biological processes, and imaging equipment is widely accessible. For these reasons, we will focus on strategies utilizing nuclear and MRI technologies.
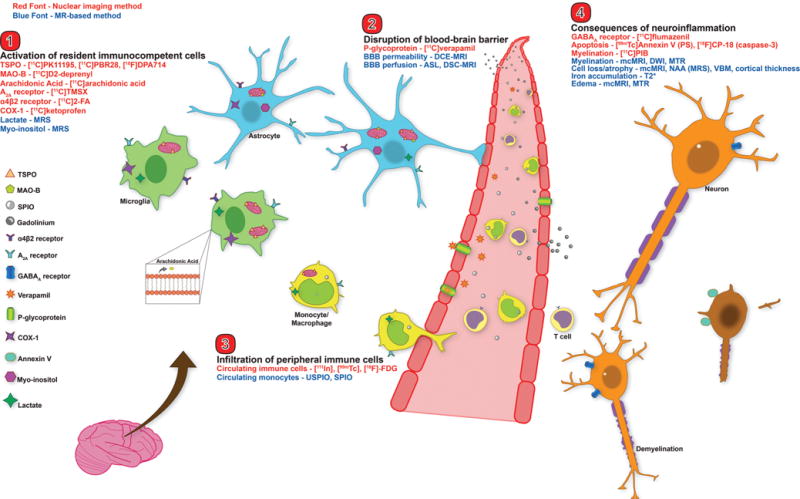
Neuroimaging targets for the major players in neuroinflammation. This figure depicts an overview of the major nuclear imaging and MRI tools to study human neuroinflammation. Nuclear imaging methods are displayed in red font, MRI methods in blue font. Categories are broken into: 1) activation of CNS immunocompetent cells (microglia and astrocytes), 2) disruption of BBB, 3) infiltration of peripheral immune cells, and 4) consequences of neuroinflammation (e.g. demyelination and cell death). TSPO – translocator protein 18kDa; MAO-B – monoamine oxidase B; SPIO – superparamagnetic iron oxide particle; USPIO – ultrasmall SPIO; COX-1 – cyclooxygenase-1; MRS – magnetic resonance spectroscopy; DCE – dynamic contrast enhanced; DSC – dynamic susceptibility contrast; ASL – arterial spin labeling; BBB – blood brain barrier; PS – phosphatidylserine; mcMRI – multiple contrast MRI; DWI – diffusion weighted imaging; MTR – magnetization transfer ratio; NAA – N-acetyl aspartate; VBM – voxel based morphometry.
Both nuclear and MR-based methods offer their own strengths and weaknesses. In general, nuclear imaging possesses superior specificity, as it images actual molecules that have been radiolableled. Meticulously developed and validated radioligands, paired with sophisticated data modeling, give nuclear imaging the ability to detect and quantify many molecular targets, often at nanomolar concentrations. Unlike nuclear methods, MRI involves no ionizing radiation, and therefore is particularly amenable to certain study designs, e.g., longitudinal studies with repeated imaging visits. Moreover, MRI possesses unique flexibility, as the same basic physical principle can be employed to measure different properties of imaged tissues (concentration of specific metabolites, perfusion, anatomical and functional properties, etc). Of course, an ideal approach is to combine imaging modalities, in order to capitalize on their complementary strengths.
Importantly, neuroinflammation is characterized by a large repertoire of local and distant responses, which can differ as a function of the type, intensity, and duration of a given proinflammatory event. For instance, increased blood-brain barrier (BBB) permeability, often considered one of the cardinal neuroinflammatory responses, is a hallmark of actively inflammatory focal lesions in multiple sclerosis (MS)11 and of ongoing inflammation in Alzheimer’s Disease (AD)12. Yet, it is unclear whether BBB compromise contributes to other conditions also thought to be characterized by neuroinflammation, such as schizophrenia8. Regardless of the specific underlying cause of the neuroinflammatory response, many of the processes are overlapping (e.g. glial activation is a factor in all three neuropathologies). The aim of this review is not to provide an exhaustive description of every neuroinflammatory element, which has been discussed extensively2, 6, 13. Rather, we will focus on methods to image in vivo biological mechanisms that are generally recognized as major players of neuroinflammation in human CNS disorders. Specifically, this review will focus on neuroimaging methods that target 1) activation of CNS immunocompetent cells, 2) compromised BBB, 3) CNS-infiltration of circulating immune cells, and 4) pathological consequences of neuroinflammation (e.g. demyelination and cell death). The relative breadth of the literature on each of these four neuroinflammatory topics will be reflected in the length of the section.
Previous reviews have discussed multitudes of potential neuroimaging targets to study neuroinflammation14, 15. However, the vast majority of these tools are currently available only for preclinical imaging, and a large percentage will never become available for human use. Additionally, observations from animal models of disease often do not translate into efficacious treatments for patients, despite very promising results at the preclinical level16. This discrepancy likely results from a combination of factors, including experimental bias, lack of negative result reports, and interspecies differences in neuroimmune targets17, 18. Therefore, this review will focus only on neuroimaging techniques that are currently available for use in human clinical research.
Imaging resident immunocompetent cells
The first line of response to an event that challenges the CNS is the activation of resident immunocompetent cells, i.e. microglia and astrocytes. Typically, although not in all circumstances19, microglia are activated first, initiating morphological changes and expression and release of pro-inflammatory mediators and other signaling molecules13. These molecules initiate astrocyte activation, leading to further release of inflammatory molecules, and influence the excitability of neurons. Homeostatic glial activation can beneficial in dealing with minor tissue dysfunction, but it can also become pathogenic if the activation is persistent20. Though oligodendrocytes have recently been suggested to participate in immune responses21, imaging methods are currently only available for microglia and astrocyte imaging in humans, and thus we will focus on techniques targeting these cells. Future efforts will hopefully lead to cell-specific imaging strategies for all CNS immunocompetent cells.
Nuclear imaging methods
TSPO
The translocator protein 18kDa (TSPO) is a five transmembrane domain protein, mainly situated in the outer mitochondrial membrane. TSPO is thought to be involved in a wide array of vital cellular functions, including steroidogenesis, mitochondrial respiration, and cellular proliferation, among others22. However, some of these functions have been the focus of several recent studies employing TSPO knockout models. Results from these studies have been equivocal, as two models suggested generally normal physiological functioning and development in knockout animals23, 24, while a third indicated that TSPO is important for both embryo viability and steroidogenesis25. Though the specific physiological function of TSPO is still a controversial subject, its features made it the molecule of choice for most PET imaging studies aimed at imaging glial activation and neuroinflammation. In the healthy CNS, TSPO is constitutively expressed by multiple cell types, including glia and neurons, but only at low levels26. During inflammatory responses, however, TSPO is substantially upregulated predominantly, if not exclusively, in glial cells27. For instance, in two animal models of neuropathic pain28, 29, ~5% of neurons and microglia, and ~30% of astrocytes expressed TSPO in the spinal cord dorsal horn prior to surgery. One week after surgery, the number of TSPO-positive neurons did not increase, whereas TSPO-positive microglia and astrocytes increased ~7-fold and ~3-fold, respectively. The colocalization of TSPO upregulation and activated glia has been observed in many animal models and human disorders30, but the specific cell type imaged by TPSO remains a large point of contention in the literature. Many studies describe TSPO tracers as markers of microglial activation, despite evidence for upregulation in astrocytes following many CNS challenges, as described above. The relative contribution of specific glial cells to the TSPO signal is likely a function of several factors, including the pathology and timecourse investigated. In some rat models of MS, TSPO upregulation is localized to microglia31, 32, whereas both astrocytes and microglia are involved in other models and human post-mortem MS data33–35, as well as in human and rodent models of ischemia35, 36 and AD33, 35, 37. As mentioned above, initial microglial activation is often followed by a delayed and prolonged astrocytic activation, although the opposite pattern has been observed in some cases29. Altogether, these observations highlight that TSPO is a marker for “glial” activation, but its ability to specifically image microglia or astrocytes may vary across studies.
Since its first use in the 1980’s, [11C]PK11195 (PK) has been the most widely used TSPO imaging agent (for a detailed review of PK, see38). Although PK imaging yielded a great deal of information on glial activation in CNS disorders, its use is suboptimal. PK has low brain penetrance and high nonspecific binding. These features limit signal to background ratio (SBR) and ability to detect subtle PET signals related to glial activation. For this reason, and given the great interest in imaging TSPO as a glial marker, a great deal of effort has recently gone into developing improved radioligands for this protein. Several of these ligands are approved for human imaging, including [11C]PBR28, [18F]DPA-714, [18F]FEPPA, [11C]DAA1106, [18F]PBR06, and [18F]PBR111 (reviewed in 22). Second-generation radioligands possess much higher SBR than PK39, and have been used to image glial activation in a number of pathologies, including brain tumors, trauma, psychiatric disorders, and chronic pain10, 40–45 (Figure 2). While second-generation TSPO ligands exhibit several advantages over PK, quantitative interpretation of their signal is confounded by the existence of two separate binding sites, one with high affinity and one with low affinity46. Differential expression of binding sites between subjects results into a tri-modal distribution of binding affinity. For instance, among people of European ancestry, ~50% express only high affinity sites (high-affinity binders; HABs), ~10% express only low affinity sites (low-affinity binders LABs), and ~40% express approximately equal numbers of high- and low-affinity sites (mixed-affinity binders; MAB)39, 47. In principle, these binding affinity differences pose potential interpretative issues, because signal differences across groups may not reflect genuine differences in glial activation, but rather binding affinity differences. Fortunately, it was recently demonstrated that a single nucleotide polymorphism (SNP) in the TSPO gene (Ala147Thr)48 fully predicts the binding affinity. Therefore, scientists can now account for global differences in binding affinity through a variety of methods, including restricting recruitment to only subjects exhibiting one genotype, using matched-pairs design, including TSPO status in the statistical model, etc39.
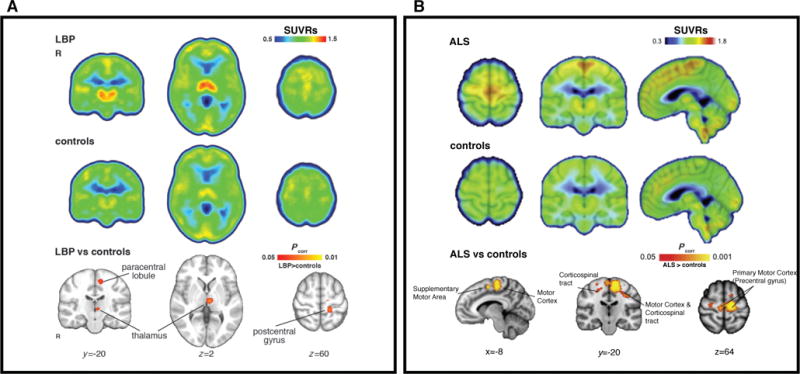
Elevated [11C]PBR28 binding in patients with chronic low back pain44 (cLBP; A) and amyotrophic lateral sclerosis42 (ALS; B) compared to healthy controls. All SUVRs represent standardized uptake value (SUV) normalized by whole-brain uptake. For both cLBP and ALS patients, regional increases in glial activation correspond to brain regions implicated in disease pathology. A) Median SUVR images for cLBP patients (top row) and controls (middle row). Bottom row – clusters are regions where SUVR in cLBP patients was significantly higher than controls. B) Mean SUVR images for ALS patients (top row) and controls (middle row), overlaid on average structural MRI. Bottom row – clusters represent voxels where ALS SUVR was significantly higher than controls. Modified from Loggia et al. “Evidence for brain glial activation in chronic pain patients”, Brain published online Jan 12. DOI: 10.1093/brain/awu37744, with permission from Oxford University Press, and Zurcher et al. “Increased in vivo glial activation in patients with amyotrophic lateral sclerosis: Assessed with [11C]-PBR28”, NeuroImage:Clinical 7: 409–414, DOI:10.1016/j.nicl.2015.01.00942, with permission from ScienceDirect under the terms of Creative Commons Attribution-NonCommercial-No Derivatives License (CC BY NC ND; http://creativecommons.org/licenses/by-nc-nd/4.0/).
Another important issue to consider when designing a TSPO study is estimation of specific binding. The gold standard for PET quantification is kinetic modeling with an arterial plasma input function and correction for plasma free fraction (fP; fraction of tracer unbound to plasma proteins) yielding distribution volume (Vt). However, this is not as straightforward for TSPO ligands as for some other radioligands. Many studies using arterial sampling for kinetic modeling of TSPO reported substantial variability in Vt even within groups, for both first- and second-generation radioligands. For PK, this may be partially due to high binding to a plasma protein that is altered in inflammatory states49. Similar tracer binding to inflammation-susceptible plasma protein is possible, but undocumented, for second-generation ligands. Presumably, measurement of fP should account for tracer binding to plasma protein, but fP values also vary widely, likely due to methodological differences and measurement inaccuracies. In addition to blood variability, estimated Vt is highly variable within and across studies. This may be associated with inaccurate blood measurement, but high vascular TSPO binding has also been proposed to significantly affect outcome estimation50.
Because absolute quantitation is challenging, several groups have estimated TSPO binding with alternative methods, including reference tissue modeling and the use of semi-quantitative measures like standardized uptake value (SUV). Reference tissue modeling, when validated, is a suitable alternative to an arterial plasma input function51, 52. Unlike many of the neurotransmitter system tracers, there is no brain region devoid of TSPO expression, and thus a true reference region is unavailable. However, several alternatives exist. For PK, supervised clustering methods have identified reference tissue53. While these methods may be inappropriate for second-generation ligands54, a recent study with [11C]PBR28 showed that estimation of binding with a cerebellar pseudo-reference region was less variable than estimation with plasma activity, and better distinguished Alzheimer’s Disease (AD) patients from controls55. Blood-free methods like this are very attractive, due to difficulties in accurate plasma measurement described above, and patient discomfort related to arterial line placement. Normalization by within-subject PET signal has the additional benefit of partly correcting for global signal differences introduced by the TSPO genotype39, 40, 42, 44, 56. However, the use of such methods must be carefully validated both for the ligand in question and the study population.
MAO-B
Monoamine oxidase is an important enzyme that exists in two sub-types: MAO-A and MAO-B. MAO-B is more relevant for studies of neuroinflammation, due to its upregulation in reactive astrocytes in certain neuroinflammatory conditions like AD57. Selective MAO-B antagonists have been radiolabeled for PET imaging of astrocytes, including [11C]-D-deprenyl and its deuterium substituted analog, [11C]-deprenyl-D258, 59. [11C]-deprenyl-D2 is the most commonly used astrocyte tracer, because of its favorable kinetics compared to [11C]deprenyl. However, specific binding of the molecule has been questioned60. MAO-B radioligands have been used to image astrogliosis in several neuroinflammatory conditions, including AD61 and amyotrophic lateral sclerosis (ALS)62, among others. Researchers wishing to use MAO-B tracers should be aware of several limitations. MAO-B expression is not selective to astrocytes, it is also present in serotonergic and histaminergic neurons63, making interpretation of findings difficult. Additionally, the exact cellular binding of MAO-B tracers in human brain is poorly understood. Overall, MAO-B PET studies can provide useful information on astrocyte function in human neuropathology, but proper study design and cautious interpretation of results is important.
Cyclooxygenase
Cyclooxygenase-1 and -2 (COX-1 and -2) catalyze conversion of arachidonic acid to prostaglandins, which are important inflammatory signaling molecules. As for TSPO, different cell types constitutively express COX-1, and COX-2, which are upregulated following CNS insult64. There is differential upregulation depending on the type of insult and experimental model, but in general COX-1 is thought to be mostly upregulated in microglia65. COX-2 tracers are currently unavailable for human brain imaging, and thus will not be discussed here. The utility of COX-1 tracers is supported by the results of a rodent study with [11C]ketoprofen66, a COX-1 radioligand whose methyl esterified form ([11C]KTP-ME) is available for human use (but so far utilized only in a single study in healthy volunteers)67. The rodent study reported selective microglial upregulation of COX-1 following injection of inflammatory agents, but despite claims of microglial selectivity, other studies documented astrocytic changes in COX-1 expression following inflammatory challenges68, 69. These discrepancies may be a function of investigational models and techniques, as studies showing astrocytic changes in COX-1 were typically conducted in glial cell cultures, as opposed to brain tissue. Further research is needed to validate the selectivity and general utility of COX-1 tracers for human disorders, and to develop COX-2 tracers for human neuroimaging.
Arachidonic Acid
As mentioned above, arachidonic acid (AA) plays a major role in inflammatory signaling, via release from membrane glycerophospholipids and conversion to prostaglandins and leukotrienes70. Because of this, arachidonic acid is a potential imaging target for neuroinflammation studies, in spite of nonspecific cellular localization. Recently, a PET study using [11C]-labeled AA reported increased AA metabolism throughout most of the brain in AD patients71, which the authors interpreted as evidence for neuroinflammation. However, AA signaling is important for multiple aspects of healthy brain function. For instance, [11C]AA imaging has also shown cortical increases in AA metabolism as a result visual stimulation71, implicating AA involvement in neurotransmission. Therefore, AA imaging encompasses varying cellular processes, and it should be used cautiously as a marker of neuroinflammation. In addition to COX and AA, other molecules involved in the AA signaling pathway may also be useful for imaging neuroinflammation. Fatty acid amide hydrolase (FAAH) is one such molecule that has been implicated in neuroinflammation and human FAAH tracers are available72.
Adenosine Receptors
Several adenosine receptors are involved in neuroinflammatory signaling, but their specific actions during a neuroinflammatory response are not well elucidated. However, the A2A receptor (A2AR) in particular is involved in crucial neuroinflammatory processes, including LPS-induced changes in synaptic plasticity73, and microglial process retraction during activation74. Previous imaging studies assessed A2AR expression in several human neurological disorders, including PD and schizophrenia (reviewed in 75), but only one study interpreted increased A2AR expression as evidence of neuroinflammation76. Using [11C]TMSX to image secondary progressive MS patients, the authors showed increased A2AR binding in normal-appearing white matter (NAWM), which is known to contain activated glia1. They also reported a negative association between tracer binding and MR indices of white matter integrity, implicating the involvement of A2ARs in white matter pathology. However, all CNS immune cell types express A2ARs, limiting the specificity of information obtained from studies using A2A ligands, similarly to other ligands described here. Future post-mortem studies of A2AR expression with cell-specific markers, or multimodal studies as mentioned above, will aid in identifying the precise location and function of A2ARs in neuroinflammation.
α4β2 Nicotinic Acetylcholine Receptors
The nicotinic acetylcholine (NACh) system regulates many important physiological functions, including stress, arousal, cognition, and pain77, but has recently been also associated with neuroinflammation. In a multitracer animal study of ischemia, the authors demonstrated that the 2[18F]-fluoro-A85380 ligand for α4β2 NAChR (2-FA) has very similar patterns of uptake to the TSPO ligand [11C]PK1119578. Histological evidence showed concomitant upregulation of both TSPO and the α4β2 NACh receptor in activated microglia and astrocytes, suggesting that α4β2 may be a glial marker with a profile of cell-type expression similar to that of TSPO. While the 2-FA tracer has an unfavorably slow kinetic profile, requiring very long image acquisitions, kinetically favorable tracers are being developed, such as [18F]flubatine79. Thus, the study of α4β2 receptors as imaging markers of inflammation may become more widespread in the future. However, it should be noted that previous NAChR studies in neurological disorders generally reported decreased receptor binding77. This indicates drastic model-dependent differences in receptor expression, and further investigations are needed to understand how α4β2 receptors contribute to neuroinflammation.
MR-based methods
Magnetic resonance spectroscopy (MRS)
MRS uses MRI technology to extract quantitative information about brain metabolites. Several MRS measures have been used as putative markers of resident immunocompetent cells. Myo-inositol (mI) is an osmolyte, or volume-regulating molecule, proposed to be a marker of astrocytes, which become hypertrophic during an inflammatory response. In fact, mI is elevated in a wide array of pathologies thought to involve neuroinflammation (for a review, see 80). However, few studies have histologically validated the initial evidence for increased mI in reactive astrocytes81, and there is evidence of pathological mI changes in disorders without a known astrocytic component, such as Down’s syndrome82. These combined results indicate mI is a poor standalone marker for reactive astrocytosis, and neuroinflammation in general. A complementary neuroinflammatory marker should be added to MRS in order to better frame interpretations of mI in neuroinflammation. For example, a recent study in hepatitis C patients reported increases in both mI and TSPO binding in patients, strengthening evidence of neuroinflammation in this cohort83. However, the two measures were not correlated, suggesting that these target co-occurring, but distinct physiological processes.
Unlike many MRS metabolites, lactate is thought to be present only under pathological states, because of its use as an energy substrate under ischemic and hypoxic conditions. In line with this, increased lactate has been reported in acute ischemia and MS lesions84, 85. However, while average lactate concentration is relatively low in healthy brain tissue, it is a critical substrate in oxidative metabolism. Lactate concentrations increase during normal energy-consuming processes, like neural activity during visual stimulation, in quantities detectable by MRS86. Furthermore, its cell specificity is equivocal. Early research suggested a specificity for activated macrophages87, but other reports indicate that astrocytic glycolysis is the major brain source of lactate, arguing for astrocyte specificity88. Similar to mI, validation studies with animal models and immunohistochemistry are needed to better understand how lactate is involved in neuroinflammation. In addition, elevated choline has been detected in inflammatory and gliotic processes89. The choline resonance arises from several soluble components of brain myelin and fluid-cell membranes including glycerophosphocholine (GPC), phosphocholine (PCho), and choline (Cho). Elevated Cho levels have been interpreted to reflect glial activation, energy failure, macrophage infiltration, and demyelination, showing that, like mI and lactate, Cho is a nonspecific neuroinflammatory marker90.
MRS techniques are not without limitations. MRS measures of creatine are often used as an internal reference, because creatine is assumed to be relatively stable across brain parenchyma91. However, creatine levels may be altered in neuroinflammatory disorders80, suggesting that only absolute measurements should be used when comparing pathological populations. Methods used for absolute quantification include: a) phantom replacement techniques92 b) using the unsuppressed water signal as a reference93, c) the use of an external reference94 and d) reciprocity95. The spatial resolution of MRS is also much lower than structural MRI scans, making metabolites in small regions difficult to assess. Additionally, large MRS voxels contain a mixture of different tissue types, such that intracellular cannot be separated from extracellular compartments. Despite these limitations, MRS has provided and continues to provide important insights into neurochemical alterations observed during inflammation. The development of advanced MRS methods capable of increasing the number of metabolites detected (e.g., 2D-COSY)96, or fast 3D spiral encoding of spatial information97 will increase the applicability of MRS to the study of neuroinflammatory and other conditions.
Blood-brain barrier Imaging
The blood-brain barrier (BBB) is a crucial barrier that limits exposure of sensitive brain tissue to outside factors. Depending on the nature of the stimulus, BBB permeability may also be increased during a neuroinflammatory response. Astrocytes play an important role in maintaining BBB integrity, and their activation during neuroinflammation may regulate, in part, permeability of the BBB98. In the healthy CNS, the BBB limits brain entry of peripheral cells and potentially harmful molecules. However, if the CNS insult is severe enough, peripheral cells such as lymphocytes and monocytes may be recruited across the compromised BBB to help remove the debris and repair damaged tissue. There are several different strategies available to image BBB function.
Nuclear imaging methods
MRI is the predominantly used methodology for BBB imaging; however, nuclear imaging is better equipped to image efflux proteins, such as P-glycoprotein (P-gp). These proteins regulate brain molecular concentrations by actively pumping them from the brain across the BBB. Importantly, P-gp activity is known to be altered by inflammatory conditions99, which has indications for CNS delivery of therapeutic drugs. The PET tracer [11C]verapamil has been used to investigate P-gp function in healthy humans100, as well as animal models of neuroinflammation101.
MRI-based methods
Contrast-enhanced MRI (CE-MRI), generally with a gadolinium (Gd) chelate, is the workhorse for clinical BBB imaging. The simplest CE-MRI-based assessment of BBB integrity involves bolus infusion of contrast agent (e.g. Gd-DPTA), typically in conjunction with a T1-weighted MR sequence. Because Gd compounds do not readily cross a healthy BBB, changes in MR signal post-infusion versus pre-infusion indicate that contrast agent has entered the CNS via some BBB disturbance. This type of assessment provides only a semi-quantitative measurement of BBB changes, but recently more advanced kinetic models have been described to isolate individual BBB components, such as the volume transfer constant Ktrans (which is weighted by both perfusion and blood flow; reviewed in 102). These kinetic methods require dynamic data acquisition and a prolonged infusion of contrast agent, and are thus referred to as dynamic CE-MRI (DCE-MRI). T1-weighted DCE-MRI is most commonly used to assess BBB permeability, while dynamic susceptibility-enhanced MRI (DSC-MRI) with T2 or T2*-weighted sequences and contrast agent is often used to detect CNS hyper- or hypoperfusion103. Alternatively to DSC-MRI, MRI with arterial spin labeling (ASL) can be used to estimate perfusion104. As a method to image perfusion, ASL has certain advantages over DSC-MRI, as it does not require contrast agent, which causes allergic reactions in a small percentage of people and is somewhat invasive. Additionally, recent work improved BBB permeability estimates by combining Ktrans measured by DCE-MRI with ASL estimates of cerebral blood flow105.
One of the pathologies where BBB imaging is most prevalent is MS, and a great amount of information on the development and persistence of lesions has been obtained with these methods in vivo in MS patients11. While much of the literature describes imaging of MS white matter lesions with T1-weighted DCE imaging, more recently developed techniques (e.g. post-contrast T2- weighted Fluid-Attenuated Inversion Recovery [FLAIR]) enable imaging of BBB disruption in areas previously not studied with neuroimaging, such as the leptomeninges106 (Figure 3). While several studies suggest that these techniques are sensitive to BBB compromise, it should be noted that they do not provide causal information, as different mechanisms of BBB compromise may lead to similar outcomes as detected by MRI.
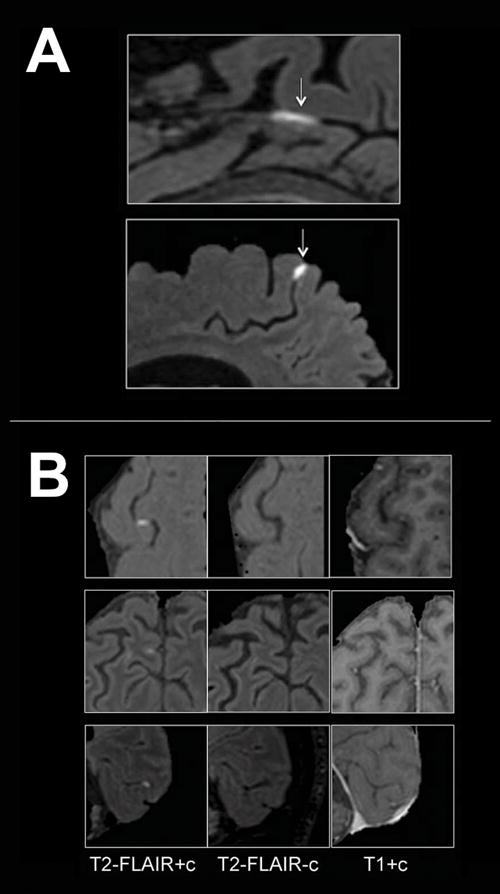
Examples of BBB imaging with contrast in MS patients. (A) Examples of leptomeningeal contrast enhancement in two representative relapsing-remitting MS cases. Foci of high signal (arrows) on 3T post-contrast T2-FLAIR images indicate leptomeningeal enhancement. Extracerebral tissues have been masked for clarity. (B) Signal intensity on different MRI sequences. Three foci of leptomeningeal enhancement are visible on post-contrast T2-FLAIR scans (left column), but not on the corresponding pre-contrast T2-FLAIR (middle column). In the right column, post-contrast T1-weighted images show minimal abnormal signal that would not routinely be classified as enhancement. T2-FLAIR = T2-weighted, fluid-attenuated inversion recovery. Reproduced from Absinta et al., “Gadolinium-based MRI characterization of leptomeningeal inflammation in multiple sclerosis”, Neurology vol. 85 no. 1, 18–28106 with permission from Wolters Kluwer Health.
Imaging infiltration of immune cells
During many neuroinflammatory events (e.g. during BBB disruption) circulating immune cells are recruited from the periphery and traffic to the CNS. Similar to the other neuroinflammatory players covered here, the contribution of peripheral immune cells to a neuroinflammatory response varies largely depending on the nature of the inciting event. Generally, the more severe the event is, the greater the likelihood of peripheral cell infiltration. For example, leukocyte and macrophage migration to CNS tissue is a hallmark of both MS and cerebral ischemia, and plays a large part in pathological progression107. However, recent evidence suggests that monocyte trafficking to the brain may also play an important role in the development of disorders not characterized by the presence of lesions, such as chronic stress108. Several neuroimaging techniques are suitable for the study of immune cell trafficking and infiltration in human neuroinflammation.
Nuclear imaging methods
For many years, human leukocyte trafficking was imaged by isolating leukocytes from blood samples, incubating with radiotracer (e.g. 111 In, 99mTc, 18F-FDG) and re-injecting the subject with autologous cells before PET or SPECT imaging (reviewed in 15). These techniques have largely fallen out of use in clinical studies, due to several disadvantages, including: a) cell preparation is time and labor intensive; b) labeled cells are also prone to loss of label in vivo via apoptosis or proliferation, as well as through non-physiological mechanisms (e.g. passive diffusion); c) if the BBB is compromised, activity leaked from labeled cells may contribute to non-specific brain signal; d) discrimination of intraparechymal from intravascular cellular location is difficult to achieve109; e) processing required to isolate and label individual cell types may be complex110, or alter cell tracking, (e.g non-metabolically active cells must be treated to increase 18F-FDG uptake)111. For all these reasons, the use of nuclear imaging to study immune cell tracking in neuroinflammation has been so far limited.
MR-based methods
MRI of human immune cell trafficking can be accomplished via cell labeling with superparamagnetic particles of iron oxide (SPIO). Typically, SPIOs are administered as a bolus to the bloodstream, where circulating monocytes internalize the particles, due to their high phagocytic activity. The iron oxide core shortens T1 and T2/T2* relaxation times, leading to hyperintense T1-weighted signal, and hypointense T2/T2*-weighted signal. SPIOs are classified by iron core diameter, ranging from 10–50 nm (ultra-small SPIO; USPIO) to 50–100 nm (SPIO) and up to >1 μm (micrometer-sized particles of iron oxide; MPIOs)112. USPIOs are the particles most commonly used for human imaging of monocyte/macrophage tracking, and have been used successfully in a number of studies of human disorders characterized by neuroinflammation, including MS and stroke (reviewed in 113). Though monocyte tracking yields important pathological information, combination with additional imaging modalities can uncover results that neither in isolation can. For example, a recent multimodal study employed longitudinal [11C]PK11195 PET and USPIO MR scanning in an animal stroke model114. The authors found both overlapping and separate stroke-induced changes in PK and USPIO signal. This observation suggests that the brain infiltration of peripheral macrophages and the activation of resident immunocompetent cells (e.g. microglia, astrocytes) in the neuroinflammatory response is partially dissociable, and further showed differences in temporal upregulation of both types of molecules.
MR studies with SPIOs have great potential for imaging human neuroinflammation, but they are also limited. In vitro labeling of autologous cells (e.g., monocytes) in humans is theoretically possible, but has not been reported. One of the potential pitfalls with in vitro labeling is that achieving enough labeling to allow for good signal detection may be challenging within the limits of a safe and tolerable dose. In vivo labeling likely results in both a smaller proportion of cells labeled and a higher concentration of cell-free iron oxide label than in vitro labeling, increasing false negative and false positive rates. Similar to radioligand labeling, in vivo-labeled cells can lose their label, resulting in signal inaccuracies as cell-free SPIOs enter the brain, particularly in disorders with increased BBB permeability. However, using MRI with both Gd and USPIO, it was shown that regions with BBB disruption were completely distinct from those showing USPIO uptake in stroke patients, suggesting that USPIOs may not cross the compromised BBB115. Finally, many SPIO-MRI studies use only qualitative or semi-quantitative signal differences as an outcome measure. A radiolabeled USPIO has been developed that would allow for absolute quantitation, but only preclinical applications have been used thus far116.
Imaging pathological consequences of neuroinflammation
Although distinct and downstream from the neuroinflammatory players discussed above, the resulting products of neuroinflammation are also important targets to investigate neuroinflammation. Like the events that cause them, the consequences of neuroinflammation are varied, and dependent on the nature of the neuroinflammatory event. Commonly studied pathological outcomes are edema, demyelination, cellular and axonal damage, and several different imaging techniques have been developed for this purpose. This type of research has important implications for diagnosis of acute insult and characterization of therapies targeting neuroinflammation.
Nuclear imaging methods
Several radiotracers have been developed for the purpose of myelin imaging, but currently only 11C-labeled Pittsburgh Compound B (PIB) has been used in human subjects15. Originally developed for β-amyloid imaging, studies have shown that [11C]PIB is able to assess demyelination117. In vitro and ex vivo experiments, however, have questioned the mechanism of PIB-white matter binding, as it seems nonsaturable and nonspecific118, 119. A recent study found strong correlations between [11C]PIB binding and expression of myelin-associated mRNA from the Allen Human Brain Atlas, suggesting that [11C]PIB does specifically bind with myelin-associated proteins120.
A great deal of interest has also gone into radiotracers for imaging cell death. The PET/SPECT ligands [11C]flumazenil (FMZ) and [123I]iomazenil, which target GABA-A receptors, have shown utility for imaging neuronal integrity in several disorders, including stroke and AD121, 122. While regional decreases in GABA-A binding seem to colocalize with regional neuronal death in human post-mortem data, some animal experiments reported poor correlation of FMZ signal with histological evidence of neuronal death123, 124. The dissociation between FMZ and neuronal death in these studies could suggest that nuclear imaging estimates of GABA-A receptor density are more specific for subtle processes, such as dendritic loss, which has been shown to be a consequence of ischemia125.
Current nuclear imaging technologies may be imperfect for detecting frank neuron loss, but there are several tracers specific for apoptotic cells (reviewed in 126). The majority of these ligands target the apoptotic marker phosphatidylserine (PS) and molecules associated with it, such as Annexin V. SPECT imaging with 99mTc-conjugated Annexin V has been used to study apoptosis for many years15, though the relatively poor resolution of SPECT incentivized PET tracer developments efforts. [18F]-labeled Annexin V synthesis has been described, but only in preclinical models126. Annexin V imaging is also limited because PS appears on necrotic as well as apoptotic cells, but several recent alternatives exist. 18F-ML-10 is a derivative of the Aposense family of apoptotic markers127. 18F-ICMT-11 and 18F-CP-18 are both specific to caspase-3, a crucial enzyme for initiation of apoptosis128, 129. Each of these tracers has received safety validation in healthy controls, but none have yet been used to study neuroinflammatory disorders.
MR-based methods
Various MR sequences can be used to study the consequences of neuroinflammation. Though many MR-methods are limited by a lack of specificity compared to nuclear methods, advances in sequence development and data analysis continue to improve, and combination with additional measures of inflammation strengthens results from individual techniques.
Voxel-based morphometry (VBM) and cortical thickness, measured with structural T1 MRI, are two of the most commonly used MR techniques to assess CNS pathology in experimental studies. The structural metrics obtained with these techniques are not direct measures of cell number or density and are likely to reflect a combination of many cellular processes130. Nonetheless, these methods are sensitive to anatomical changes that can occur in the context of pathology such as atrophy8, 130, 131, that have been linked to inflammation132. For instance, a recent multimodal study in AD demonstrated a negative association between gray matter volume and [11C]PBR28-measured TSPO levels133, thus supporting a relationship between gray matter loss and neuroinflammation. While most of the MRI structural studies focus on gray and white matter, a recent investigation used VBM to demonstrate enlarged choroid plexus volume in complex regional pain syndrome (CRPS)134. As the choroid plexus sits at an important entry point for circulating immune cells into the CNS, these results suggest yet one more link between neuroinflammation and chronic pain44, and further highlight the flexibility of MR-based methods.
N-acetyl aspartate (NAA) is a MRS-measured metabolite thought to be a marker of neuronal integrity. Therefore, studies often interpret reduced NAA (or NAA normalized by creatine levels, NAA/Cr) as evidence for neuronal loss80. Reductions in NAA signal have been reported for a wide range of neuroinflammatory disorders, but there is evidence that NAA levels can return to baseline after a period of recovery135. Therefore, NAA is likely not a specific marker of neuronal death, and some evidence suggests that NAA levels reflect more closely the functional capacity of neuronal mitochondria135.
Pathological products of neuroinflammation such as edema, demyelination, tissue loss, and iron accumulation lead to variable changes in quantitative measures of proton relaxation times (T1, T2, and T2*) as well as in semiquantitative parameters such as magnetization transfer ratio (MTR)136.
As explained in detail in 136–138, T1 relaxation times (rt) in the CNS are essentially influenced by free water protons and by the relative amount of CNS tissue components (e.g. cells, myelin, lipids, proteins). In this context, increased T1-rt indicates increased water content (i.e. due to inflammatory edema) or decreased CNS tissue “structure” (i.e. loss of cells, axons, myelin, etc.) Similarly, greater density of CNS “structure” and reduced water content as well as iron accumulation tend to reduce T1-rt.
T2 relaxometry measures the loss of spin coherence of excited water molecules, therefore reflecting the dynamic state of water protons and of their interaction with macromolecules in a tissue. An increase in T2-rt in the CNS may be due to a loss of macromolecules (e.g. myelin in white matter) and/or increased water content, which may be intracellular (e.g. neuroinflammatory gliosis) or extracellular (e.g. neuroinflammatory edema).
Iron accumulation is also considered to be a consequence of mitochondrial dysfunction, which may be provoked by CNS inflammation. Among existing relaxometry techniques, T2* relaxometry best reflects iron accumulation. The T2*-rt is a measure of the loss of transverse magnetization due to T2 relaxation and magnetic field inhomogeneities, which depend on the presence of paramagnetic or ferromagnetic ions like iron. Because of this, an increase in T2* most often indicates a loss of macromolecules or an increase in water (T2 component), while a decrease suggests an increase of macromolecular compounds (T2 component) or iron (susceptibility).
MT images are based on the interaction between macromolecule-bound immobilized protons and free water protons. A lower MTR therefore indicates a reduced spin exchange between free and bound protons, suggesting neuroaxonal damage or myelin breakdown and/or water increase. Another commonly used MR technique to assess neuroinflammation is diffusion-weighted imaging (DWI)139.
DWI measures the diffusion of water molecules, and has been widely used to study white matter changes in demyelinating and hypomyelinating disorders. Myelin-sheathed axons restrict the free diffusion of water molecules (anisotropy), and thus a reduction in directional diffusion is often assumed to indicate white matter disruption. DWI, like the methods described above, suffers from nonspecificity, and can be influenced by axonal pathology as well as changes in myelin content140. However, advanced DWI models appear to improve correlations with histology141.
The combination of multiple MR contrasts (mcMRI) provides high specificity and sensitivity to detect CNS inflammatory processes. Thanks to recent hardware and software development, it is nowadays feasible to acquire multiple quantitative and semi-quantitative MRI techniques in a clinically compatible protocol137, 138. Combining T1, T2 and T2* relaxometry with MTI, Bonnier et al.137 showed subtle increases in T2- and T2*-rt in NAWM of early stage and minimally impaired MS patients. T2/T2* increases, in the absence of significant changes in T1-rt, suggested extracellular water accumulation in patients suffering from chronic autoimmune neuroinflammation. In addition, by combining the four contrasts above, the authors classified MS lesion pathology and identified groups of lesions with predominant inflammatory or degenerative components138.
The multimodal approach above shows once again that results from somewhat nonspecific MR methods may also be strengthened with a multimodal approach. Similarly, a recent TSPO PET imaging study in MS also acquired MTR, and found differential glial activation in nonlesional regions dependent on high or low MTR, which was assumed to be myelin-related in the context of the study142.
In sum, MRI metrics are important for characterization of neuroinflammatory disorders, and some have already proven their usefulness for diagnosis, and follow-up of patients affected by neuroinflammation. Future efforts should focus on exploring the clinical feasibility of combined mcMRI and DWI acquisitions with techniques like MRI fingerprinting143 and on the reproducibility and standardization of MRI measurements of neuroinflammation.
Conclusions
As new findings increasingly suggest the involvement in neuroinflammation in a host of different human disorders, sensitive and specific in vivo imaging methods will continue to be important for accurate depiction of complex neuroinflammatory processes. Advances in our understanding of neuroinflammation occurring independently of neuroimaging are crucial for this field of research to mature. Ideally, translational collaborations between basic and clinical scientists will lead to more specific and relevant neuroimaging targets to study neuroinflammation. In addition to development of novel techniques, neuroimaging researchers need to design studies using existing methods in innovative ways to overcome the inherent limitations described here. This could encompass refining existing data analysis techniques, or the clever use of multimodal studies, where results from one imaging technique can inform results obtained with the other. Several such studies have been highlighted in this review, showing that relatively unspecific methods often can yield more specific information about pathology when paired with a complementary technique. We expect that neuroimaging, complemented by basic science discoveries, will make fundamental contributions to research and clinical investigations of neuroinflammation in CNS pathology.
Table 1
Summary of neuroimaging agents targeting neuroinflammatory processes
Neuroinflammatory Target | Modality | Imaging Agent/Technique |
---|---|---|
CNS Immunocompetent cells | ||
TSPO | PET | [11C]PK11195, [11C]Ro5-4864, [11C]vinpocetine |
[11C]PBR28, [11C]DAA1106, [11C]DPA-713 | ||
[18F]DPA-714, [18F]FEDAA1106, [18F]PBR111 | ||
[18F]PBR06, [18F]FEPPAA | ||
SPECT | [123I]PK11195, [123I]CLINDE | |
MAO-B | PET | [11C]-D-deprenyl, [11C]-deprenyl-D2 |
COX-1 | PET | [11C]ketoprofen, [11C]KTP-Me |
Arachidonic Acid | PET | [11C]AA |
Adenosine A2A | PET | [11C]TMSX, [11C]SCH442416 |
α4β2 NAChR | PET | [18F]2-FA, [18F]flubatine |
Myo-inositol | MRI | MR Spectroscopy |
Lactate | MRI | MR Spectroscopy |
Choline | MRI | MR Spectroscopy |
Blood-brain barrier | ||
P-glycoprotein | PET | [11C]verapamil, [11C]loperamide |
[11C]desmethyl-loperamide | ||
BBB permeability | MRI | DCE-MRI with gadolinium chelates |
BBB perfusion | MRI | DSC-MRI with gadolinium chelates, ASL |
Infiltration of immune cells | ||
Leukoctyes | PET | [18F]FDG |
SPECT | 111 In, 99mTc | |
Monocytes | MRI | Superparamagnetic iron oxide particles |
(SPIO, USPIO, MPIO) | ||
Pathological consequences of neuroinflammation | ||
Demyelination | PET | [11C]PIB |
MRI | mcMRI, DWI, MTR | |
Apoptosis | ||
![]() | SPECT | [99m]Tc-Annexin V |
![]() | PET | [11C]CP-18, [18F]ICMT-11 |
![]() | [18F]FM-10 | |
Neuronal loss | ||
![]() | PET | [11C]flumazenil |
![]() | MRI | MR Spectroscopy |
![]() | MRI | mcMRI, VBM, cortical thickness |
Iron Accumulation | MRI | T2* |
Edema | MRI | mcMRI, MTR |
Acknowledgments
We would like to acknowledge the following funding mechanisms for support of this project: 5T32EB13180 (T32 supporting Dr. Albrecht), 1R21NS087472-01A1 (MLL), and DoD W81XWH-14-1-0543 (MLL). We would also like to thank Dr. Eva Ratai for helpful feedback on the manuscript.
References
Full text links
Read article at publisher's site: https://doi.org/10.1021/acschemneuro.6b00056
Read article for free, from open access legal sources, via Unpaywall:
https://europepmc.org/articles/pmc5433433?pdf=render
Citations & impact
Impact metrics
Citations of article over time
Alternative metrics
Smart citations by scite.ai
Explore citation contexts and check if this article has been
supported or disputed.
https://scite.ai/reports/10.1021/acschemneuro.6b00056
Article citations
Evolving Radiological Approaches in the Diagnosis and Monitoring of Arachnoiditis Ossificans.
Cureus, 16(9):e68399, 01 Sep 2024
Cited by: 0 articles | PMID: 39355477 | PMCID: PMC11444744
Review Free full text in Europe PMC
A blood-free modeling approach for the quantification of the blood-to-brain tracer exchange in TSPO PET imaging.
Front Neurosci, 18:1395769, 22 Jul 2024
Cited by: 0 articles | PMID: 39104610 | PMCID: PMC11299498
Exploring Symptom Overlaps: Post-COVID-19 Neurological Syndrome and Post-Concussion Syndrome in Athletes.
Biomedicines, 12(7):1587, 17 Jul 2024
Cited by: 0 articles | PMID: 39062160 | PMCID: PMC11274969
Review Free full text in Europe PMC
Characterization of cortico-meningeal translocator protein expression in multiple sclerosis.
Brain, 147(7):2566-2578, 01 Jul 2024
Cited by: 1 article | PMID: 38289855
Evaluation of microglia activation related markers following a clinical course of TBS: A non-human primate study.
PLoS One, 19(5):e0301118, 16 May 2024
Cited by: 0 articles | PMID: 38753646 | PMCID: PMC11098425
Go to all (108) article citations
Similar Articles
To arrive at the top five similar articles we use a word-weighted algorithm to compare words from the Title and Abstract of each citation.
Recent developments on PET radiotracers for TSPO and their applications in neuroimaging.
Acta Pharm Sin B, 11(2):373-393, 25 Aug 2020
Cited by: 55 articles | PMID: 33643818 | PMCID: PMC7893127
Review Free full text in Europe PMC
Evidence of diffuse cerebellar neuroinflammation in multiple sclerosis by 11C-PBR28 MR-PET.
Mult Scler, 26(6):668-678, 11 Apr 2019
Cited by: 10 articles | PMID: 30973800 | PMCID: PMC6788961
In vivo quantitative imaging of hippocampal inflammation in autoimmune neuroinflammatory conditions: a systematic review.
Clin Exp Immunol, 210(1):24-38, 01 Oct 2022
Cited by: 4 articles | PMID: 35802780
Review
In vivo molecular imaging of neuroinflammation in Alzheimer's disease.
J Neurochem, 149(4):438-451, 26 Nov 2018
Cited by: 49 articles | PMID: 30339715 | PMCID: PMC6563454
Review Free full text in Europe PMC
Funding
Funders who supported this work.
NIBIB NIH HHS (2)
Grant ID: 5T32EB13180
Grant ID: T32 EB013180
NINDS NIH HHS (2)
Grant ID: R21 NS087472
Grant ID: 1R21NS087472-01A1
Swiss National Science Foundation (1)
Advanced techniques to study the physiopathology of multiple cclerosis (MS) using high and ultra-high field MRI
Cristina Granziera, University of Lausanne
Grant ID: 154508
U.S. Department of Defense (1)
Grant ID: W81XWH-14-1-0543
U.S. Department of Health and Human Services (2)
Grant ID: 1R21NS087472-01A1
Grant ID: T32 5T32EB13180