Abstract
Free full text

Modeling ALS using motor neurons derived from human induced pluripotent stem cells
Abstract
Directing the differentiation of induced pluripotent stem cells into motor neurons has allowed investigators to develop novel models of ALS. However, techniques vary between laboratories and the cells do not appear to mature into fully functional adult motor neurons. Here we discuss common developmental principles of both lower and upper motor neuron development that have led to specific derivation techniques. We then suggest how these motor neurons may be matured further either through direct expression or administration of specific factors or co-culture approaches with other tissues. Ultimately, through a greater understanding of motor neuron biology, it will be possible to establish more reliable models of ALS. These in turn will have a greater chance of validating new drugs that may be effective for the disease.
Introduction
Human induced pluripotent stem cells (iPSCs) are derived by expressing pluripotency genes in differentiated adult somatic cells. This reprograms the cells back in time to an embryonic-like pluripotent state1–3. These pluripotent cells can then be differentiated into most any cell type of the human body. Furthermore, when taken from patients with specific neurological disorders, the cells can be used to create powerful “disease in the dish” models that recapitulate certain patient disease phenotypes. Corticospinal “upper” motor neurons (UMNs) and spinal cord “lower” motor neurons (LMNs) specifically degenerate in motor neuron diseases such as amyotrophic lateral sclerosis (ALS). This unexplained MN wasting results in paralysis and death normally within 4 years of disease onset. Apart from one drug Riluzole that extends lifespan by approximately 3 months, there are no current treatments for this disease.
Recent studies generating MNs from ALS patient’s iPSCs have revealed specific disease-relevant phenotypes, thereby validating the use of this system to explore the molecular underpinnings of ALS and to develop new screening platforms for novel drug development4. However, certain key challenges still remain, namely: which criteria to use to identify MNs at different stages in development; how to compare the many existing protocols for LMN differentiation; how to establish directed UMN differentiation strategies; and how to properly mature MNs in vitro. This review summarizes the current state for iPSC generation into MNs for investigators interested in the basic biology and ultimate treatment of MN-based diseases.
Generating Lower Motor Neurons from Pluripotent Stem Cells
LMNs are a highly studied neuronal subtype due to their critical role in activating skeletal muscle. Current protocols for directing pluripotent stem cells (PSCs) toward LMNs are built upon extensive neural specification studies conducted in amphibian, chick and mouse embryos5–7; (reviewed by Jessell8, Kanning9 and Stifani10). During gastrulation (Figure 1, i), ectodermal cells are initially fated along the anterior-posterior axis by activation of fibroblast growth factors (FGFs) and Wingless-type MMTV integration site family members (Wnts) (Figure 1, ii). The neuroectoderm is specified (Figure 1, iii) by inhibition of mesoderm and endoderm differentiation factors bone morphogenic proteins (BMPs) and transforming growth factor beta (TGFbeta). Because the mere inhibition of alternate signaling pathways (e.g., BMP; activin/TGFbeta) is sufficient for neural induction, neural fate is seemingly the “default” path of embryonic differentiation11, 12. As the neuroectoderm is specified, signaling gradients act as positional cues to establish rostrocaudal and dorsoventral neural axes13. Presomitic mesoderm cells surrounding the spinal cord express Aldehyde Dehydrogenase 1 Family, Member A2 (Aldh1a2), an enzyme that synthesizes retinoic acid (RA) (Figure 1, iv). RA induces caudal neuronal subtypes of the hindbrain and rostral spinal cord and further directs neurogenesis. RA signaling decreases caudally down the spinal cord, as indicated by both decreased expression of Aldh1a2 and increased inhibitory activity of FGFs that are highly expressed in the lumbar spinal cord and tail bud14. In addition to RA and FGFs, Wnts are also required for the induction of caudal hindbrain and spinal cord identities15. Another key molecule is the signaling protein Sonic Hedgehog (SHH) that determines dorsoventral spinal identity, and is secreted from the notochord in a ventral gradient along the ventrodorsal axis (Figure 1, v)16. High SHH concentrations have been shown to promote ventral spinal subtypes and are critical for MN induction17.
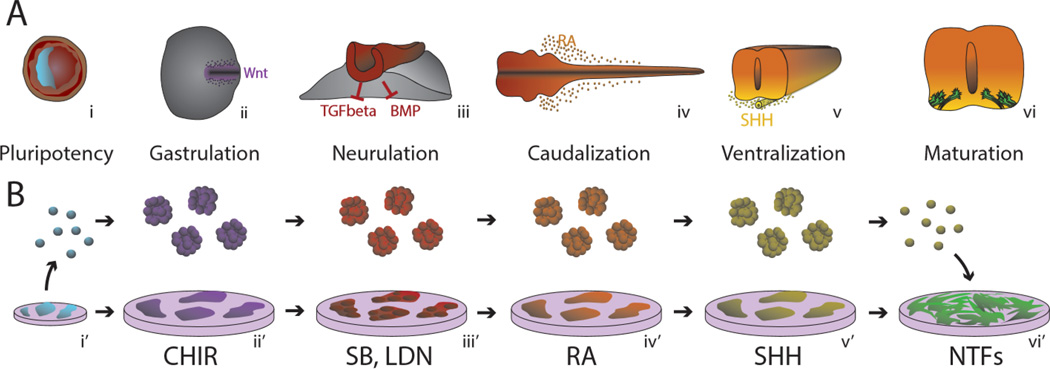
Emulating MN Developmental Signaling In Vitro. Developmental stages of human MNs (a) are reproduced in vitro (b) through the use of small molecule and recombinant signaling molecules. (i) Blastocyst containing pluripotent stem cells derived from the inner cell mass (blue) is generated in vitro from adult somatic tissue through reprograming into iPSC cultures. During gastrulation, Wnt-dependent primitive streak formation (ii) is simulated using a GSK3 inhibitor (CHIR99021). Neural ectoderm that emerges during neurulaiton (iii) is directed through the use of dual-SMAD inhibitors SB431542 and LDN193189 (SB, LDN). Retinoic Acid (RA) (iv) is produced by neighboring somites (not shown) that act as caudalizing molecules that specify a hindbrain and anterior spinal cord fate (iv’). (v) Sonic Hedgehog (SHH) is released from the ventral notochord, causing a gradient that induces MN fate in the ventral portion of the spinal cord. (v’) This is reproduced in vitro with small molecule (smSHH) or recombinant SHH signaling agonists. (vi) MN progenitors depend on trophic support to connect axon projections to target muscles and develop into functioning LMNs. (vi’) Neurotrophic factors (NTFs) such as GDNF, BDNF, CNTF and others are used in vitro to provide maturation and survival signaling.
In seminal studies, these developmental signaling molecules were used to guide mouse embryonic stem (ES) cells into MNs in vitro18. Mouse ES cells were induced to differentiate by withdrawing the mitogen Leukemia Inhibitory Factor, and cultured as self-organizing suspended cellular aggregates referred to as embryoid bodies (EBs). By exposing mouse ES cells to RA and SHH in the presence of knockout serum, EBs were directed to a LMN fate. In human ES (hES) cells, the same developmental principle was followed with the consideration that, even in vitro, human cells inherently follow a much longer rate of development. hES cells were first differentiated to EBs and then into primitive neural stem cells in the absence of morphogens19, 20. This was followed by treatment with RA and SHH to induce ventral spinal progenitors before they became postmitotic MNs21. While these protocols showed promise and have been widely used, they were long, expensive and inefficient.
Enhanced LMN Generation in vitro
A key breakthrough came with the discovery by Chambers et al. (2009)22 that inhibition of BMP and TGFbeta signaling by the small molecules SB431542 (SB) and LDN193189 (LDN) early during PSC differentiation selectively blocks endoderm and mesodermal cell fates. This induced “default” neural specification, termed dual-SMAD inhibition, dramatically enriches neural ectoderm directly from pluripotent cells as indicated by a high percentage of paired box protein 6 (Pax6) and sex determining region Y-box 2 (Sox2) expressing neural progenitors in ES cell and iPSC cultures (Figure 1 iii’). Early Pax6/Sox2 enrichment in turn intrinsically represses alternate mesoderm and endoderm fates thereby substantially reducing the length of differentiation protocols and, critically, the appearance of non-neuronal cell types.
Drawing from these discoveries, most human embryonic and iPSC-derived LMN (referred to hereafter as hPSC-LMN) differentiation protocols share three fundamental steps in induction: neuralization through dual-SMAD inhibition, caudalization through RA exposure and ventralization through SHH activation by recombinant SHH protein or small molecule SHH activators (smSHH). Of note, smSHHs potentially bypass feedback mechanisms related to SHH itself including observed upregulation of Patched and Shh binding protein Hhip23. The downstream consequences in neuronal differentiation between smSHH and recombinant SHH have yet to be compared in vitro. Beyond these common patterning principles, the recent protocols for MN induction from PSCs are described below (Figure 2)24–28 and show that even subtle in vitro differences in timing, plating and media composition can strongly influence MN yield, purity and phenotype.
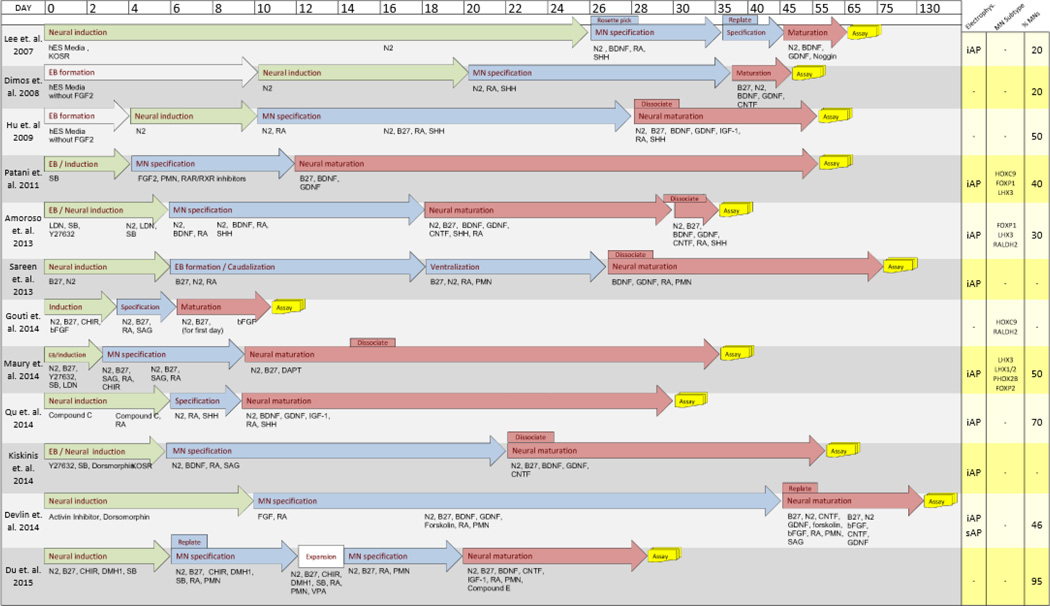
Comparison of Published LMN Differentiation Protocols. 12 iPSC to LMN protocols compared with respect to time (days in vitro). End of experiment (Assay) based on last data presented. Fibroblast growth factor 2 (FGF2), brain-derived neurotropic factor (BDNF), glial cell line-derived neurotropic factor (GDNF), cilliary neurotropic factor (CNTF), retinoic acid (RA), retinoic acid receptor (RAR), sonic hedgehog (SHH), purmorphamine (PMN). Yellow column lists summary of results, unique MN subtype markers observed, and approximate percent yield of MNs based on reported percent cells expressing HB9 or Islet-1. Induced action potentials (iAP), spontaneous action potentials (sAP), Caudal spinal cord associated Hox family member C (HoxC9), Forkhead Box P1, P2 (FoxP1), (FoxP2) LIM Homeobox 3 (LHX3), LIM Homeobox 1/2 (LHX1/2), Paired Mesoderm Homeobox 2B (PHOX2B).
Until recently, the caudalization and ventralization phases of human MN differentiation had not been systematically improved over general RA and SHH administration. This resulted in inefficient yields of human MNs compared to mouse ES cell protocols. By altering the concentration, order and timing of the RA, Wnt3a, FGF2 and SHH signals during differentiation in vitro, Maury et al. (2014)25 reveal a previously under-appreciated role of graded Wnt signaling in MN development. Wnt activation with the glycogen synthase kinase 3 (GSK3) inhibitor CHIR-99021 (CHIR) resulted in up to 80% of cells expressing the oligodendrocyte transcription factor (Olig2), a marker for MN progenitor cells (Figure 1, ii’). Remarkably, this optimization of caudalization also results in the generation of MNs from pluripotent cells in as little as 14 days25. Similarly, by altering ventralizing signals, Du et al. (2015) indicate that while high concentrations of smSHH can efficiently induce Olig2-expressing ventral progenitors, perhaps by bypassing endogenous feedback mechanisms, they also promote the specification of super-ventral progenitors that co-express NK2 homeobox 2 (NKX2.2). These co-expressing progenitors produce interneurons instead of MNs during the neurogenic phase. To limit the specification of NKX2.2-expressing progenitors and still promote the generation of Olig2-expressing progenitors, a cocktail of CHIR and SB can be applied to dampen the effect of smSHH. This thereby reduces the most ventral NKX2.2-expressing progenitors induced by the highest concentration of smSHH and enriches the Olig2+/Nkx2.2− MN progenitors29.
While a protocol to generate a pure population of MNs in the shortest amount of time may benefit manufacturing and screening, it remains unclear whether “fast-tracked” hPSC-LMN maturation paradigms accurately reflect overall cell physiology and maturation.
Rostral-Caudal Patterning of LMNs
It is clear that current MN differentiation protocols to do not give rise to more caudal types of neurons associated with lower limb function in animals and humans. The sequential expression of Cdx and Hox family members during development, referred to as colinearity30, dictates rostro-caudal identity along the hindbrain and spinal cord30–32. Early Hox family members associated with hindbrain, cervical-spinal and brachial-spinal origin are predominantly expressed in hPSC-LMNs25, 33, 34. Further refinement of this rostral spinal cord phenotype indicates an enrichment of HoxC4, HoxA5, HoxC6 and HoxC833. HoxA2, HoxA4, HoxB4 and HoxA5, associated with hindbrain and rostral spinal cord identity, are highly dependent on RA signaling in vitro25, 27. hPSC-LMN protocols developed thus far do not generate cells that significantly express HoxC9 through HoxC12, which are associated with thoracic and lumbar identities27, 33, 35. However, very low expression of HoxC9 and HoxC10 is observed when RA is omitted during differentiation36.
It may be possible that developmental FGF, TGFbeta and RA signaling pathways could lead to refined protocols that enrich for lumbar spinal identities. FGF8 and growth differentiation factor 11 (GDF11) are highly expressed at caudal levels of the developing spinal cord and tail bud. Treatment with a high concentration FGF8-soaked beads induces lumbar MN identity in chick embryonic neural explants, and the addition of GDF11 significantly increases the expression of HoxC9 and HoxC10 family members37. The repression of RA receptor gamma (RARγ) signaling, both by small molecule inverse agonists and overexpression of dominant negative RARγ, increases the expression of posterior Hox genes in Xenopus embryos38. The specific activities of these lumbosacral morphogens are currently untested in hPSC-LMNs, and additional or distinct signaling molecules may be required to modify Hox gene profiles.
In contrast to the protocols described above, the description of a common axial neuromesodermal progenitor (NMp) cell population in the caudal epiblast and tail bud of chicken6, mouse39–41 and human42 embryos has led investigators to develop protocols with distinct caudal LMN signatures. Following developmental evidence that neuroectodermal patterning specifies anterior neural fates43, bipotent NMps are unique in expressing both primordial neural and mesodermal signatures44, 45. Following FGF signaling, neuromesodermal induction in human ES cells is achieved through Wnt activation by recombinant Wnt3a or CHIR44, yielding up to 80% of cells expressing both the neural marker Sox2 and the mesodermal marker, Brachury. NMps can subsequently elicit either spinal neural tissue or paraxial mesoderm fates, which constitute the spinal cord or adjacent somites, respectively. In contrast to the observations of Patani et al. (2001) described above, posterior Hox genes HoxC9 and HoxD10 are observed at significantly higher levels by altering the timing of RA administration after activation of Wnt signaling by CHIR46.
Whether or not NMp differentiation schemes represent a distinct population of MN progenitor cells from that of hPSC-LMNs remains to be seen. Wnt-induced activation of Cdx and Hox genes by CHIR is an important similarity between hPSC-LMN and NMp protocols. The down-stream effects of blocking neuromesodermal identity by early dual-SMAD inhibition, however, have not been adequately compared. Clearly, more work is necessary to compare these methods with respect to one another (Figure 2), and the manipulation of lumbosacral morphogens described above may be similarly applicable in both differentiation strategies to achieve more caudal MN fates. To conclude, global anterioposterior axis representation in vitro will be needed in order to study hindlimb-specific onset commonly observed in mouse models of MN disease47 or lower limb onset in ALS.
General and Sub-type Specific Markers of hPSC-LMNs
Protocols used in ALS-disease modeling studies to date characterize LMNs by the expression of molecular markers. While no single marker is MN specific, the co-expression of two or three markers can provide stringent and reliable criteria for MN identification. Nascent LMNs are characterized by transient co-expression of LIM homeodomain (LIM-HD) transcription factors: insulin gene enhancer 1 (ISL1), LIM homeobox 3 (LHX3) and MN and pancreas homeobox 1 (MNX1, better known as HB9)48, 49. Of these, HB9 is the most specific and is most often used as the primary method for identification of stem cell-derived LMNs. Analysis relying on this marker alone, however, could result in over-estimation due to its expression in a subset of spinal interneuron subtypes shown in mouse50. Further, HB9 is rapidly down-regulated in a subset of brachial and lumbar limb innervating LMNs and in preganglionic thoracic LMNs51.. This in turn could paradoxically lead to an underestimation of LMNs in culture. Many studies therefore rely on Isl1 and Isl2 antigens or a combination of HB9 and ISL1/2 immunostaining (referred to as pan-MN staining24). Overall, LIM-HD proteins are eventually down-regulated during development, making characterization of more mature LMNs challenging. SMI32, an antibody that recognizes LMN-enriched Neurofilament Heavy Chain (NFH)52 facilitates morphological studies; however, NFH expression in other cell types complicates analysis of mixed populations. Choline acetyltransferase (ChAT) is expressed by all cholinergic neurons including LMNs, however expression is weak in young stem cell derived-LMNs and therefore it is not a reliable marker for young motor neurons, but is a good marker for mature ones. Another cholinergic marker that labels both MN cell bodies and their cholinergic presynaptic specializations is vesicular acetylcholine transporter (VAChT)53. However, similar to ChAT, its expression is detectable only in more mature MNs. Non-traditional molecular markers such as micro-RNAs (miRs) have promising utility for accurate MN identification in vitro, with miR-218 being recently identified to be the most MN-specific to date54.
Strategies to generate and quantify hPSC-LMN diversity (reviewed by Stifani10) beyond pan-LMN markers are necessary to assess vulnerabilities specific to LMN subtypes in in vitro models of ALS55. Investigators have begun to characterize hPSC-LMNs as limb-innervating lateral motor column (LMC) and axial muscle-innervating medial motor column (MMC) based on transcriptional profiles observed in developing mouse embryos56. Forkhead Box Protein (FOXP1) and Aladh1a2 (also referred to as Retinoic Acid Dehydrogenase 2 (RALDH2))33, 57 characterize LMC innervating LMNs and Limb Homeobox Containing 3 (LHX3) characterize MMC in vivo58. Fate specification studies have also shown high expression of FOXP1 is required for LMC and preganglionic motor column (PGC) specification57. Studies that overexpress this transcription factor in mES-derived MNs show successful innervation of limb skeletal muscle when implanted into the spinal cord of developing chick embryos59. While most groups25, 34, 60 report mixed populations of LMC and MMC subtypes in human hPSC-LMNs in vitro, Patani et al. (2011) show an enriched MMC identity with the omission of retinoids from the differentiation medium, and Amoroso et al. (2013) show an enhanced LMC fate with the combined use of the SHH small molecule agonist and purmorphamine. More constitutive column-specific markers are needed, as LHX3, for example, is expressed by all MNs early in development, but then LHX3 is maintained specifically in MMC subtypes, which ultimately downregulate LHX3 when they mature in vivo58, 61. In addition, hypaxial motor column subtypes remain poorly defined in vitro.
Transcriptional Molecular Profiling
Genome wide assessment of gene expression by RNA or protein profiles can provide a global, systems level measure of LMN maturity. Cross-sectional comparisons between disease and control hPSC-LMNs are routinely performed to gain insight into the ALS-induced disruptions to gene expression62, 63. However, the fidelity, and thus relevance of these models to molecular events occurring within mature LMNs in the human body remains unclear. This is due to the lack of comparisons between in vitro-derived hPSC-LMNs and in vivo-derived embryonic, fetal, adult and aged LMNs. Previous works that compare the transcriptome of iPSC-derived neural tissue to counterparts in vivo add to the consensus that iPSC-derived tissues resemble an embryonic state64, 65. However, a prolonged time in culture advances their progression towards a mature molecular signature to some extent66. A more recent study leverages transcriptomic data from the Allen Brainspan Atlas to include human brain specimens ranging from embryonic to aged adult stages65. Employing several methods to compare mRNA expression profiles with stem cell and progenitor-derived neurons, the authors conclude that despite several months of post-differentiation culture, in vitro-differentiated neural cells are restricted to an embryonic/fetal-like state. This obstacle to neural maturation may be due to a technical lack of understanding for the key elements in tissue culture to produce mature cells that are transcriptionally equivalent to their in vivo counterparts. Alternatively, this obstacle may also be due to the protracted time course of human development. Whether this obstacle to maturity also holds true for LMN cultures as well remains to be seen. Investigations comparing in vitro LMN cultures to in vivo LMNs during finely resolved developmental time points using genome wide assays such as RNA sequencing and mass spectrometry promise to provide greater insight into transcriptional and post-transcriptional mechanisms regulating LMN maturity. This could in turn guide in vitro culturing strategies to promote maturation in hPSC-LMNs and provide deeper understanding of MN development and disease.
Electrophysiology
Monitoring the electrophysiological status of MNs in vitro is currently the most comprehensive method to assess their maturation. To date, studies examining electrophysiological properties of hPSC-LMNs in vitro have focused upon basic intrinsic membrane properties and excitability of differentiating hPSC-LMNs at various time points in culture62, 63, 67, 68. In early stages, iPSC-derived neurons develop voltage-gated K+ and Na+ ion channel currents that are sufficient to generate induced action potentials. Interestingly, the nature of these action potentials develops over time (Figure 3)69. Initially induced action potentials show relatively slow depolarization and repolarization, correlating with small Na+ and K+ currents. With maturation, these action potentials become sharper as Na+ and K+ currents improve, but very few hPSC-LMNs develop the ability to fire trains of action potentials comparable to adult in vivo. Transition from simply being able to fire induced action potentials to generating spontaneous activity requires the development of intrinsic (eg. sufficiently polarized resting membrane potentials) and extrinsic (eg. synapse formation) properties that may indicate an even greater level of maturation.

Induced Action Potentials Evolve Over Time. (a) Current injection (100pA) whole-cell recordings of human iPSC-derived MNs (hPSC-MNs) over time in culture. Example action potential recordings show maturity over time in vitro as depolarization and hyperpolarization events occur more rapidly. (b) More mature hPSC-MNs in vitro display trains of action potentials (black arrows) with an abortive event (x). Action potentials displayed as change in membrane voltage (mv) over time (ms).
Even with extended time in culture, hPSC-LMNs exhibit intrinsic properties and action potential dynamics that are broadly consistent with rodent LMNs at an embryonic developmental stage70. Specifically, this is indicated by inadequately polarized resting membrane potentials, and input resistances 5–20 fold higher than adult in vivo counterparts. Functionally, this produces artificially hyper-excitable neurons, but with limited ability to spontaneously generate action potentials. Another important intrinsic property is the membrane capacitance, which is defined as the ability to store an amount of charge across the membrane for a given voltage. As the membrane capacitance is proportional to the cell surface area, it is both an important parameter for excitability and used as a crude measure for the morphological complexity of neurons. In accordance with inadequate polarization and spontaneous activity, hPSC-LMNs maintain significantly lower capacitance71 than levels observed in vivo72.
In addition to embryonic-like intrinsic properties, hPSC-LMNs show little ability to generate synapses, a critical component of a neuron’s function, as they allow the propagation of electrical signals from one neuron to the next. Further, network–driven spontaneous action potential activity is thought to drive LMN maturation and functional integration into the spinal cord73. By contrast, there is a distinct lack of network-level activity in hPSC-LMNs, which may be as a consequence of a lack of appropriate input neurons and further confounded by depolarized resting membrane potentials. Co-culture with primary cortical rodent astrocytes results in a significant improvement in both network-level spontaneous activity and basic intrinsic properties in hPSC-LMNs71. The precise role of the primary astrocytes in this case is unknown, however primary astrocytes co-culture elicits increased synaptogenesis and hyperpolarized resting membrane potentials in iPSC-derived neuronal cultures69.
The Search for Specific LMN Reporters
Cell surface antigens and florescent reporters are powerful tools for purification and analysis of LMNs in living culture systems. Several groups have generated stably integrated HB9::green fluorescent protein (GFP) transgenic reporter cell lines74. However, the utility of HB9 reporters is limited by the variable levels of GFP expression in individual cell lines and only partial overlap between GFP and other LMN markers, likely due to positional effects of transgene integration and lack of complete promoter regions for HB9 that would give more specificity. Furthermore, HB9 reporters are down-regulated over the course of maturation, complicating long-term live imaging of LMN and analysis of LMN maturation and survival.
New fluorescent reporters based on expression from Islet-1, ChAT, and VAChT promoters are being pursued for a more developmentally stable expression system. However, relatively low expression and high intracellular turnover can result in limited reporter signal. To circumvent these issues, new dual reporter constructs are being developed that combine MN-specific expression of Cre recombinase with a Cre activated reporter system. In this system, Cre recombinase is expressed under the weak MN-specific promoter; meanwhile, a second construct is inserted into a safe-harbor genomic site and includes a strong promoter downstream of a floxed transcriptional stop site that will ultimately drive a florescent reporter. When both constructs are introduced into cells, activity of the endogenous MN-specific promoter generates small amounts of Cre, which in turn acts to excise the transcriptional stop site, thereby allowing the strong promoter to permanently turn on the reporter gene in the MN. While this approach will amplify the expression of reporters in MNs and circumvent reporter down-regulation during long-term maturation studies, it will also magnify the lack of specificity associated with many currently used MN promoters. It will therefore be critical to transition from randomly integrated transgenic constructs to knock-ins of Cre recombinase into MN-specific genes. Further refinement could be achieved by the use of tamoxifen-regulated Cre fused to a modified fragment of the estrogen receptor (Cre-ERT2) instead of basic Cre. While the efficiency of recombination and MN labeling might be decreased, the ability to “pulse-label” MNs will provide a much-needed tool to study long-term MN survival by eliminating the confounding effects of ongoing MN genesis in human cultures75. In addition to these efforts to improve the available repertoire of florescent MN reporters, the identification or engineering of new MN-specific cell surface antigens for magnetic-assisted cell sorting paradigms will facilitate the purification of MNs from mixed cultures with less mechanical stress than traditional flow-cytometry approaches. While primary MNs have been successfully purified from embryonic rat spinal cord by NGF receptor p7576, identification of more specific and developmentally stable surface antigens will be required for efficient purification of human iPSC-derived MNs.
Completing the Neuromuscular Circuit
As cell type-specific derivation methods evolve, opportunities to recreate the entire neuromuscular circuit in vitro are underway (Figure 4). It is clear that filling in cellular interactions critical for MN function will make studies on hPSC-MNs more relevant to their physiology and pathology in vivo, and may enhance maturation in vitro. Indeed, mouse ESC-derived MNs are capable of maturing and forming functional neuromuscular junctions when transplanted into chick spinal cords77 and mouse sciatic nerve,78. Non-MN cell types involved in the neuromuscular circuit such as astrocytes, Schwann cells and myofibers play heavily on LMN function and may increase LMN maturity during development. The re-creation of a functional NMJ in vitro remains a coveted aim in neuromuscular research, as initial studies using primary and immortalized cell lines have had limited success. Co-culture of murine myoblast cell lines with mouse ESC-derived LMNs show deficient NMJ formation in vitro. α-bungarotoxin immunostaining, that identifies the nicotinic acetylcholine receptors enriched in functional NMJs, is lacking compared to the robust pretzel-like shape observed in vivo25, 70. Electrophysiological recordings from putative NMJs in vitro also show generally poor NMJ-induced myofibril activation. Interestingly, recent studies on xenogeneic co-culture of mouse iPSC-derived MNs and primary chick myoblasts show more typical endplate morphology and electrical function in vitro77.
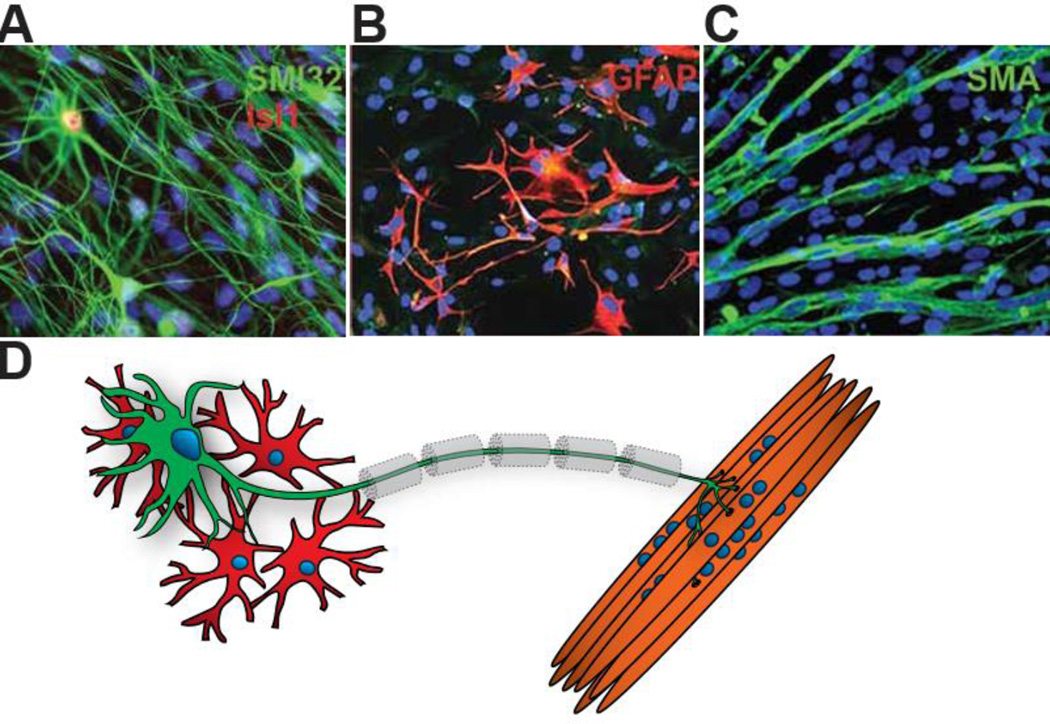
Co-Culture of Neuromuscular Circuit. (a). hPSC-astrocytes (b) taken with permission from Sareen et. al 2014. hPSC-myofibers (c) taken with permission from Hosoyama 2014. These examples of published iPSC-derived cell types could comprise of (d) a conceptualized neuronmuscular circuit. Other cell types such as myelinating schwann cells (dotted lines) and terminal schwann cells (not shown) have not yet been generated from iPSCs and may be required for functional NMJ formation in vitro. Neurofiliment Heavy chain (NFH) and specific NFH epitope SMI32, Islet-1 (Isl1), Glial fibrillary acidic protein (GFAP), Neurofiliment Heavy chain Smooth Muscle Actin (SMA).
For the first time, the concept of completely patient-derived NMJ models are now considered possible through the combination of LMN, astrocyte79, 80, and myofiber81 differentiation schemes (Figure 4). It is worth noting that additional cell types including upper MNs, schwann cells, microglia, oligodendrocytes, interneurons, monocytes, endothelial cells and others also contribute to neuromuscular circuit function and could be relevant in co-culture systems. Moreover, the potential influence of the regional identity of astrocytes in the spinal cord82, presence of specific interneuron subtypes, and functional interaction with myofibers present unique challenges compared to other neural systems in the CNS. Though these co-culture methods strive to bring us closer to the complexity inherent in all multicellular organisms, two dimensional culture systems are far from the physiological milieu of the spinal cord. Consequently, microfluidic organ-on-chip systems are now being developed where different cell types can be compartmentalized and grown juxtaposed in a 3-dimensional environment. These systems, (reviewed by Bhatia83), require very low volumes of media, allow for enhanced control of cell-cell contact and paracrine conditioning effects. If these systems could be adapted for LMN differentiation they may further enhance LMN maturity and function.
UMN Developmental Mechanisms and Directed Differentiation in vitro
Although hPSCs have theoretical potential to generate large quantities of corticospinal motor neurons (CSMN)/UMN in vitro, existing hPSC protocols are not yet capable of generating specific classes, types, or subtypes of neocortical projection neurons84. Though much more needs to be done to achieve bona fide UMN differentiation from PSCs, there has been substantial progress in our understanding of stepwise developmental molecular programs that direct neocortical projection neurons and their subtypes in mice85–87. Analyzing existing UMN derivation protocols with these newly discovered characteristics have revealed heterogeneous, neocortical-like neurons that are immature and “stalled” at a stage resembling mid-embryonic differentiation in vivo88. By applying lessons from initial mouse PSC and hPSC telencephalic, cortical neuron differentiation studies to emerging molecular understanding of UMN development described here, it is now practically possible to design more refined routes to promote UMN-specific differentiation schemes to benefit the study of UMN degeneration observed in ALS.
Telencephalic to Neocortical Development
Modeling UMNs for ALS research is not as straightforward as it has sometimes been presented, since there is immense diversity of cerebral cortex projection neurons (of which UMNs are one relatively rare and minor subtype), and ALS does not promiscuously affect the broad population of cortical projection neurons, either in humans or in ALS model mice. The diversity contained within the adult neocortex and among UMNs progressively emerges during an extended course of development. UMNs are a subtype of the neocortical projection neuron subclass of corticofugal projection neurons (CFuPN; fugal ~ flight; Latin; “away from the cortex”), and are among the very first neurons to populate the embryonic neocortex. CFuPNs are classified by their distinct axonal projections to multiple targets within or outside the neocortex85, 89, 90 (Figure 5). In an analogous situation to “default” neural induction, the rostral specification of neural tube progenitors occurs largely in the absence of caudal-derived, spinal cord morphogens (e.g., FGFs and retinoids). To a similar effect in vitro, the first successful telecephalic differentiation from mouse ES (mES) cells involved the removal of serum, and treatment with Wnt and Nodal antagonists91. Default rostral differentiation now constitutes the first step of most protocols of telencephalic differentiation by PSCs84, 92. Importantly, the identity of early rostral progenitors, characterized by the specific expression of Forkhead box G1 (FoxG1) regions93, 94 is reinforced by cell-intrinsic expression of transcriptional regulators, including Otx2, which demarcates the midbrain-hindbrain boundary and is required for early specification of forebrain and midbrain95.
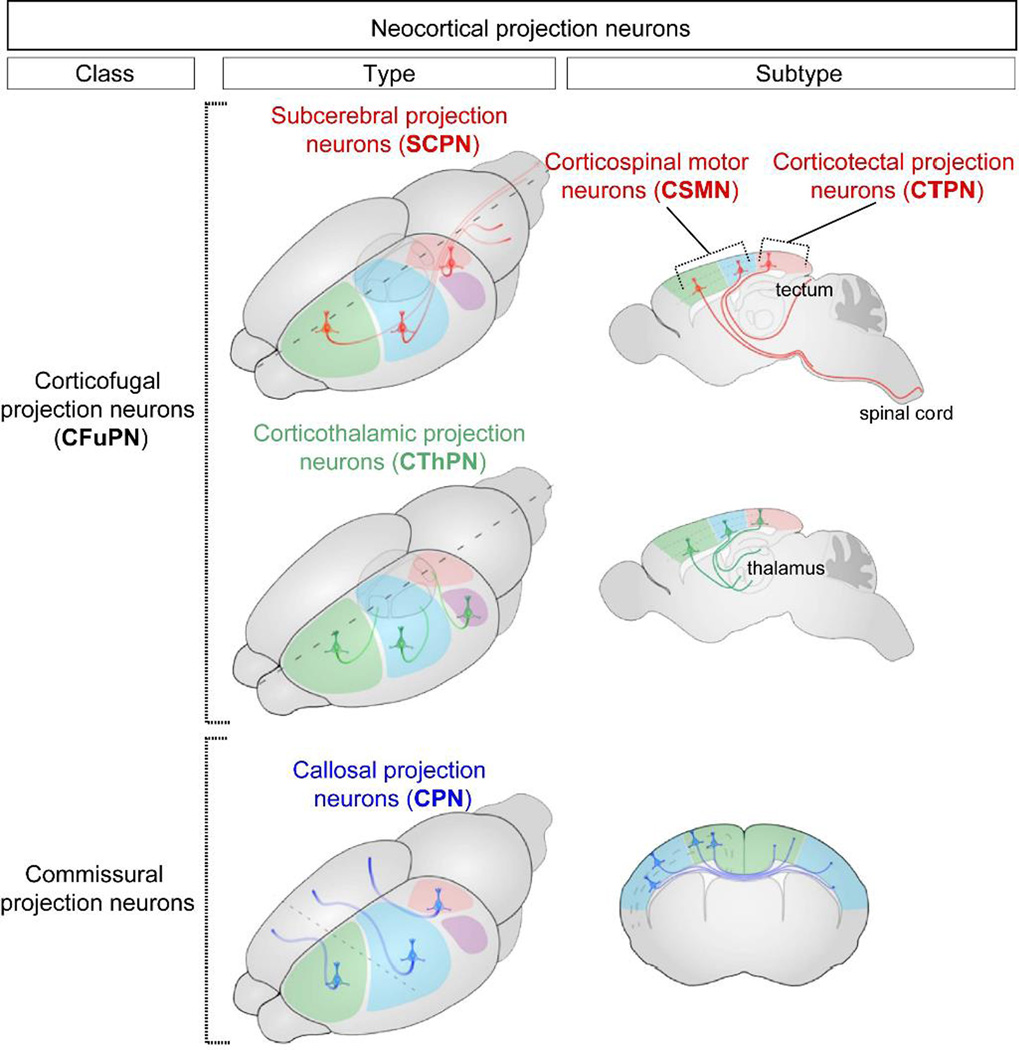
Classification of Diverse Neocortical Projection Neurons. Neocortical projection neurons can be subdivided into broad classes, types, and subtypes largely based on their axonal projections. (Figure adapted from Greig et al., Nat Rev Neurosci, 2013.) Illustrations are of the mouse brain.
There is a series of steps necessary to generate UMNs during development, making their generation from PSCs more complex than that of LMNs. The dorsal forebrain or telencephalon, called the pallium, and roughly equivalent to the neocortex (here “cortex”, “cortical”), ultimately gives rise to all (neo)cortical (“new cortex”, roughly equivalent to cerebral cortex) projection neurons, and is developmentally specified in the absence of Shh signaling and in the presence of Wnts and BMPs12, 96. Antagonism of Shh signaling can be exploited to enrich the differentiation of mES cells to dorsal, cortical fates97. This approach by Gaspard and colleagues is widely used for investigations of mouse corticogenesis in vitro96, and has been adapted to hPSCs98, 99. In contrast to mouse PSC derivation, however, Shh antagonism is reported to not similarly enhance the generation of human cortical progenitors, which seem more dependent on timing of retinoid signaling for cortical enrichment99, 100. Thus, making some type of “generic cortical neurons” appears straightforward, but these are not UMNs sufficient for ALS modeling, nor are they specific in their identity.
There is a progressive set of cross-repressive events that sequentially distinguishes projection neuron subtypes, in particular UMNs. Relatively later in mouse development, cortical-restricted transcription factor Pax6 promotes cortical fate in part by reciprocal and robust cross-repression of GS homeobox 2 (Gsh2), whose expression is restricted to the subpallium101, or ventral telencephalon. Complementary to the role of Pax6, Sox6 is also required for the proper specification of cortical progenitors, and its absence results in mis-specification, with ectopic expression of ventral genes including Achaete-Scute Family BHLH Transcription Factor 1 (Ascl1)102. Sox2 is also required for proper early cortical differentiation103, 104 (Reviewed by Georgala105), and later LIM homeobox 2 (Lhx2) is required for proper neocortical progenitor development by embryonic day 10.5 (E10.5) in the mouse106, 107. Thus, multiple transcriptional regulators expressed in the cortex (Pax6, Sox6, Lhx2 and Sox2) ultimately enable proper differentiation of neocortical progenitors, which increasingly relies on cell-intrinsic mechanisms of neocortical development (Figure 6). That said, their sequence and dose matters critically, not just their expression.
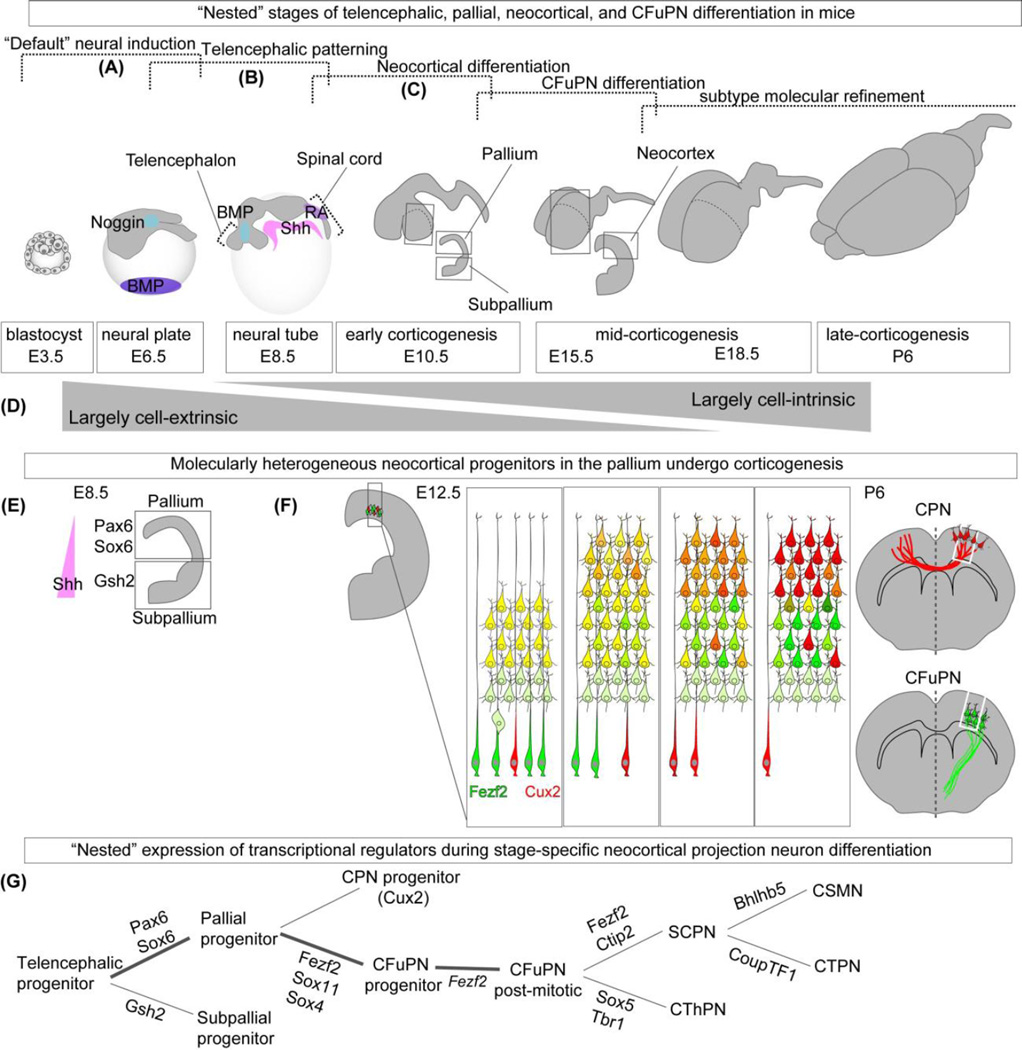
Cell-extrinsic and Cell-intrinsic Factors Regulate the Development of CFuPN within Sequential, “Nested” Stages of Differentiation. (a) “Default” neural and rostral differentiation occurs by repression of alternate signaling pathways induced by multiple morphogens (e.g., Noggin inhibits BMP signaling during neural plate formation at ~E3.5–E6.5 in mice; cortical progenitors require low or absent expression of caudalizing retinoids (RA) and ventralizing Shh at ~E6.5–E8.5). (b) The dorsal aspect of the telencephalon is called the pallium, which gives rise to the neocortex. In contrast, the ventral telencephalon is called the subpallium. The delineation of these two telencephalic progenitor domains occurs between ~E8.5 and ~E10.5. (c) During corticogenesis, beginning at ~E10.5 in mice, multiple diverse cortical projection neuron classes, types, and subtypes are sequentially generated from cortical progenitors. These projection neurons become refined with continued maturation through post-natal ages. (d) Early stages of corticofugal projection neuron (CFuPN) differentiation are largely mediated by cell-extrinsic factors, whereas later stages of are largely mediated by cell-intrinsic factors. (e) Following Shh-mediated dorsal-ventral patterning of the telencephalon, cortical and ventral identities are reinforced by transcriptional regulation (Pax6 and Sox6 in the pallium; Gsh2 in the ventral areas). (f) Early cortical progenitors give rise to more definitive (neo)cortical progenitors, which generate projection neuron subtypes at ~E10.5. CFuPN populate the deep layers of the cortex. Later-born CPN populate both deep and superficial layers of cortex. Molecular distinction of CPN and CFuPN occurs with continued maturation (represented by transition from yellow, dual-marker expression to red or green single-marker expression). (g) “Nested” expression of distinct transcriptional regulators at distinct developmental stages promotes stepwise CFuPN and thus UMN differentiation.
The specification, post-mitotic differentiation, axon guidance, and axon pruning of UMNs are largely controlled by the transcriptional activities of FEZ family zinc finger 2 (Fezf2), COUP-TF interacting protein 2 (Ctip2)/B-cell CLL/lymphoma 11B (BCL11B), and Orthodenticle Homeobox 1 (Otx1)108–110. Although these three transcription factors are initially expressed both by UMNs and by corticothalamic projection neurons (CThPN) at lower doses, the dose and timing of their expression controls UMN specificity. First, Fezf2 is necessary for the specification of UMN and the broader set of related subcerebral projection neurons (SCPN), but not of CThPN (though they develop abnormally in the absence of Fezf2). In the absence of Fezf2, downstream Ctip2 transcription factor expression is lost, and prospective UMNs instead differentiate into deep layer commissural neurons and CThPN111. Second, Ctip2 is critical for the post-mitotic development of UMN / SCPN, and, in its absence, UMNs do not project to the spinal cord, with additional defects in axon outgrowth, fasciculation, and guidance. Third, Otx1 is specifically expressed by post-mitotic UMN, and directs pruning of SCPN axonal projections during late postnatal maturation112. Together, Fezf2, Ctip2, and Otx1 are essential for the proper differentiation of UMN/SCPN, and the specifics of UMN development might be critical to appropriate ALS modeling.
Overcoming Deficits in Directed UMN Differentiation
Observations by Sadegh and Macklis88 describe distinct deficits of mES cell-derived cortical-like progenitors and neocortical-like neurons that are likely shared by those derived from human iPSCs; however, direct species comparisons are lacking. Current mES cell-derived cortical-like progenitors are more heterogeneous than in vivo counterparts, and seemingly include many incorrectly specified progenitors indicative of both cortical and general forebrain fates. Additionally, current mES cell-derived neurons are not uniformly or completely mature, but even the most advanced display crucial hallmarks of early maturation roughly equivalent to mouse neocortical neurons in vivo at E16.5-E18.5. Specifically, these neurons co-express multiple transcription regulators (e.g., T-box, brain 1 (Tbr1), Ctip2, and SATB homeobox 2 (Satb2)), which are consistent with immature neocortical projection neuron expression signatures at an equivalent in vivo stage of mid-corticogenesis. Finally, these “stalled” neocortical-like neurons appropriately co-express certain post-mitotic regulators of area-specific differentiation (such as, COUP transcription factor 1 (CoupTF1), basic helix-loop-helix domain containing, class B5 (Bhlhb5), which may indicate deficits in area-specific cortical differentiation. These regulators specify cortical motor neurons and cortical visual neurons, the latter of which is not involved in ALS. It is clear that current UMN derivation methods are in nascent stages in vitro. Multiple cell-intrinsic and cell-extrinsic processes, however, including focal chromatin remodeling, post-translational modifications of transcriptional regulators, cell-cell contacts, and paracrine molecular signaling, regulate the stepwise generation of UMNs in vivo, and provide promising leads that remain largely untested in hPSC-derived cultures.
Additional layers of control might be required to generate reasonable UMNs from PSCs for modeling. During the sequential generation of neocortical projection neuron subtypes, the refinement and maturation of neocortical progenitors and post-mitotic neurons are progressively shaped by multiple epigenetic changes, including histone remodeling and methylation, DNA methylation, and regulation by non-coding RNA113. The roles of histone deacetylases have been identified in multiple forebrain cell types, including retinal subtypes114 and in cortical neurons113, 115. A number of neocortical subtype-specific transcriptional regulators (e.g. Ctip2, Satb2, Ski) can directly interact with chromatin remodeling enzymes, implicating epigenetic mechanisms for cortical subtype specification and maturation. In addition, post-translational modifications (e.g. phosphorylation, SUMOylation) of critical transcription factors might be important for the regulation of subtype specification, and possibly for directed UMN differentiation and maturation. For example, the SUMOylation of Ctip2, shown in hippocampal neurons116, and Satb2, in non-neocortical cells117, might be important for regulating precise subtype specification in the neocortex. Thus, these post-translational modifications of critical transcriptional regulators are potentially useful for the assessment of appropriate UMN specification from pluripotent stem cells.
Though established monolayer protocols offer advantages for high-throughput optimization and therapeutic screening, the absence of three-dimensional cell-cell interactions in monolayer culture might impede subtype-specific refinements. Notch signaling is one of the best-studied mechanisms of cell-cell signaling in the cortex, and is particularly relevant to directed differentiation, given its longstanding roles in delineating distinct identities in related cell types118, including cortical migration119, neurogenesis120 and asymmetric cell division121. Consistent with this hypothesis, an established embryoid body protocol of forebrain differentiation generates neurons with seemingly reduced co-expression of distinct cortical subtype markers122, 123. Despite the apparent benefits of embryoid body culture, distinct deficits in neuronal laminar organization indicate that cell-cell contacts are either incompletely replicated or insufficient in embryoid body culture without additional factors and/or regulatory mechanisms.
Further complicating UMN modeling, numerous cell-extrinsic factors are required for cortical neuron development in vivo (reviewed by Tiberi96). Among these factors, a number of them are subtype-specific. First, the well-studied endo-cannabinoid signaling pathway has been recently identified to be critical for maintenance of proper UMN subtype124. Second, insulin-like growth factor 1 (IGF-1) specifically activates and enhances rate of extension of UMN axons125. Moreover, multiple other factors, including IGF-2 and BDNF, enhance survival of cultured UMN126. Finally, mutant mice lacking choroid plexus have a reduction in UMNs127. This phenotype is striking because it suggests that one or more of multiple growth factors secreted in the cerebral spinal fluid (e.g. IGF-1, IGF-2) are critically important for molecular refinement of UMN identity128. Similar to enhanced LMN generation protocols, the concentration and timing of these and non-subtype specific factors such as Fgf8122 and retinoids99 may also benefit from high-throughput optimization studies to increase UMN generation efficiency and function in vitro.
Methods to Accelerate MN Maturation and Aging in vitro
It is clear that in both human development and in vitro, LMNs and UMNs require significantly more time for neurogenesis and maturation to occur than murine counterparts. Aside from apparent challenges such as increased time in culture, study length and cost, the ability of in vitro hPSC-derived cells to exhibit mature, native functional properties are crucial for accurate in vitro modeling of adult-onset diseases. An interesting approach to artificially accelerate the aging process genetically manipulates iPSC-derived neurons to express progerin, a mutated form of lamin A, that causes the premature aging disease Progeria129. Upon progerin overexpression, iPSC-derived dopaminergic neurons exhibit age-associated changes, however the non-age related effects of artificial Lamin A expression in neurons is unknown. By interfering with Notch signaling, small molecule γ-secretase inhibitors, namely DAPT and Compound E, have been shown to accelerate neuronal differentiation by delaying G1/S phase long enough to commit neurons to neurogenesis130, 131. These inhibitors have been shown to be effective in hPSC-LMN protocols as well25. While these compounds have proven useful in promoting cell cycle exit, the consequences of this treatment in MN diversity is unexplored. As different MN subtype fates emerge at distinct developmental timepoints in vivo, premature cell cycle exit could obstruct later MN subtype programs.
An alternate method to generate human MNs in vitro is described as induced-MNs (iMN)28. This process circumvents reprogramming to pluripotency by directly converting patient somatic cells to MNs through transgenic expression of transcription factors that drive MN differentiation. By avoiding the epigenetic “reset” that occurs during reprogramming to pluripotency132, this approach has been shown to maintain age-related epigenetic signatures accrued over the lifetime of the patient133. iMNs display unique age-related cellular defects not observed in hPSC-MN approaches. However, these approaches are challenged by genomic instability resulting from increased somatic cell expansion requirements, as well as deficient MN maturation in vitro.
It is widely accepted that the maturation status of iPSC-derived cells remains a significant hurdle to the field of regenerative medicine at large, and yet it remains largely under-explored in humans4. The ability to harness cell signaling to promote maturation in vitro, rests on increased understanding of anatomical, molecular and electrophysiological data from fetal, adult and aged human spinal post mortem tissue. Projects such as the Allen Brain Atlas provide valuable templates to guide anatomical- and genomic-level evaluation of native human MN maturation and aging. However, detailed functional data on human MNs are lacking and therefore, comparative evaluation of functional maturity relies largely upon the characterization of other mammalian species, often at cost to fidelity.
Can we Currently Model ALS using iPSC-derived MNs?
Only a small percentage (10%) of ALS cases are inherited (termed familial ALS, or fALS). A common strategy to study diseases with this familial signature is to interrogate specific causal gene mutations within this population for disease mechanisms that can then be applied to the patient population as a whole. Traditional murine transgenic models of ALS have been extensively studied (reviewed by Swarup, V. & Julien134), however treatments developed in these models have not been effective in human trials indicating a need for the study of human tissue. Human LMNs differentiated from iPSCs that had been generated from an ALS patient carrying a mutation in the Super Oxide Dismutase 1 (SOD1) gene135 provided the groundwork for subsequent fALS disease modeling studies in vitro. More recently, separate iPSC studies from SOD1A4V mutants, an aggressive form of fALS, have yielded distinct phenotypes. Significant ISL1+ cell loss, reduced neuronal soma size and increased apoptotic cells are observed in one study with hPSC-LMNs cultured for 30 days post-differentiation. Comparative RNA-sequencing of genetically corrected isogenic controls reveal distinct mitochondrial and transport dysfunction in the non-corrected lines62. Meanwhile, another group using a similar isogenically controlled approach show that mutant SOD1 binds to neurofilament mRNA and leads to neurite swelling and degeneration, but that mutant SOD1 does not induce cell death in vitro136.
ALS research progressed with the recent discovery of a mutation in the Chromosome 9 Open Reading Frame 72 (C9Orf72) gene137. This mutation, involving intronic hexanuclotide repeat expansion, was identified as the most common form of familial ALS and fronto-temporal dementia, accounting for approximately 40% of familial and 8–10% of sporadic cases137, 138. Unlike SOD1 transgenic mouse models, C9ORF72 mutant mice have not yet revealed disease-specific MN loss in vivo. To study C9ORF72 mutant effects on human tissue, investigators have generated iPSCs from C9Orf72 patients. In contrast to SOD-1 mutant studies, three recent investigations with C9Orf72 mutant hPSC-LMNs report no overt LMN loss in vitro63, 68, 139. These hPSC-MN cultures do, however, exhibit intranuclear C9Orf72 repeat-containing RNA foci detected by florescent in situ hybridization indicating a disease-specific physiological phenotype independent of overt cell death. These foci can be partially resolved using antisense oligonucleotides that target the hexanuclotide expansion63, 139. Additionally, the hPSC-LMN cultures derived from C9ORF72 patients display RNA binding protein interactions and repeat-associated non-ATG (RAN) proteins, along with a high susceptibility to glutamate-mediated excitotoxicity139.
An important phenotypic distinction between fALS disease modeling studies lies within neuronal excitability. Altered LMN excitability has long been associated with ALS pathogenesis in rodent models140–142 and patients143, 144, and mutant SOD1 and C9Orf72 patient-derived hPSC-LMNs have also been shown to demonstrate a hyperexcitable state. In these experiments, the hyperexcitability phenotype was shown to be effectively attenuated by the small molecule Retigabine, an activator of Kv7 currents71. In contrast, another group noted a hypoexcitable state in C9Orf72-derived cultures63. As a result, hPSC-LMN membrane excitability phenotypes in relation to ALS have remained an area of dispute. Recently, however, temporal analysis studies using iPSCs derived from both familial C9Orf72 and TAR DNA-binding protein (TARDBP) mutants have demonstrated that both hyper- and hypo-excitable phenotypes exist at distinct time-points of culture maintenance68. Specific subsets of C9Orf72 and TARDBP patient-derived LMNs classified according to their firing state exhibited a hyperexcitable state early during maturation, followed by a hypoexcitable state after 9 weeks in culture with a loss of both voltage-activated Na+ and K+ currents. fALS patient-derived hPSC-LMNs also showed progressive loss in synaptic activity in accordance with ALS patient data and rodent models68. While this study seems to have resolved the inconsistencies between competing functional phenotypes, discrepancies in hPSC-LMN vulnerability in vitro are still confounded by differing hPSC-LMN derivation and culture techniques between investigators.
Altered excitability in LMNs as evidenced by these studies offers a plausible role within ALS disease progression. However, further experimental support of these findings is required to determine whether reported phenotypes are pathogenic or simply homeostatic responses to culture conditions, particularly a reduced synaptic input. To add to this complex dynamic, recently published electrophysiological studies of SOD1G93A mouse LMNs demonstrate that the subclass of immediate firing LMNs that innervate slow-contracting fibers (S-type MNs), which do not degenerate in the SOD1G93A mouse model of ALS, show reversed hyperexcitability whilst the vulnerable subclass of delayed firing LMNs that innervate fast-contracting fibers (F-type MNs) do not show altered excitability145, 146. These data further highlight the need for an enhanced grasp of the specific cellular identity of LMNs in vitro to correctly establish whether altered LMN excitability contributes to ALS disease progression.
The contribution of UMN hyperexcitability in the pathogenesis of ALS is an area of intense investigation in both clinical and laboratorial settings. Studies in transgenic ALS mice have observed evidence of hyperexcitable UMNs that precede the onset of motor symptoms and lower motor neuron degeneration147. The selective suppression of mutant SOD1 in the motor cortex of SOD1G93A ALS mice can delay overall disease onset148. In the clinic, the presentation of cortical hyperexcitability is well documented149 (reviewed by Bae)150. Though retigabine is effective in reducing excitability in hPSC-derived LMNs, its efficacy remains largely untested in newer upper motor neuron derivation protocols. Elucidating UMN-specific mechanisms in ALS in vivo and in vitro will be crucial for the field moving forward.
Conclusion
While the complete recapitulation of human ALS pathology in vitro is admittedly a lofty prospect, these studies demonstrate the utility of disease modeling technology available today. As these applications are developed across different laboratories, it is crucial that stringent criteria emerge to adequately compare results among investigations. These include: maturation, molecular characterization, electrical and transcriptional function of iPSC-derived MNs, as well as their interaction with other cell types in vitro. However, the relevance of these models will ultimately be determined by their ability to translate to real treatment for patients. Though functional and molecular phenotypes associated with ALS patient-derived hPSC-MNs have been observed, successful treatments in vitro have yet to meet this ultimate goal. Critically, the need to address population diversity in these models is becoming more apparent. While early proof-of-concept studies only represent up to a few patients, a requirement that disease phenotypes be supported by a more significant sample size are being addressed. In the short term, increasing sample size to 4–10 individuals will have an appreciable effect on the strength of observed ALS-related phenotypes. Beyond this, efforts are underway to generate iPSCs from large patient populations will allow future studies to more adequately represent diverse patient pools. To capitalize on these large iPSC banks, a single, unified method for studying human MNs ex vivo may help to accelerate therapy development by enabling joint efforts that can capitalize on diverse strengths in the research community. However, there is still work to be done in defining what that method should be; given the rapid developments in the field of neural differentiation at the present time.
In spite of these uncertainties, it is clear that progress in modeling ALS and in turn, our understanding of this terminal disease, rests on replicating phenotypes across multiple investigators, maturing cultures to emulate aged tissue, and enhanced methods to assess experimental data generated from patient-derived cultures. With collaborative approaches among researchers aligned in the interests of human biology and improving patient care, these objectives within reach.
Acknowledgments
We would like to thank Dr. Soshana Svendsen for assistance in editing this manuscript. All authors were funded by various grants from the ALS Association. The corticospinal motor neuron / UMN / cortical projection neuron work of Macklis and Sadegh was supported by NIH grants R01NS075672, R01NS045523, R01NS049553, and R37NS041590, and by grants from the ALS Association and Spastic Paraplegia Foundation. Work of Wichterle and Lowery were also funded in part by Project ALS and Track ALS. Contributions from Zhang were funded by ALS Association grant 15-IIP-194.
References
Full text links
Read article at publisher's site: https://doi.org/10.1038/nn.4273
Read article for free, from open access legal sources, via Unpaywall:
https://europepmc.org/articles/pmc5015775?pdf=render
Citations & impact
Impact metrics
Article citations
Polyethyleneimine facilitates the growth and electrophysiological characterization of iPSC-derived motor neurons.
Sci Rep, 14(1):26106, 30 Oct 2024
Cited by: 0 articles | PMID: 39478194 | PMCID: PMC11525838
How is Excitotoxicity Being Modelled in iPSC-Derived Neurons?
Neurotox Res, 42(5):43, 15 Oct 2024
Cited by: 0 articles | PMID: 39405005 | PMCID: PMC11480214
Review Free full text in Europe PMC
Formation and Long-Term Culture of hiPSC-Derived Sensory Nerve Organoids Using Microfluidic Devices.
Bioengineering (Basel), 11(8):794, 05 Aug 2024
Cited by: 0 articles | PMID: 39199753 | PMCID: PMC11352057
Expanded ATXN1 alters transcription and calcium signaling in SCA1 human motor neurons differentiated from induced pluripotent stem cells.
Neurobiol Dis, 201:106673, 20 Sep 2024
Cited by: 0 articles | PMID: 39307401
Molecular pathology, developmental changes and synaptic dysfunction in (pre-) symptomatic human C9ORF72-ALS/FTD cerebral organoids.
Acta Neuropathol Commun, 12(1):152, 18 Sep 2024
Cited by: 0 articles | PMID: 39289761 | PMCID: PMC11409520
Go to all (156) article citations
Similar Articles
To arrive at the top five similar articles we use a word-weighted algorithm to compare words from the Title and Abstract of each citation.
Modeling amyotrophic lateral sclerosis in pure human iPSc-derived motor neurons isolated by a novel FACS double selection technique.
Neurobiol Dis, 82:269-280, 21 Jun 2015
Cited by: 17 articles | PMID: 26107889
A cellular model for sporadic ALS using patient-derived induced pluripotent stem cells.
Mol Cell Neurosci, 56:355-364, 25 Jul 2013
Cited by: 172 articles | PMID: 23891805 | PMCID: PMC4772428
Modeling hallmark pathology using motor neurons derived from the family and sporadic amyotrophic lateral sclerosis patient-specific iPS cells.
Stem Cell Res Ther, 9(1):315, 15 Nov 2018
Cited by: 30 articles | PMID: 30442180 | PMCID: PMC6238404
ALS, a cellular whodunit on motor neuron degeneration.
Mol Cell Neurosci, 107:103524, 03 Jul 2020
Cited by: 5 articles | PMID: 32629110
Review
Funding
Funders who supported this work.
NIDDK NIH HHS (1)
Grant ID: P30 DK063491
NIGMS NIH HHS (1)
Grant ID: T32 GM007753
NINDS NIH HHS (9)
Grant ID: R01NS045523
Grant ID: R01NS049553
Grant ID: R37 NS041590
Grant ID: R01 NS045523
Grant ID: R01 NS041590
Grant ID: R01NS075672
Grant ID: R01 NS049553
Grant ID: R37NS041590
Grant ID: R01 NS075672