Abstract
Free full text

α3β1 Integrin suppresses prostate cancer metastasis via regulation of the Hippo pathway
Abstract
Existing anti-cancer strategies focused on disrupting integrin functions in tumor cells or tumor-involved endothelial cells have met limited success. An alternative strategy is to augment integrin-mediated pathways that suppress tumor progression, but how integrins can signal to restrain malignant behavior remains unclear. To address this issue, we generated an in vivo model of prostate cancer metastasis via depletion of α3β1 integrin, a correlation observed in a significant proportion of prostate cancers. Our data describe a mechanism whereby α3β1 signals through Abl family kinases to restrain Rho GTPase activity, support Hippo pathway suppressor functions, and restrain prostate cancer migration, invasion, and anchorage-independent growth. This α3β1-Abl kinase-Hippo suppressor pathway identified α3 integrin-deficient prostate cancers as potential candidates for Hippo-targeted therapies currently under development, suggesting new strategies for targeting metastatic prostate cancer based on integrin expression. Our data also revealed paradoxical tumor suppressor functions for Abl kinases in prostate cancer that may help to explain the failure of Abl kinase inhibitor imatinib in prostate cancer clinical trials.
Introduction
Changes in cell-extracellular matrix interactions are hallmarks of prostate cancer progression (1,2), yet our understanding of the mechanistic contributions of these events is far from complete. For example, altered integrin expression has been noted in prostate cancer (1,2); however, translating knowledge of changes in integrin expression and function into the clinical setting has proved challenging. Thus far, αv integrin targeting agents have been tested clinically in prostate cancer, and multiple trials using an anti-αv antibody, or ligand-mimetic peptide failed to show clinical benefit (3–5). Existing integrin-directed therapeutic strategies disrupt integrin function, but integrins can also exert tumor suppressive activities (6–8). Therefore, an alternative yet neglected approach would be to augment integrin-mediated tumor suppressor functions. In prostate cancer, reduced α3 integrin expression was suggested to associate with a more aggressive phenotype (9), but whether α3 downregulation contributes mechanistically to prostate cancer progression was unknown. Our recent study revealed that loss of α3 integrin can indeed promote prostate cancer colonization of metastatic sites (10), but did not identify the signaling mechanism by which α3 integrin restrains malignant behavior or whether α3 functions at earlier stages in prostate cancer progression.
The Hippo tumor suppressor pathway is a master integrator of multiple extracellular inputs, including cell adhesion and detachment, cell-cell contacts, and growth factor signaling (11–13). Although the Hippo pathway is clearly influenced by cell adhesion, aside from E-cadherin, few specific adhesion receptors have been identified as upstream inputs. We now provide evidence of an α3 integrin-Abl kinase-Hippo suppressor pathway in prostate cancer in which α3β1 integrin signals through Abl kinases to restrain Rho GTPase activity, sustain Hippo suppressor functions, and curtail metastatic cell phenotypes. Importantly, multiple members of this novel α3-Abl-Hippo pathway are amenable to pharmacological manipulation, suggesting new strategies for targeting metastatic prostate cancer based on integrin expression.
Materials and Methods
Cell Culture
GS6889.Li cells, a metastatic subline of PC-3 prostate carcinoma cells created in 2009 (14), were re-authenticated in 2016 for this study by STR analysis (IDEXX Bioresearch). DU-145 prostate carcinoma cells, originally obtained from ATCC in 2005, were cultured for fewer than 20 additional passages from the original stock during the course of the present study. GS689.Li cells and DU-145 cells were cultured in DMEM:F12 with 10% FBS. Cells were transduced with a pQCXIN retroviral expression vector encoding luciferase, and a pQCXIP retroviral vector encoding GFP.
Antibodies and Reagents
Antibodies used were: α3 integrin, A3-X8 (8); A3-CYT (15); and HPA008572, (Sigma-Aldrich); RhoA (ARHO1, Cytoskeleton Inc); RhoC, (D40E4, Cell Signaling Technology); actin (AC-15, Sigma-Aldrich); LATS1 C66B5, YAP/TAZ D24E4 (Cell Signaling Technology); TAZ/WWTR1 (1H9, Lifespan Biosciences, Inc); YAP1 (H-125, Santa Cruz Biotechnology); CTGF (L-20, Santa Cruz Biotechnology); β-catenin (610153, BD Biosciences); p190RhoGAP (D2D6, Millipore); phosphotyrosine (P-Tyr-100, Cell Signaling Technology); p120RasGAP (B4F8, Santa Cruz Biotechnology); Crk (22/Crk, BD Biosciences); phospho-Crk/CrkL, (#3181, Cell Signaling Technology); Arg/Abl2 (A301-986A, Bethyl Laboratories, Inc); andFAK and phospho-FAK (77/FAK and 18/FAK (pY397), BD Biosciences). Secondary reagents were PE-goat anti-mouse, Alexa 680 goat anti-mouse, Alexa 790 goat anti-rabbit, and Dylight 800 Neutravidin (Thermo Fisher Scientific).
Other reagents were D-luciferin (Gold Biotechnology), rat tail collagen I (Corning), C3 transferase (Cytoskeleton, Inc), lysophasphatidic acid (LPA) (Avanti Polar Lipids), Y-27632 (Enzo Life Sciences), blebbistain (Selleckchem), imatinib (Cayman Chemical), DPH [5-(1,3-diaryl-1H-pyrazol-4-yl)hydantoin, 5-[3-(4-fluorophenyl)-1-phenyl-1H-pyrazol-4-yl]-2,4-imidazolidinedione] (Sigma-Aldrich), and PF-573228 (Tocris).
RNA Interference
α3-KD, LATS sh1-sh4, shYAP, shTAZ, YAP sh1, YAP sh2, and Arg retroviral shRNA constructs had a pSIREN RetroQ vector backbone (Clontech); α3-KD2, TAZ sh1-sh3, and Abl sh1-sh3 constructs had a pZIP-mCMV-ZsGreen backbone (Transomics Technologies). RhoA, RhoC and LATS sh5 and sh6 constructs had a pLKO backbone (Sigma-Aldrich). A non-targeting shRNA in the appropriate vector backbone was included to produce vector control cell lines. Cells were maintained as stably transduced, polyclonal populations. See Supplementary Information or RNAi targeting sequences.
Orthotopic Prostate Cancer Models
Animal protocols were approved by the University of Iowa Animal Care and Use Committee (Approval #5031328). 50,000 GS689.Li cells were implanted in the left anterior lobe of the prostate of 8 SCID/NCr (BALB/C) mice/cell line. Bioluminescent imaging (BLI) was performed using an Ami X imaging system (Spectral Instruments Imaging) as described (10). Upon sacrifice, livers, kidneys, and lungs were dissected for analysis of disseminated cells by fluorescence microscopy. Liver and kidney colonization was imaged with an Olympus SZX12 stereomicroscope. To image lung metastases, one lobe of each lung was cut into ~3–5 mm pieces and flattened between two glass slides. Ten random fields/lung were photographed using the 10X objective of a Leica DMIRE2 inverted microscope. Areas occupied by GFP-labeled cells were determined using the ImageJ Threshold command followed by the Analyze Particle command (size range 50.00-Infinity μm2). A similar approach was used to quantify kidney and liver colonization except that the data were converted to %GFP-positive area by dividing by the total area of each kidney or liver. Anterior and posterior aspects of each liver and dorsal and ventral aspects of each kidney were examined.
In vitro Tumor Growth Assays
3D collagen growth assay
300 μl of collagen I (0.8 mg/ml in DMEM) was polymerized per well in 24 well plates for 45 min at 37°C. 15,000 cells/well were seeded in 500 μl of defined growth medium (DGM) (DMEM:F12, 5 mg/ml BSA, 2 mM L-glutamine, 100U/ml penicillin, 100 μg/ml streptomycin, 0.1 mM non-essential amino acids, 25 mM HEPES pH 7.2, plus insulin-transferrin-selenium (ITS) supplement). After 7–10 days, cell number was quantified by BLI. Six wells/cell type/condition were quantified in each trial.
Detached growth assay
24 well plate were treated twice with 100 μl/well of 20 mg/ml poly-HEMA [Poly(2-hydroxyethyl methacrylate)] in 95% ethanol, which was allowed to evaporate. Wells were rinsed with PBS and 15,000 cells were seeded per well in 500 μl of DGM. After 7–10 days, cell growth was quantified by BLI.
Starvation growth assay
15,000 cells per well were plated in uncoated 24 well plates in serum free medium (SFM) (DMEM:F12, 5 mg/ml BSA, 2 mM L-glutamine, 100U/ml penicillin, 100 μg/ml streptomycin, and 0.1 mM non-essential amino acids, 25 mM HEPES pH 7.2, no ITS supplement). After 7–10 days, cell growth was quantified by BLI.
Time-lapse Microscopy and Transwell Migration Assays
2.3 X 105 cells were plated in SFM on 35 mm dishes coated with 10 μg/ml rat tail collagen I. Time-lapse images were acquired as described (15). ImageJ software was used to record the XY position of 50–70 cell centroids in frames 5 min apart and determine the migration speed, using the mTrackJ plugin (16). In the cell contraction assay, serum starved cells were stimulated with 10% FBS, and cell areas were measured in frames 1 min apart using ImageJ.
For matrigel invasion, 25,000 cells per well were seeded in SFM in the upper wells of BD BioCoat Matrigel invasion chambers. SFM with 5 μM LPA was placed in the lower well. After 24 h, cells were stained with Cell Tracker Red (ThermoFisher Scientific), and non-invaded cells were removed using a cotton swab. Invaded cells were quantified in 4 fields/chamber representing peripheral and central parts of the chamber.
Rho Activity Assays
Confluent 100 mm dishes were serum-starved overnight and stimulated with 5 μM LPA for indicated amounts of time. Cells were lysed with ice cold 1% Triton X-100, 0.1% SDS, 10 mM MgCl2 with protease inhibitors, and activated Rho was recovered by pulldown with GST-Rhotekin fusion protein, followed by immunoblotting for active and total Rho, as described (17).
Immunoblotting and Immunoprecipitation
Immunoprecipitations and blotting were performed as described (15) and quantified using a LiCOR Odyssey blot imager. For immunoprecipitation of p190RhoGAP complexes, cell lysates were prepared in 1% Triton X-100 detergent. For analysis of YAP and TAZ protein in cells growing under detached conditions, cells were grown in DGM in suspension over poly-HEMA for 4–11 days prior to lysis.
Immunohistochemistry
Antigen unmasking was in citrate buffer, pH 6. Endogenous peroxidase was quenched with 3% H2O2, and either 1.5% horse serum (α3 integrin) or 10% goat serum (TAZ and YAP) was used to block non-specific staining. Sections were incubated with rabbit anti-α3 integrin (HPA008572) at 1:20, mouse anti-WWTR1/TAZ (1H9) at 1:100 or rabbit anti-YAP (Santa Cruz sc-15407) at 1:100 for 1 hour. Slides were incubated with secondary antibody and detection reagent (DAKO Rabbit Envision HRP System reagent for 30 minutes for α3 integrin and YAP, and biotinylated anti-mouse IgG at 1:500 followed by ABC for TAZ). Slides were developed with DAKO DAB plus for 5 minutes, DAB Enhancer for 3 minutes, and counterstained with hematoxylin.
Quartile staining system: for α3 integrin, 3 = strong basal staining typical of benign glands; 2 = reduced, delocalized staining; 1 = faint, discontinuous staining; and 0 = essentially absent staining. For TAZ, 3 = most nuclei show strong staining, clearly higher than cytoplasmic staining; 2 = moderate staining, majority of nuclei still clearly positive; 1 = faint staining, many nuclei appear negative; 0 = absence of nuclear staining characteristic of the luminal cells of most benign glands (note that proliferative basal cells in benign glands are TAZ positive). For YAP, 3 = most nuclei show strong staining, clearly higher than cytoplasmic staining; 2 = intermediate staining, some nuclei appear more highly stained than adjacent cytoplasm; 1 = faint staining, a few scattered nuclei appear more highly stained than adjacent cytoplasm; 0 = faint cytoplasmic staining, unstained nuclei characteristic of luminal cells of benign glands.
Results
Loss of α3 integrin promotes prostate cancer progression and metastasis and growth under low-anchorage and low-growth factor conditions
To investigate the contribution of α3 integrin to prostate cancer progression and spontaneous metastasis, we inoculated luciferase and GFP-labeled α3-depleted (α3-KD) and vector control (NT) cells in the left anterior lobe of the mouse prostate. At 28 days post-inoculation, mice harboring α3-KD cells showed dramatically increased apparent tumor burdens by BLI (Fig. 1A). The α3-KD cells showed significantly higher BLI signal from Day 22 to Day 35, at which time the mice harboring α3-KD cells had reached a pre-defined BLI endpoint of >109 photons/sec total tumor burden (Fig. 1B, annotation “a”). Flow cytometry confirmed that α3 integrin was ~90% depleted in the α3-KD cells (Fig S1), as reported (10).
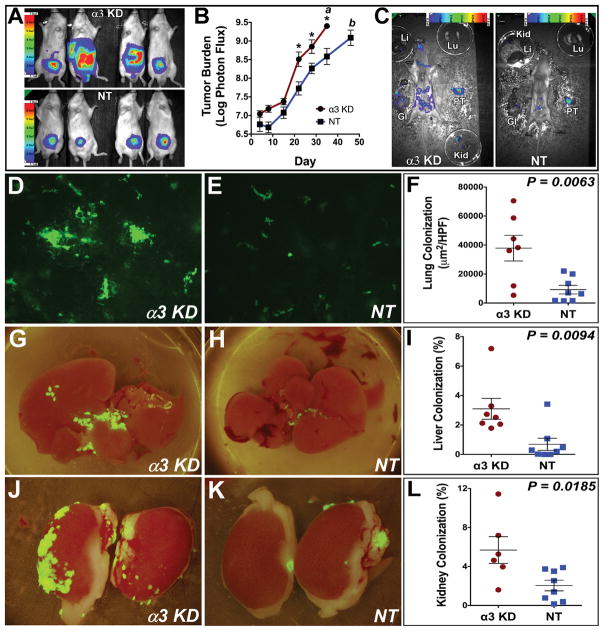
(A) Bioluminescence imaging (BLI) of orthotopic tumors 28 d post-implantation. Color scale indicates photons/sec/cm2/sr. (B) Tumor burden versus time. Annotation “a”: mice harboring α3-KD tumors and one mouse harboring an NT control tumor were sacrificed on day 35. Annotation “b”: the remaining mice harboring NT control tumors were sacrificed on day 46. *P=0.0004, P=0.007, and P=0.001 on days 21, 28, and 35 respectively, Holm-Sidak multiple comparison test with α=0.05. (C) Gross necropsies of mice harboring α3-KD and NT control tumors on day 35. Tumor burden of both mice was ~1 X 109 photons/sec. PT, primary tumor; GI, gastrointestinal tract; Kid, kidney; Li, liver; Lu, lung. (D–E) Representative images of GFP-labeled α3-KD and NT control lung metastases. (F) Quantification of lung metastasis as μm2 GFP-positive cells per high-powered field (HPF). P=0.0063, unpaired t test. (G–H) Liver colonization by GFP-labeled α3-KD and NT control cells. (I) Quantification of liver colonization as % total surface area occupied by tumor cells. P=0.0094, unpaired t test. (J–K) Kidney colonization by GFP-labeled α3-KD and NT control cells. (L) Quantification of kidney colonization as % total surface area occupied by tumor cells. P=0.0185, unpaired t test.
On Day 35, the mouse with the highest burden of NT control cells was sacrificed along with the mice harboring the α3-KD cells. This NT control mouse was compared to an α3-KD mouse with a similar total tumor burden. A BLI-assisted gross necropsy revealed widespread dissemination of α3-KD cells compared to NT cells (Fig. 1C). Therefore, we allowed the remainder of the mice harboring NT cells to continue for another 11 days, until their mean BLI signal approached that of the α3-KD cell mice on Day 35 (Fig. 1B, annotation b). We took this approach to account for the possibility that increased dissemination was simply a function of increased α3-KD tumor growth. Disseminated α3-KD and NT cells were imaged by GFP fluorescence in tissues harvested just after sacrifice on Day 35 and Day 46, respectively. Compared to the NT cells, the α3-KD cells showed increased lung metastasis lung (Fig. 1D–F), and increased liver and kidney colonization (Fig. 1G–L). The primary tumor burden of α3-KD tumors and NT tumors was not significantly different at the time of sacrifice (Fig. S2A). Nevertheless, we normalized the lung, liver, and kidney data to the primary tumor BLI just prior to sacrifice. Even after adjusting for primary tumor signal, α3-KD cells showed greater lung metastasis and liver colonization (Fig. S2B, C), and an increased trend of kidney colonization (Fig. S2D), suggesting that loss of α3 integrin promotes dissemination from the prostate in addition to more rapid primary tumor growth.
We identified multiple in vitro assays of tumor cell growth that recapitulated the increased growth of the α3-KD tumors in vivo. Culturing cells on 3D collagen, under detached conditions, or under serum starvation all uncovered dramatically increased growth of α3-KD cells (Fig. 2A–C and Fig. S3A, B). Importantly, growth of the cells under standard 2D culture conditions in 10% serum did not reveal any difference (Fig. 2D); thus, the growth advantage of α3-KD cells pertains to low anchorage and/or low growth factor conditions. Control experiments confirmed that α3-KD and NT cells have virtually identical luminescence (Fig. 2E), eliminating the trivial possibility that differences between α3-KD and NT control cells in vivo and in vitro are due to BLI intensities. Silencing α3 integrin in another prostate cancer cell line, DU-145, produced similar results (Fig. S3C, D).
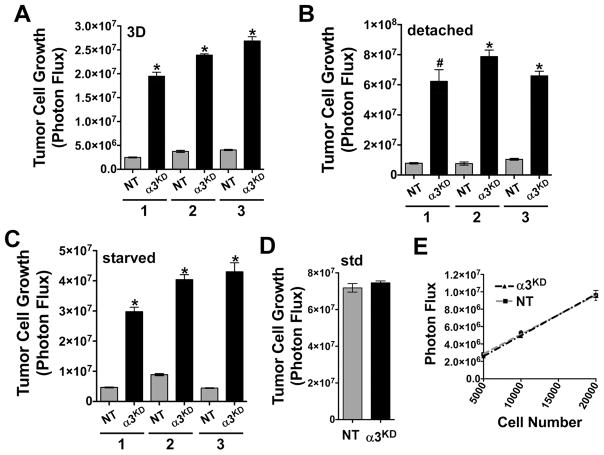
Tumor cell growth (A) on a 3D collagen matrix, (B) under detached conditions, floating over a poly-HEMA coated surface, and (C) on a 2D matrix under serum growth factor deprivation is shown for 3 independent trials of each condition, *P<0.0001, #P=0.0022, unpaired t test. (D) Tumor cell growth under standard tissue culture conditions in 10% fetal bovine serum. (E) Both α3-KD and NT control cells have a similar photon flux/cell and are not significantly different, P=0.5512, linear regression analysis. Error bars, ± SEM, n=6 wells per cell type/number of cells.
The increased growth of α3-KD cells is linked to aberrant RhoA signaling
Genetic deletion of α3 integrin causes aberrant signaling through Rho GTPases in both mammary myoepithelial cells and neurons (18,19). Therefore, we investigated the kinetics of Rho activation in α3-KD and NT cells. In α3-KD cells, LPA stimulation triggered a prolonged activation of both RhoA and RhoC. In contrast, Rho activation was more transient in NT cells, returning to baseline by 30 min post-stimulation (Fig. 3A, B).
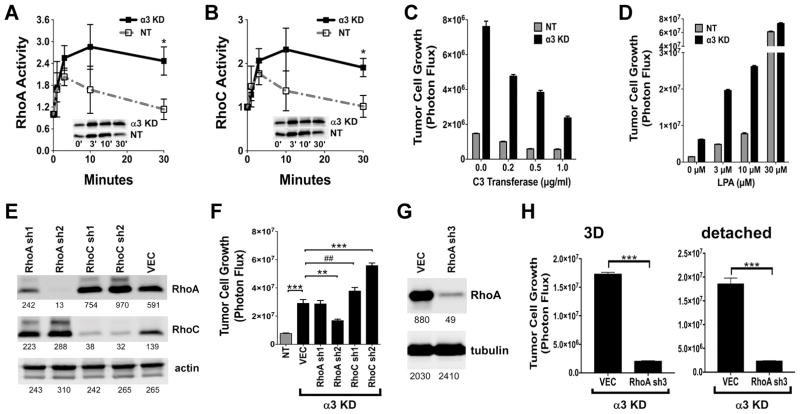
(A&B) RhoA and RhoC activation elicited by 5 μM LPA treatment was measured by GST-Rhotekin pulldown (*P=0.0355, unpaired t test, RhoA; *P=0.0492 unpaired t test, RhoC). Graphs show means ± SEM for 4 trials. Insets show representative blots of active RhoA and RhoC. (C) Treatment with C3-transferase drove down the 3D growth of the α3-KD cells in a dose-dependent manner. (D) LPA promoted the 3D growth of the α3-KD cells and NT control cells. (E) Stable silencing of RhoA and RhoC in the α3-KD cells. Numbers indicate intensity units measured with a LiCOR blot imager. (F) 3D growth assays comparing α3 integrin-expressing NT control cells to α3-KD cells harboring a non-targeting vector (VEC) or RhoA or RhoC shRNA constructs. Bars show mean ± SEM, n=6. Differences in growth were analyzed by ANOVA with Holm-Sidak’s multiple comparison test, ***P<0.0001, **P=0.003, ##P=0.0041, α=0.05. (G) Stable silencing of RhoA in α3-KD cells with a third shRNA; numbers indicate intensity units measured with a LiCOR blot imager. (H) Growth of α3-KD cells harboring a non-targeting vector (VEC) or the RhoA sh3 construct under 3D or detached conditions. Bars show mean ± SEM, n=6. Differences in growth were analyzed by unpaired t test, ***P<0.0001.
Since Rho signaling can promote anchorage-independent growth (20), we examined the effect on 3D growth of inhibiting Rho with C3 transferase or activating Rho with LPA. C3 transferase strongly suppressed the increased growth of the α3-KD cells (Fig. 3C), while LPA promoted 3D growth of both NT and α3-KD cells, and maximal Rho stimulation abolished the growth difference between the two cell types (Fig. 3D). This was consistent with α3 integrin functioning upstream to limit Rho-dependent 3D growth, with maximal Rho activation overriding α3’s suppressor effects.
We next used retroviral RNAi to silence RhoA or RhoC in α3-KD cells. RhoC was upregulated in the RhoA knockdown cells, while RhoA was upregulated in the RhoC knockdown cells, consistent with an earlier report (21) (Fig. 3E). Strikingly, depleting RhoA significantly blocked the 3D growth of α3-KD cells (Fig. 3F), but depleting RhoC actually increased 3D growth, to an extent that correlated with the extent of RhoA upregulation observed in the RhoC knockdown cells in Fig. 3E. An additional shRNA that produced strong RhoA knockdown also strongly suppressed the increased growth of α3-KD cells in 3D collagen and detached conditions (Fig. 3G, H).
Rho-driven anchorage-independent growth is linked to increased myosin-driven contractility (20). Indeed, we found that α3-KD cells displayed a hypercontractile phenotype upon serum stimulation (Fig. S4A, B), and the myosin inhibitor blebbistain dramatically reduced the growth of α3-KD cells under low growth factor or low anchorage conditions, with no effect on the low basal growth of NT control cells (Fig. S4C, D).
Increased expression and nuclear localization of Hippo pathway targets YAP and TAZ in α3 integrin-depleted cells
Several recent studies link Rho signaling and contractility to activation of proto-oncogenic transcriptional co-activators YAP1 and WWTR1/TAZ (11–13). In cells growing under detached conditions, YAP and TAZ were upregulated 2.5–3 fold in α3-KD cells compared to NT cells (Fig. 4A, B). Connective tissue growth factor (CTGF), a YAP/TAZ transcriptional target, was also upregulated in α3-KD cells (Fig 4C). Additionally, TAZ protein was upregulated in α3-KD DU-145 cells under detached conditions (Fig. S5), consistent with their increased 3D growth (Fig. S3D). In response to stimulation with 5 μM LPA, α3-KD cells displayed a higher nuclear to cytoplasmic (N:C) ratio of TAZ and YAP than did NT cells (Fig. 4D–F), consistent with the prolonged activation of RhoA in α3-KD cells treated with this dose of LPA (Fig. 3A).
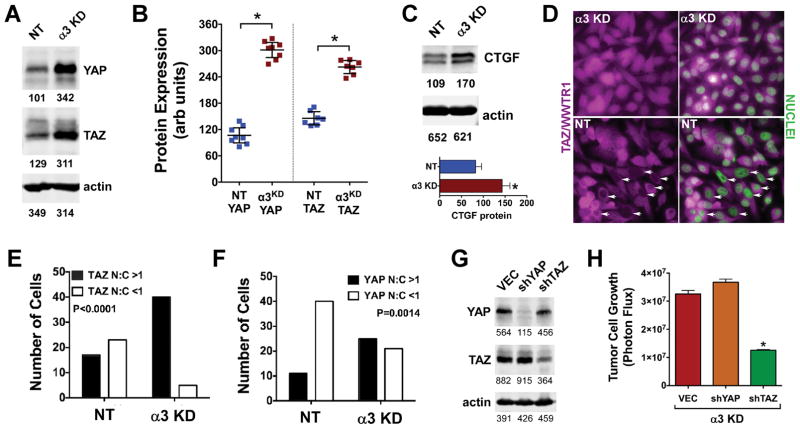
(A) Immunoblot of YAP and TAZ in NT control and α3-KD cells growing under detached conditions. (B) Multiple independent experiments showed increased YAP and TAZ in α3-KD cells versus NT control cells. *P<0.0001, unpaired t test. (C) Lysates of NT control and α3-KD cells growing under detached conditions were immunoblotted for CTGF. The graph shows mean CTGF levels in 3 trials after normalization to actin. (D) Serum-starved α3-KD or NT control cells were treated with 5 μM LPA for 20 h then fixed and stained for TAZ (magenta), with nuclei counterstained with DAPI (green). Arrows indicate NT control cell nuclei with reduced or absent TAZ staining compared to adjacent cytoplasm. An identical experiment was performed for YAP (not shown). (E & F) The proportions of cells with a nuclear:cytoplasmic (N:C) ratio of TAZ or YAP greater than or less than 1.0 were determined using Image J. A larger number of 7agr;3-KD cells displayed a nuclear:cytoplasmic ratio of TAZ and YAP>1 (P<0.0001 or 0.0014 respectively, Fisher’s exact test, n ≥ 40 cells). (G) Stable knockdown of YAP and TAZ in α3-KD cells. (H) Knockdown of TAZ but not YAP inhibited growth of the α3-KD cells on 3D collagen (P<0.0001, ANOVA with Tukey’s post-test; bars show mean ± SEM, n=6). Numbers below immunoblots show intensity units measured with a LiCOR blot imager.
To examine the link between Rho signaling, YAP, and TAZ under conditions where α3-KD cells show increased growth compared to NT cells, we performed Rho pulldown assays on 3 biological replicates of cells growing under detached conditions. α3-KD cells showed increased RhoA activity compared to NT cells under these conditions (Fig. S6A, B, D), but not increased RhoC activity (Fig. S6A, C, E), consistent with the specific role of RhoA observed in Fig. 3. Moreover, YAP and TAZ were again upregulated in α3-KD cells compared to NT cells (Fig. S6A, H, I), further confirming results in Fig. 4A–B.
We used retroviral RNAi constructs to generate stable YAP or TAZ knock down in α3-KD cells (Fig. 4G, Fig. S7A, C). Interestingly, silencing TAZ, but not YAP, strongly suppressed the 3D growth of the cells (Fig. 4H, Fig. S7B, D). YAP and TAZ are the targets of negative regulation by the Hippo pathway kinases, LATS1/2, but can also be activated by Rho signaling through LATS-independent mechanisms (11–13). Therefore, we silenced LATS1 in GS689.Li cells (Fig. S8A). LATS1-silenced cells displayed a 7-fold increase in 3D growth compared to vector control or cells with inactive shRNAs (Fig. S8B), phenocopying α3-KD cells. Conversely, force-expressing LATS1 in α3-KD cells partially reduced 3D growth (Fig. S8C, D). An additional LATS1 shRNA produced moderate LATS1 knockdown and increased 3D growth compared to vector control or an inactive LATS1 shRNA (Fig. S8E, F). These data are consistent with a role for LATS1 in α3-mediated growth suppression, but do not rule out LATS-independent mechanisms.
The invasive growth of α3 integrin-depleted cells is linked to impaired Abl kinase signaling
In neurons, α3β1 integrin activates the Arg/Abl2 tyrosine kinase, which in turn phosphorylates and activates a p190RhoGAP/p120RasGAP complex that inhibits Rho signaling (19). To investigate whether this pathway may be disrupted in our α3-KD cells growing in 3D collagen, we immunoprecipitated p190RhoGAP and blotted for (i) phosphotyrosine to detect activated p190, (ii) p120RasGAP to detect a p190/p120 complex, another readout for activated p190 (19), or (iii) total p190. Phospho-p190RhoGAP and p190-associated p120RasGAP were both reduced in α3-KD cells growing in 3D (Fig. 5A), consistent with the increased Rho activation in α3-KD cells. A similar result was obtained using cells growing in suspension (Fig. S9A) and with an antibody that recognizes Abl family kinase phosphorylation sites on Crk and CrkL (Y221/Y207), another readout of Abl kinase activity (22) (Fig. S9B).
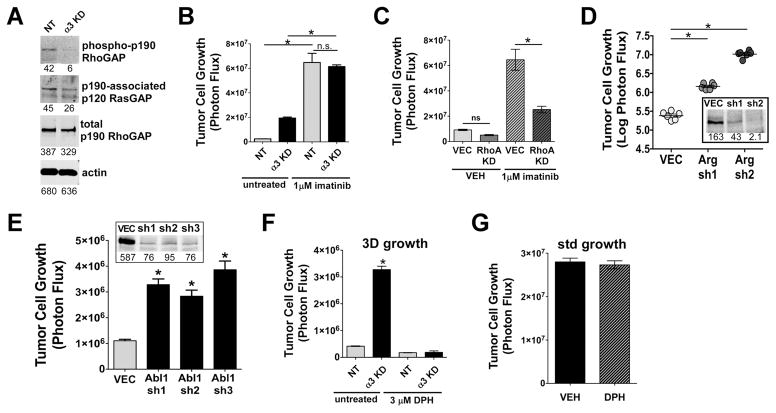
(A) The Arg/Abl2 substrate, p190RhoGAP, was immunoprecipitated from lysates of cells growing on 3D collagen in the presence of 5 μM LPA. p190RhoGAP IPs were blotted for phosphotyrosine, p120RasGAP, or total p190RhoGAP. (B) Imatinib increased the growth of NT control cells by ~25 fold, and the growth of α3-KD cells by ~3 fold, *P<0.0001, ANOVA with Tukey’s post-test. (C) A RhoA knockdown but not a non-targeting control vector (VEC) blocked the increased 3D growth of NT control cells caused by imatinib, *P<0.0001, ANOVA with Tukey post test. (D) Knockdown of Arg/Abl2 dramatically increased 3D growth of GS689.Li cells, *P<0.0001, ANOVA with Tukey’s post test. Inset: immunoblotting of Arg in cells harboring a non-targeting control vector (VEC) or Arg sh1 or sh2 constructs. (E) Knockdown of Abl1 significantly increased 3D growth of GS689.Li cells, *P<0.0001, ANOVA with Tukey’s post test. Inset: immunoblotting of Abl in cells with non-targeting control vector (VEC) or the 3 shRNA constructs. (F) Abl kinase activator, DPH, abolished the increased 3D growth of the α3-KD cells. *P<0.0001, ANOVA with Tukey’s post test. (G) DPH had no effect on α3-KD tumor cell growth under standard tissue culture conditions.
If an α3 integrin-Abl kinase pathway signals to suppress RhoA in prostate carcinoma cells, Abl kinase inhibitors might have paradoxical growth-promoting effects. Indeed, imatinib potently increased the 3D growth of NT cells by ~25-fold (Fig. 5B). For α3-KD cells, in which Abl kinase signaling may already be partially compromised (contributing to their ~8-fold growth advantage over NT cells), imatinib only increased growth an additional ~3-fold (Fig. 5B). RhoA RNAi revealed that imatinib’s pro-growth effects depend on the presence of RhoA (Fig. 5C).
We next depleted Arg by RNAi. Silencing Arg by 75% (sh1 construct) or 99% (sh2 construct) dramatically increased the 3D growth of the cells, with stronger silencing leading to a greater growth increase (Fig. 5D). Silencing Abl1 kinase also increased 3D growth, indicating that Abl1 and Arg cooperate to limit 3D growth (Fig. 5E). To investigate the effects of stimulating Abl kinase activity, we treated cells with the Abl kinase activator, DPH (23). A dose of 3 μM, near the reported cellular EC50 (23), abolished the growth of α3-KD cells in 3D (Fig. 5F), but had no effect on their growth under standard culture conditions (Fig. 5G).
The above experiments provided mechanistic insight into the increased growth of α3-depleted cells, but our in vivo assays suggested that more rapid growth of α3-KD tumors was not sufficient to explain their increased dissemination. Using time-lapse microscopy, we found that α3-KD cells migrate significantly faster than NT cells (Fig. 6A, B); α3-KD cells also showed increased invasion towards LPA in a transwell assay (Fig. 6C, D). Imatinib increased the mean migration speed of NT cells up to that of α3-KD cells (Fig. 6E). Conversely, DPH potently suppressed cell motility (Fig. 6F). In aggregate, our data support a model in which α3 integrin signals through Abl kinases to activate p190RhoGAP, suppress RhoA activity, and support Hippo pathway function. Upon loss of α3 integrin, this pathway is disrupted, resulting in increased RhoA activation, and increased TAZ/YAP activity driving invasive growth (Fig. 6G). TAZ/YAP activation by RhoA may involve both LATS1-dependent and –independent mechanisms.

(A) Cell motility tracks for NT control and α3-KD cells migrating on collagen I monitored by time-lapse microscopy, n= 20 tracks for each cell type. (B) Migration speed for NT control and α3-KD cells on collagen I for 6 independent trials; n ≥ 50 cells of each type per experiment; P=0.0056, unpaired t test. (C) High powered fields of migrated cells in a transwell invasion assay with NT control and α3-KD cells migrating towards 5 μM LPA in the lower chamber. (D) Quantification of invasion assay showing mean ± SEM of invaded cells, n=4 fields in each of 3 wells/cell type, P<0.0001 unpaired t test. (E) Time-lapse microscopy of NT control cells and α3-KD cells before and after 5 μM imatinib addition, P<0.0001, paired t test. (F) Time-lapse microscopy of NT control and α3-KD cells before and after addition of 3 μM DPH, P<0.0001, paired t test. (G) Working model. Suppressors of invasive growth are shown in blue and promoters in red.
A parallel mechanism for the increased 3D growth of α3-KD cells could involve altered signaling through FAK, a major integrin effector. Indeed, FAK activation was increased in α3-KD cells compared to NT cells growing under detached conditions (Fig. S10A). However, the selective FAK inhibitor PF-573228 failed to block 3D growth of either α3-KD or NT cells (Fig. S10B). Therefore, we currently favor an α3-Abl kinase-Hippo pathway signaling axis as the explanation for the increased growth of α3-depleted prostate carcinoma cells.
Loss of α3 integrin and increased nuclear TAZ and YAP in human prostate carcinoma
Our data suggested that loss of α3 integrin expression and altered TAZ and YAP activity may co-occur in prostate cancer. Therefore, we examined α3 integrin, TAZ, and YAP expression on adjacent sections of a human prostate cancer tissue microarray. We observed frequent downregulation of α3 integrin in prostate cancer compared to the strong basolateral staining found in benign glands (Fig. 7A, B). TAZ and YAP nuclear staining was observed in the proliferative basal cell layer of benign prostate glands, as reported (24), but TAZ was mainly absent from luminal cells of benign glands, and YAP localization was cytoplasmic rather than nuclear in luminal cells (Fig 7C–F). In contrast, TAZ and YAP nuclear staining were prominent in prostate carcinoma (Fig. 7C–F). We quantified staining using a quartile scoring system (Fig. S11; see Materials and Methods). Each core was assigned a primary and secondary score, which were summed, similar to the Gleason scoring system. These analyses confirmed that α3 integrin was significantly downregulated and nuclear TAZ and YAP were significantly upregulated in cancerous glands, compared to differentiated luminal cells of benign prostate epithelia (Fig. 7G).
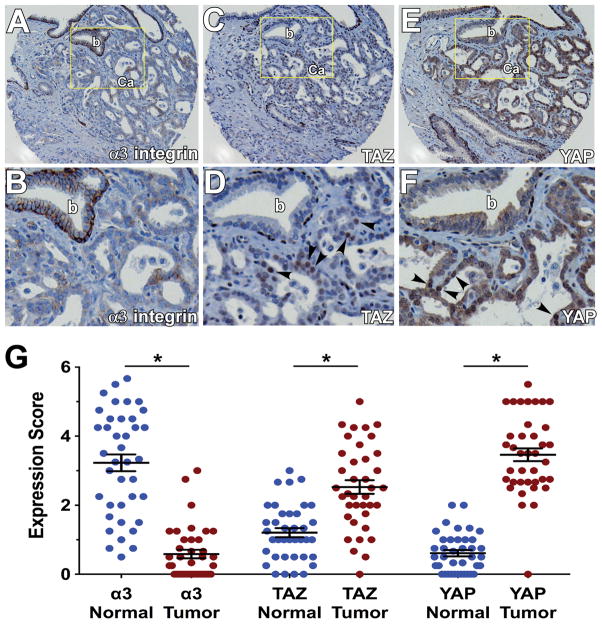
Immunohistochemical staining of α3 integrin (A&B), TAZ (C&D), and YAP (E&F) on adjacent sections of a prostate cancer tissue microarray. B, D, and F show enlarged views of the indicated fields in A, C, and E (b, benign gland; Ca, prostate carcinoma). Arrowheads indicate nuclear TAZ and YAP staining in prostate carcinoma. (G) Mean staining scores for α3 integrin, TAZ, and YAP on a 40 case microarray of human prostate cancer and matched normal tissues. Each case was represented by 4 cores of cancer and 4 cores of normal tissue. Each point represents the average score for each patient sample, across the 4 cores. The expression of α3 integrin was reduced, while nuclear expression of TAZ and YAP was increased in tumor versus normal glands *P<0.0001, paired t test. For scoring TAZ and YAP in normal glands, only luminal cells were considered.
Discussion
While evidence of Hippo pathway disruptions in prostate cancer continues to mount (25–27), little is known about the cell surface inputs that regulate the pathway. We now provide evidence that a cell surface receptor, α3β1 integrin, signals through Abl kinases to restrain Rho activity, support Hippo pathway function, and inhibit metastasis. Our study contributes to an emerging theme of α3β1 integrin as a moderator of Rho activity. Depleting α3 integrin activates RhoA in breast cancer cells (28) and loss of the α3 partner, tetraspanin CD151, drives Rho-dependent collective invasion (29). Conditional deletion of α3 integrin in mice deregulated Rho activity, disrupting the contractility-relaxation cycle of mammary myoepithelial cells (18) and the morphology of hippocampal neurons (19). Interestingly, we found that the increased 3D growth of α3-KD cells depends specifically on RhoA rather than RhoC. The basis for this specificity requires further investigation, but a potential clue comes from ovarian cancer cells, where RhoA but not RhoC signaled to promote YAP activation (30). RhoC might still contribute to the enhanced migration, invasion, and metastasis of α3 integrin-deficient prostate cancer cells, since RhoC can promote breast cancer or melanoma metastasis without influencing primary tumor growth (31,32).
Deregulated Abl kinase activity can drive malignant progression (33–35), but Abl kinase signaling can also suppress tumor growth in some contexts (33,36). In MDA-MB-231 breast cancer xenografts, the Arg knockdown dramatically potentiated primary tumor growth and lung metastatic colonization after tail vein inoculation, but suppressed spontaneous metastasis from the mammary fat pad (33). However, loss of Arg was accompanied by downregulation of Rho GTPase expression (33), which we did not observe in our α3-KD cells, and which thus may help to explain context-specific differences between the breast and prostate cancer models.
Context-specific, pro- and anti-tumor functions have also been reported for α3 integrin (8,10,37–40). Factors that influence whether α3β1 promotes or restrains tumor progression may include (i) whether the tumor secretes an α3β1 integrin ligand (38), (ii) the stage at which tumor progression is being modeled (39), (iii) the role of α3 integrin in controlling mRNA splicing (41), and (iv) the downstream signaling pathways driving malignant progression. For example, α3β1 promotes Rac activation (42), even as it limits Rho activity, and is required for Rac-driven mammary tumors (40), even as it restrains Rho-driven prostate carcinomas, as we show here.
In contrast to our results, imatinib in combination with paclitaxel was reported to inhibit the growth of a PC-3 prostate cancer sub-line in an intratibial model of tumor growth (43). In this pre-clinical model, imatinib was intended to target the PDGF receptor on tumor-associated endothelial cells and bone-adjacent tumor cells. Imatinib treatment inhibited proliferation and increased apoptosis of tumor cells growing in bone, but not cells invading into adjacent tissue (43). Thus, the effect of imatinib on prostate cancer growth in vivo is context-dependent, and the potential for endogenous Abl kinases to suppress the growth of PDGFR-independent tumor cells might help to explain the failure of the Abl kinase inhibitor imatinib in prostate cancer clinical trials, where imatinib treatment was associated with lower probability of PSA decline, and shorter progression-free and overall survival (44).
There is growing recognition that Hippo pathway disruptions promote not only tumorigenesis, but also progression and metastasis (27,45–47). Intriguingly, TAZ but not YAP was required for the increased 3D growth of α3-KD cells, reminiscent of the dominant role of TAZ in promoting expansion of human embryonic stem cells (48). Nuclear localized YAP promotes tumor growth, but cytoplasmic YAP can suppress proliferation (49), which might help to explain why we observed no net effect on tumor cell growth upon YAP depletion. However, YAP might still contribute to increased invasion and metastasis (45). Therefore, further studies are required to determine the relative contributions of TAZ and YAP to different stages of prostate cancer progression.
The working hypothesis supported by our data provides several clear predictions and potential therapeutic opportunities based on the context of α3 integrin expression. First, while Abl kinases can function as oncogenes in some contexts, our data predict that they could function as tumor suppressors in others, such as α3 integrin-deficient prostate cancers. If substantiated, this could lead to novel strategies involving the use of small molecule activators of Abl kinases (23) in carefully selected circumstances, and better informed use of multi-tyrosine kinase inhibitors that cross-inhibit Abl. A second prediction of our hypothesis is that, when present, the anti-tumor activity of Abl kinases stems from signaling to suppress Rho activity and thereby inhibit TAZ/YAP. This would provide a key mechanistic insight to explain how Abl inhibitors such as imatinib can sometimes paradoxically fuel cancer proliferation. Lastly, our model predicts that the increased progression and metastasis of α3-depleted prostate cancer cells requires TAZ (and potentially YAP), advancing α3-deficient prostate cancers as potential candidates for treatment with Hippo-directed therapies under pre-clinical development (13).
Acknowledgments
This work was supported by NIH R01 grants CA13664 to C.S. Stipp and CA130916 to M.D. Henry, and the Department of Defense Prostate Cancer Research Program Award #W81XWH-14-2-0182, W81XWH-14-2-0183, W81XWH-14-2-0185, W81XWH-14-2-0186, and W81XWH-15-2-0062 to the Prostate Cancer Biorepository Network (PCBN).
Core facilities used in these studies were supported by NIH grant P30 CA086862. Dr. Brown is the current University of Iowa Andersen-Hebbeln Professor in Prostate Cancer Research. This endowed professorship provided financial support related to this research effort.
Footnotes
Conflict of interest statement: The authors have no potential conflicts of interest to disclose
References
Full text links
Read article at publisher's site: https://doi.org/10.1158/0008-5472.can-16-1483
Read article for free, from open access legal sources, via Unpaywall:
https://cancerres.aacrjournals.org/content/canres/76/22/6577.full.pdf
Citations & impact
Impact metrics
Article citations
Roles for epithelial integrin α3β1 in regulation of the microenvironment during normal and pathological tissue remodeling.
Am J Physiol Cell Physiol, 326(5):C1308-C1319, 18 Mar 2024
Cited by: 1 article | PMID: 38497112
Review
Role of cell rearrangement and related signaling pathways in the dynamic process of tip cell selection.
Cell Commun Signal, 22(1):24, 09 Jan 2024
Cited by: 0 articles | PMID: 38195565 | PMCID: PMC10777628
Review Free full text in Europe PMC
Integrins Can Act as Suppressors of Ras-Mediated Oncogenesis in the Drosophila Wing Disc Epithelium.
Cancers (Basel), 15(22):5432, 15 Nov 2023
Cited by: 0 articles | PMID: 38001693
Crosstalk between Endothelial Cells and Tumor Cells: A New Era in Prostate Cancer Progression.
Int J Mol Sci, 24(23):16893, 29 Nov 2023
Cited by: 1 article | PMID: 38069225 | PMCID: PMC10707594
Review Free full text in Europe PMC
Abl kinases can function as suppressors of tumor progression and metastasis.
Front Oncol, 13:1241056, 08 Sep 2023
Cited by: 1 article | PMID: 37746268 | PMCID: PMC10514900
Go to all (37) article citations
Data
Data behind the article
This data has been text mined from the article, or deposited into data resources.
BioStudies: supplemental material and supporting data
HPA - The Human Protein Atlas
- (2 citations) HPA - HPA008572
Similar Articles
To arrive at the top five similar articles we use a word-weighted algorithm to compare words from the Title and Abstract of each citation.
Integrin α3β1 regulates tumor cell responses to stromal cells and can function to suppress prostate cancer metastatic colonization.
Clin Exp Metastasis, 30(4):541-552, 06 Dec 2012
Cited by: 25 articles | PMID: 23224938 | PMCID: PMC3604149
The metastasis suppressor CD82/KAI1 inhibits fibronectin adhesion-induced epithelial-to-mesenchymal transition in prostate cancer cells by repressing the associated integrin signaling.
Oncotarget, 8(1):1641-1654, 01 Jan 2017
Cited by: 26 articles | PMID: 27926483 | PMCID: PMC5352085
Receptor tyrosine kinase Met promotes cell survival via kinase-independent maintenance of integrin α3β1.
Mol Biol Cell, 27(15):2493-2504, 15 Jun 2016
Cited by: 10 articles | PMID: 27307589 | PMCID: PMC4966988
Integrin α3β1 as a breast cancer target.
Expert Opin Ther Targets, 15(10):1197-1210, 13 Aug 2011
Cited by: 42 articles | PMID: 21838596 | PMCID: PMC3212412
Review Free full text in Europe PMC
Funding
Funders who supported this work.
NCI NIH HHS (3)
Grant ID: P30 CA086862
Grant ID: R01 CA136664
Grant ID: R01 CA130916
NIH (3)
Grant ID: CA130916
Grant ID: P30CA086862
Grant ID: CA13664
Prostate Cancer Biorepository Network (1)
Grant ID: #W81XWH-14-2-0182, W81XWH-14-2-0183, W81XWH-14-2-0185, W81XWH-14-2-0186, and W81XWH-15-2-0062