Abstract
Free full text

New findings on venous thrombogenesis
Summary
Venous thrombosis (VT) is the third most common cause of cardiovascular death worldwide. Complications from VT and pulmonary embolism are the leading cause of lost disability-adjusted life years. Risks include genetic (e.g., non-O blood group, activated protein C resistance, hyperprothrombinemia) and acquired (e.g., age, surgery, cancer, pregnancy, immobilisation, female hormone use) factors. Pathophysiologic mechanisms that promote VT are incompletely understood, but involve abnormalities in blood coagulability, vessel function, and flow (so-called Virchow’s Triad). Epidemiologic studies of humans, animal models, and biochemical and biophysical investigations have revealed contributions from extrinsic, intrinsic, and common pathways of coagulation, endothelial cells, leukocytes, red blood cells, platelets, cell-derived microvesicles, stasis-induced changes in vascular cells, and blood rheology. Knowledge of these mechanisms may yield new therapeutic targets. Characterisation of mechanisms that mediate VT formation and stability, particularly in aging, are needed to advance understanding of VT.
Venous thrombosis (VT) is a complex pathology defined as the formation of intra-vascular blood clots in the venous circulation that can migrate to the lungs (pulmonary embolism, collectively venous thromboembolism) (1). VT is the third most common cause of cardiovascular death worldwide, accounting for over half a million deaths each year in both the United States and European Union (1, 2). Mortality is particularly high in the first 30 days after VT due to development of fatal pulmonary embolism. One-year mortality following VT is 10 %, and there is a significant risk of VT recurrence in survivors.
VT risk rises with age, particularly after age 55 (3). VT risk also increases with
surgery,
pregnancy,
cancer,
prolonged immobilisation such as long-haul travel or hospitalisation,
female hormone use (hormone replacement therapy or oral contraceptives), and
recent myocardial infarction.
Genetic risk factors for VT include
non-O-blood group,
factor V mutations that increase resistance to activated protein C (most commonly factor V Leiden),
prothrombin G20210A mutation, and
congenital deficiencies in proteins C, S, or antithrombin.
Half of patients with chronic VT will develop the post-thrombotic syndrome, characterised by pain, edema, and ulcers.
The frequency of VT in the general population and the identification of risk factors associated with VT have helped drive research on VT pathogenesis for over a century. The seminal work of German physician Rudolf Virchow led to the identification of three broad risk factors for the development of VT:
blood hypercoagulability,
endothelial dysfunction, and
altered blood flow.
Even 100 years after the first description of this so-called Virchow’s Triad, pathophysiologic mechanisms thought to drive VT can be grouped into these three basic categories (Fig. 1). Herein, we review the current state of VT pathogenesis research and place recent findings within in the framework of Virchow’s triad.
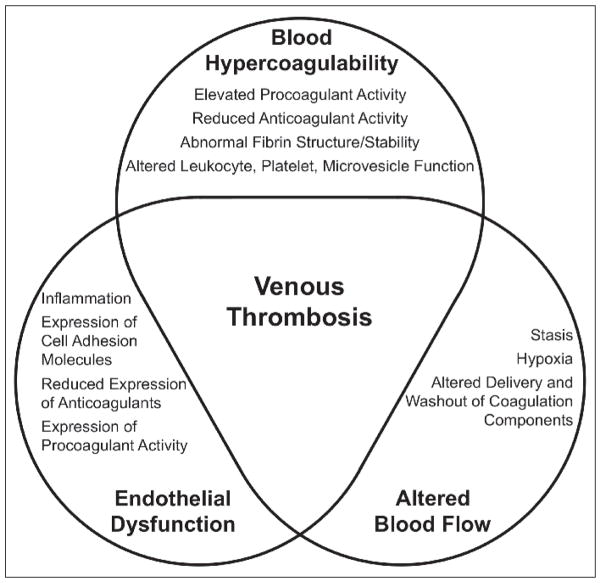
Virchow’s Triad and venous thrombosis (VT). Risk factors for VT are broadly grouped into the three tenets of Virchow’s Triad: blood hypercoagulability, endothelial dysfunction, and altered blood flow.
We first provide a brief summary of animal models of VT, and then describe current views on the roles of blood hypercoagulability, endothelial dysfunction, and altered blood flow in venous thrombogenesis. We also discuss newly-recognised contributors to VT. Throughout, we will connect basic research discoveries with clinical observations to provide a broad overview of the pathogenesis of VT.
Animal Models of VT
The most frequent animals used to model VT are rodents, the most common of which is the mouse due to their small size and ease of genetic manipulation (4). The most common rodent VT models can be segregated into three main groups according to their triggering mechanism:
inferior vena cava (IVC) stasis or stenosis,
ferric chloride application, and
electrolytic stimulation.
The IVC stasis and stenosis models both involve placement of a suture around the IVC just below the renal branches. In the stasis model, ligation is complete, whereas, in the stenosis model, ligation is incomplete, maintaining some blood flow. The IVC stenosis model exhibits considerable variability in thrombus development, but is considered valuable for therapeutic studies as flow may permit transport of therapeutics to the growing thrombus. A brief crushing of the IVC is occasionally used to increase thrombus frequency and reproducibility (5). In both IVC models, thrombi are typically analysed 6–24 hours after thrombus induction.
The ferric chloride model involves exposing the vein wall to ferric chloride that induces vessel damage, resulting in thrombus formation. In the electrolytic model, direct current is applied to the vein wall; this induces free radical formation, and results in vessel damage, endothelial activation, and thrombus formation. Although the ferric chloride and electrolytic models reliably produce thrombi that are amenable to real-time monitoring via intravital microscopy, these thrombi develop rapidly (less than 1 hour) and the relevance of this time course to human VT is unclear.
More recently, a model of spontaneous thrombosis has been described. This model uses siRNA to knockdown antithrombin and protein C, which leads to diffuse, spontaneous venous thrombus formation within two days of knockdown (6). This VT model does not require surgical manipulation and may better recapitulate the human condition than the more commonly-used IVC stenosis or stasis models. However, the spontaneous VT model is accompanied by severe coagulopathy, so the operant pathogenic mechanisms remain unclear.
Nonhuman primates have also been used to model VT (7). Although this model may best recapitulate aspects of VT in humans, it is less frequently used due to size, expense, and ethical considerations.
Mechanisms of VT
Venous thrombi are described as „red clots“ because of their high red blood cell (RBC) content. These thrombi are also enriched in fibrin, and contain platelets and leukocytes. Given the numerous, diverse risk factors associated with VT, it is not surprising that pathophysiologic mechanisms attributed to VT implicate multiple aspects of the blood and vascular system. These pathways are broadly characterised in three major areas:
blood hypercoagulability,
endothelial dysfunction,
and altered blood flow (stasis).
Notably, penetrance is generally low, giving rise to the premise that simultaneous dys-regulation in more than one of these areas precipitates VT. Consequently, identifying the operant mechanisms has been challenging.
Blood hypercoagulability
Initiation of coagulation
Coagulation can be triggered by either the extrinsic (via the tissue factor/factor VIIa complex) or intrinsic/contact (via factor XIIa) pathways. Interestingly, studies using murine models of thrombosis have implicated both pathways in VT pathogenesis; however, findings from these studies are discordant.
Several studies have implicated tissue factor in thrombus initiation; however, these studies have not yet resolved the source of cellular tissue factor. Using the IVC stasis model of VT, Day et al. (8) implicated vessel wall-derived tissue factor in VT initiation, but observed no role for leukocyte tissue factor. In contrast, using the IVC stenosis model, von Brühl et al. (9) found that leukocyte-derived tissue factor was necessary for thrombus formation. The discord between these observations may reflect differences in the thrombosis models employed for these studies.
Other studies have implicated the contact pathway activator factor XII(a) in VT initiation. In experiments using the IVC stenosis model, von Brühl et al. (9) found that VT mass is reduced in mice genetically-deficient in factor XII. However, a recent study using the antithrombin/protein C-knockdown thrombosis model questioned the role of factor XII in this process (10). Unexpectedly, that study showed increased severity of thrombosis in antithrombin/protein C/factor XII-depleted mice relative to controls, although the mechanism for this increased pathology is unknown. Here again, discord between these studies may stem from mechanistic differences in the VT models used.
Given the discord in these findings, the primary „initiating pathway“ in VT remains unclear.
Clarifying the activity that initiates VT is important, because it may expose a therapeutic target that would prevent even early intravascular thrombus formation. In particular, since factor XII-deficient patients do not have a bleeding risk, therapeutically targeting this factor may prevent thrombosis with a minimal risk of bleeding.
Downstream coagulation factors
Downstream of these initiating events, clinical studies have shown that plasma hypercoagulability can result from elevated levels and/or activity of coagulation factors (11–15) or from deficiencies in the levels of functions of anticoagulant proteins (16, 17). These „hypercoagulable states“ ultimately increase thrombin generation, and are observed in patients with both primary and recurrent VT (18–21).
One example of an established hypercoagulable state that results in increased thrombin generation is found in individuals with the G20210A prothrombin mutation. Individuals with this mutation exhibit hyperprothrombinaemia and have increased risk of VT (15). Patients with the G20210A mutation have mildly elevated markers of coagulation activation at baseline, and markedly increased thrombin generation following activation of coagulation (22, 23).
In agreement with clinical observations, basic studies using mathematical models and in vitro systems have also demonstrated that elevated levels or activity of factors VIII, IX, XI or prothrombin increase plasma thrombin generation (24–28). Other in vitro studies have extended these observations to show that elevated thrombin generation results in the formation of dense fibrin networks (27), which is associated with increased thrombosis risk (29).
The role(s) of several coagulation factors in VT have been tested in mouse models. Following ferric chloride injury to the IVC, mice deficient in factor XI show reduced thrombus weight relative to controls, suggesting factor XI contributes to VT (30). Interestingly, factor XI-deficient mice do not have a bleeding phenotype in a tail-bleeding model (30), suggesting factor XI inhibition may prevent thrombosis without increasing bleeding. Indeed, factor XI antagonism has been tested in pre-clinical and clinical studies; as anticipated from animal models, these suggest FXI antagonism reduces the incidence of VT without substantively increasing bleeding (31). Notably, however, a small percentage of human patients with factor XI deficiency exhibit tissue-specific bleeding following trauma, suggesting FXI inhibition could increase bleeding risk in certain individuals. Further studies to define the contributions of factor XI to thrombosis and haemostasis and identify individuals who may be at risk for bleeding are important for the continued development of this therapeutic approach.
Mouse models have also been used to test the roles of established hypercoagulabilities, including elevated factors VIII and prothrombin, and presence of factor V Leiden in VT. Mice with elevated factor VIII have shortened time to saphenous vein occlusion following ferric chloride injury (28). Mice infused with prothrombin to mimic the hyperprothrombinemia found in patients with the G20210A prothrombin mutation show enhanced plasma thrombin generation and increased thrombus size in the IVC stasis model, and faster fibrin deposition in the electrolytic saphenous vein injury model of VT (32). Similarly, following electrolytic injury, genetically-engineered mice bearing the clinically-relevant prothrombotic factor V Leiden mutation form larger femoral vein thrombi relative to wild-type controls (33). Finally, the effect of anticoagulant deficiency is also demonstrated in the antithrombin/protein C-knockdown model of VT (6). The significant prothrombotic effect of these knockdowns is consistent with the potent prothrombotic phenotype observed in patients with protein C or antithrombin deficiency (16, 17).
Collectively, these studies not only support causative roles for these hypercoagulabilities previously associated with VT, but also demonstrate the utility of mouse models in studies of VT.
Fibrin(ogen) and factor XIII
During coagulation, soluble fibrinogen is cleaved by thrombin and then polymerises into fibers that structurally reinforce the thrombus. In epidemiologic studies, elevated levels of fibrinogen (hyperfibrinogenaemia) or presence of dysfunctional fibrinogen (dysfibrinogenemia) have been associated with increased risk of VT (11, 34, 35). We have shown that following ferric chloride injury, mice infused with fibrinogen to model hyperfibrinogenemia exhibit accelerated saphenous vein thrombosis; this effect is associated with increased thrombus fibrin content and resistance to thrombolysis (36).
In many prothrombotic disorders including diabetes, hyperhomocysteinaemia, and smoking-related thrombosis, fibrinogen is subject to aberrant post-translational modifications in circulation. These modifications can result in an „acquired dysfibrinogenemia“ that likely contributes to VT through alterations in fibrinogen cleavage, fibrin polymerisation, and fibrin network structure (37).
Elevated levels of fibrinolysis inhibitors in patients, as well as increased activation of fibrinolytic inhibitors (e.g., thrombin activatable fibrinolysis inhibitor) may also contribute to VT by decreasing endogenous fibrinolytic mechanisms, leading to decreased fibrin dissolution (38).
In circulation, some fibrinogen molecules are bound by zymogen factor XIII (FXIII). During fibrin formation, thrombin activates FXIII (to FXIIIa), which stabilises fibrin by introducing covalent crosslinks between the fibrin γ-chains to form γ-γ dimers, and later between fibrin α-chains to form high molecular weight crosslinked species. When the interaction between fibrinogen and zymogen FXIII is disrupted in either mouse (39) or human (40) proteins, fibrin crosslinking is delayed.
The contributions of FXIII(a)-mediated fibrin crosslinking to VT are poorly understood, and findings regarding the relationship between FXIII antigen/activity levels and VT risk have been discordant (41). The most widely studied FXIII polymorphism is Val34Leu, which results in accelerated FXIII activation. Overall, studies have suggested this polymorphism has a slight protective effect against VT, but this effect is dependent on complex gene-environment interactions (41).
Recently, we directly examined the role of FXIII activity in VT. Compared to wild-type mice, FXIII-deficient mice produce smaller thrombi in the IVC stasis model, and this reduction in thrombus size is secondary to decreased RBC retention. Furthermore, clots from FXIII-deficient patients also exhibit reduced RBC retention following clot contraction (39, 42). Follow-up studies showed that FXIIIa-dependent crosslinking of fibrin α-chains, and subsequent formation of high molecular weight crosslinked species, drives RBC retention in clots (43). Interestingly, crosslinked fibrin is a remarkable biopolymer with strength and extensibility superior to that of spider silk, and previous biophysical studies have shown that the mechanical stability of the fibrin network is primarily derived from α-chain crosslinking and high molecular weight crosslinked species formation (44–47).
Taken together, these data implicate fibrin network strength as an important mediator of RBC retention in clots and suggest that inhibiting FXIII(a) may reduce thrombus size.
Intriguingly, data from mice with reduced binding of zymogen FXIII to fibrinogen show reduced thrombus RBC content and size in both the IVC stasis and stenosis models (39). These data suggest that disrupting the FXIII-fibrin(ogen) interaction also may be a strategy for reducing VT. In the context of previous studies showing that RBCs reduce the permeability and fibrinolytic susceptibility of clots (48, 49), reducing RBC content in thrombi may not only reduce thrombus size, but also increase thrombus susceptibility to lysis. It remains to be seen whether these are viable strategies for reducing VT.
Circulating blood cells and cell-derived microvesicles
The role of circulating blood cells in VT has been a major focus of VT research. Leukocytes, RBCs, and platelets are all present in VT, and data suggest each contributes to VT pathogenesis.
Leukocytes
Leukocyte-mediated activation of coagulation is a key component of innate immunity, as clots trap invading pathogens and recruit immune cells to the site of infection. Unfortunately, the procoagulant functions of leukocytes can also manifest in a pathologic setting such as VT. The aberrant function of leukocytes in VT pathogenesis has been termed „immunothrombosis“ (50). Elevated leukocyte counts are associated with VT in cancer patients prior to chemotherapy (51, 52). Although leukocytes have long been considered important players in VT, potential mechanisms by which leukocytes promote thrombosis have only recently been identified.
Both mouse and rat models of VT have implicated leukocyte tissue factor in venous thrombogenesis. In the IVC stenosis model of VT in mice, neutrophils and monocytes are recruited early following thrombus initiation (53). Similarly, in a rat stasis model, Zhou et al. (54) observed rapid accumulation of tissue factor-positive leukocytes early in thrombogenesis, suggesting this leukocyte-derived tissue factor may drive VT. Consistent with this premise, mice expressing low levels of leukocyte tissue factor are protected from VT, indicating leukocyte tissue factor can initiate thrombosis, at least in the murine stenosis model of VT. Studies with P-selectin-deficient mice suggest leukocyte accumulation depends on expression of endothelial P-selectin (9).
Neutrophils are also thought to contribute to thrombosis by programmed release of DNA as neutrophil extracellular traps (NETs). NETs are networks of DNA, histones, and antimicrobial proteins that have been shown to interact with RBCs and platelets (55). NETs have been detected in thrombi from mice (9), as well as baboons (55) and humans (56). Depletion of neutrophils or treatment with DNase 1 to eliminate NETs reduces thrombus growth in mice (9). Interestingly, extracellular DNA and RNA have also been shown to activate factor XII, which may link leukocyte function to contact pathway-driven activation of coagulation and thrombosis (9, 57).
Furthermore, the proteases found in neutrophil releasates can cleave and inactivate tissue factor pathway inhibitor, which effectively increases local tissue factor activity (58). Interestingly, however, in mice lacking protein C and antithrombin due to siRNA treatment, neutrophil depletion does not reduce the development of spontaneous VT (10).
Red blood cells (RBCs)
Historically, RBCs have been considered passive bystanders in VT that simply become trapped in the growing thrombus. However, thrombus RBC content is a major determinant of venous thrombus mass. Using mice with deficient RBC retention in clots, we have shown that reducing thrombus RBC content significantly reduces thrombus size (39).
These data suggest that reducing thrombus RBC content may be a viable strategy for reducing VT.
Epidemiologic studies suggest RBCs may actively contribute to VT. For example, the Tromsø Study (59) showed that individuals with haematocrits in the upper quintile had a 1.5-fold increased risk of VT relative to individuals with haematocrits below the 40th percentile. RBCs have several properties that may mediate prothrombotic effects during VT:
RBCs are a significant mediator of blood viscosity. Elevated haematocrit promotes RBC aggregation in the low-shear environment of the venous circulation, which increases blood viscosity and may reduce blood flow (60).
Furthermore, in vitro studies have shown that RBCs and RBC-derived microvesicles can support thrombin generation, likely through the exposure of phosphatidylserine on their surface (61–63).
In flowing blood, RBC motion causes platelets to marginate to the vessel wall. The platelet concentration in this area can be enriched 3–5-fold (64), which may promote platelet interactions with the vessel wall during thrombus initiation.
Whether this relationship is causal remains unknown, and more studies investigating the role of haematocrit and platelet margination in VT are required. However, studies of elevated haematocrit in both humans and mice are difficult because of complicating comorbidities associated with altered haematocrit. Animal models of varying haematocrit in isolation from other pathologies are required to fully investigate the relationship between haematocrit and VT.
Beyond their potential function in thrombin generation, the sheer bulk of RBCs in venous thrombi may also play an important functional role in VT pathogenesis. RBCs reduce the permeability of fibrin clots (48), and consequently may retard fibrinolysis by preventing lytic factors from penetrating the clot (49). Scanning electron microscopy of contracted whole blood clots shows that RBCs within thrombi are compacted into tightly-packed polyhedra (65). These compacted RBCs reduce clot permeability in vitro (65) and possibly also in vivo.
Collectively, these data suggest the retention of RBCs within venous thrombi not only increases thrombus mass, but also may increase the resistance of thrombi to fibrinolysis.
Platelets
The contribution of platelets to VT is controversial. Elevated platelet counts are a risk factor for VT in patients with cancer, undergoing chemotherapy, or hospitalisation (66–68). In addition, two meta-analyses of the WARFASA and ASPIRE clinical trials suggested low-dose acetylsalicylic acid reduces the risk of recurrent VT (69, 70). However, a recent literature review did not find evidence for efficacy of platelet antagonism in treating VT or reducing primary VT incidence (71).
In contrast to the epidemiologic and clinical studies, investigations with mice generally support the notion that platelets contribute to VT. Mice with decreased platelet counts exhibited smaller thrombi and less frequent thrombosis in both the IVC stenosis and protein C/antithrombin-depletion models of VT, respectively (9, 10). In addition, von Willebrand factor (vWF)-deficient mice have reduced thrombus mass and this may result from reduced platelet recruitment to the developing thrombus (72). A recent study implicated the mechanistic target of rapamycin complex 1 (mTORC1)-mediated platelet hyper-activation in increasing thrombus size in aged mice (73). Platelets also mediate contraction of blood clots from humans and from mice; clot contraction consolidates thrombi and decreases thrombus porosity, which protects the clot by slowing delivery of fibrinolytic proteins to the thrombus core.
The apparent discord between the epidemiologic and clinical studies, and the in vitro and mouse studies may suggest that the platelet functions antagonised by acetylsalicylic acid are not prominent pathologic pathways during venous thrombogenesis. Further in vivo studies are required to define the platelet signaling and activation pathways that contribute to VT.
Cell-derived microvesicles
Microvesicles are sub-micron membrane vesicles shed from activated and apoptotic cells, including
leukocytes,
platelets,
endothelial cells, and
RBCs.
Early hypotheses speculated that micro-vesicles are pathologic because they can possess procoagulant properties of the parent cell without the normal regulatory mechanisms. However, in spite of many investigations, the contribution of micro-vesicles to VT is unclear. In vitro, micro-vesicles influence coagulation in a variety of ways, including promoting thrombin generation, accelerating fibrin formation, and increasing fibrin stability (74, 75). Some animal studies suggest microvesicles can promote VT. For example, in the murine IVC stasis model, infusion of leukocyte- or platelet-derived microvesicles increases thrombus weights (76).
However, there are little clinical data to support a causative role for elevated circulating microvesicles in the development of VT. The only clinical setting in which microvesicles have been implicated in VT is pancreatic cancer, where levels of tissue factor-positive, tumour-derived microparticles are associated with increased VT risk (77). This observation was supported by murine studies showing that injection of mice with exogenous tissue factor-positive microvesicles leads to increased thrombosis in both the IVC stenosis (78) and ferric chloride injury (79) VT models.
Endothelial dysfunction
Normal vascular endothelium expresses both antithrombotic and profibrinolytic factors and is crucial for maintaining the fluidity of circulating blood. One of the most important anticoagulant proteins expressed by endothelial cells is thrombomodulin. Interestingly, the concentration of thrombomodulin on the endothelium within valve pockets is higher than on endothelium adjacent to the valve pocket. The valvular endothelium also expresses higher levels of endothelial protein C receptor than adjacent endothelium (80). Increased expression of these anticoagulant proteins are thought to help reduce thrombus initiation in the highly-prone valve pocket that lacks the protection of laminar shear-mediated anticoagulant activity.
It is largely thought that venous thrombi form on intact, but dysfunctional, endothelium. Endothelial dysfunction is thought to arise from inflammation and/or abnormal blood flow, which triggers conversion of normally quiescent endothelial cells to a procoagulant phenotype. Inflammatory biomarkers associated with VT include interleukins-6 and -8, C-reactive protein, and tumour necrosis factor-α (81). Recent studies using the mouse IVC stasis model also identified galectin-3 and galectin-3 binding protein as potential biomarkers for VT (82).
Interestingly, some of these biomarkers have been shown to directly link inflammation and endothelial dysfunction. For example, inflammation can lead to increased circulating levels of cytokines (e.g., interleukin-1, tumour necrosis factor-α) that activate and alter endothelial protein expression (83, 84). These inflammation-induced changes convert the vessel wall to a prothrombotic surface. Consistent with this concept, tumour necrosis factor-α treatment promotes thrombin generation on cultured endothelial cells, and consequently, the formation of abnormally dense fibrin networks that resist fibrinolysis (84).
Hypertension can also increase pressure on the vessel wall which may alter endothelial tissue factor expression (85) and increase endothelial microvesicle formation (86).
Changes that promote cell adhesion, such as increased expression of intracellular adhesion molecule-1, E-selectin, and P-selectin also promote endothelial cell prothrombotic potential (87, 88). For example, stasis-induced translocation of Weibel-Palade bodies brings P-selectin to the endothelial cell surface. P-selectin recruits leukocytes to the endothelium via P-selectin glycoprotein ligand 1 (PSGL1) (9). Mouse and baboon models of thrombosis show that either PSGL1 or P-selectin antagonism prevents VT, supporting the premise that leukocyte adhesion to dysfunctional endothelium supports VT (89–91). Furthermore, Weibel-Palade bodies also release vWF, which promotes platelet and leukocyte adhesion. Studies using murine VT models have implicated vWF in VT development (72).
Altered blood flow
The role of altered blood flow on thrombosis is perhaps the most poorly understood component of Virchow’s Triad, but it is clear that abnormal blood flow is a significant contributor to VT. A study of stroke patients with hemiparesis showed the occurrence of VT in the immobilised leg (53 %) is significantly higher than in the functional leg (7 %) (92).
The valve pockets of large veins in the leg are particularly susceptible to changes in blood flow (turbulence or complete stasis), and are thought to be the originating site of most venous thrombi (93). Investigators examining oxygen distribution in vein valve pockets observed that stasis induces a declining oxygen concentration gradient from the top of the pocket to the bottom (94). Hypoxia induces the expression and release of inflammatory mediators from endothelial cells and concurrently reduces thrombomodulin expression (95). Collectively, these changes increase the thrombogenicity of the endothelial surface.
Blood flow in the vasculature is defined by two interdependent variables: shear stress and shear rate. Shear stress is typically used to describe the tangential force of blood flow on the vessel wall, whereas the shear rate is usually used to describe the rate at which blood is flowing past the vessel wall. Both shear stress and shear rate are important factors for regulating homeostasis in circulation, and abnormalities in either can promote VT.
In the presence of normal shear stress, the shear-sensitive Krüppel-like transcription factors (KLFs), namely KLF2 and KLF4, maintain endothelial homeostasis by regulating expression of anti- and pro-coagulant proteins on the endothelial surface. However, in situations in which blood flow is reduced (stasis), such as can occur during hospital-related immobilisations or long-haul travel, shear stress is reduced. Reduced flow or stasis downregulates the activity of the KLFs, and triggers activation of nuclear factor-κB (NF-κB) (96). Decreased KLF activity reduces thrombomodulin expression (97), whereas NF-κB activity promotes expression of cellular adhesion molecules (e.g., vascular cell adhesion protein 1, E-selectin), the antifibrinolytic plasminogen activator inhibitor-1, and possibly also tissue factor (54, 97–99). Combined with the downregulation of thrombomodulin, these changes render the endothelium prothrombotic.
Shear-sensitive KLFs are also expressed in circulating blood cells, including myeloid cells; however, the relationship between altered blood flow, KLF activity, and function in circulating blood cells is unclear. Interestingly, the JUPITER trial (100) showed that patients taking rosuvastatin are protected from VT. Statins increase KLF2 activity and downregulate NF-κB signaling (101), which may reduce stasis-mediated endothelial dysfunction and perhaps also monocytic tissue factor expression (102), and consequently, reduce thrombus development.
Like shear stress, shear rate also has a multifaceted role in VT. Shear rate controls the delivery of procoagulant reactants to the site of thrombosis as well as the diffusion of these reactants away from the thrombus. This balance of delivery of reactants to the growing thrombus and diffusion of products downstream are crucial determinants of the rate of thrombin generation, fibrin formation, and consequently thrombus growth (64). For example, influx and efflux of procoagulant molecules have a significant impact on fibrin network formation. Increasing shear rate from 10 s−1 to 100 s−1 reduces the local availability of fibrin monomers at the site of coagulation, leading to reduced protofibril formation and lateral aggregation (103).
Furthermore, the shear rate influences the orientation of forming fibrin fibers. In static conditions, fibers are randomly distributed, whereas even slight flow orients fibers in the direction of the shear and causes the formation of thick, bundled fibers (103, 104). These differences in fiber arrangement can dramatically affect the biochemical stability of the network during fibrinolysis; compared to thin fibers, thick, bundled fibers exhibit increased resistance to lysis (105, 106).
Collectively, these data suggest that blood flow itself is an important determinant of the ultimate fibrinolytic resistance of a venous thrombus.
Conclusions and future
In the century since Virchow described the roles of blood hypercoagulability, endothelial dysfunction, and altered blood flow in VT, basic understanding of mechanisms underlying each tenet of Virchow’s Triad has greatly improved. However, many questions remain unanswered:
First, there is some discord between animal models used for laboratory investigations, and between these models and aspects of human pathophysiology. Increased understanding of these models is essential to reconcile these differences and appropriately recapitulate venous disease in humans. Furthermore, most animal models are performed in young mice that do not recapitulate conditions under which age-related VT occurs in humans. Even studies that have examined VT in aged mice are limited by fundamental differences in the physiology of aged mice versus aged humans. For example, platelet count rises with age in mice, whereas it falls with age in humans. Future research aimed at understanding the operant mechanisms in age-related thrombosis will be valuable for developing new strategies to limit VT in the most affected demographic, the elderly.
Second, whereas some biomarkers for thrombosis (e.g. D-dimer) have been incorporated into clinical decision-making algorithms to identify ongoing VT in patients, the role of other thrombosis biomarkers (e.g. C-reactive protein, cell-free DNA) in identifying VT remains unclear.
Lastly, this review is limited in scope to mechanisms affecting the development and propagation of VT, and does not discuss mechanisms affecting thrombus resolution or the post-thrombotic syndrome. These are important components of VT pathology, and we direct the reader to excellent review articles focused on these topics (107, 108). Additional investigation of the pathophysiologic mechanisms determining the rate of thrombus resolution and severity of post-thrombotic syndrome will improve treatment of VT patients after the onset of thrombosis.
Factors contributing to blood hyper -coagulability are the primary targets of current antithrombotics, including warfarin and direct oral anticoagulants. Although these are effective, they also carry a risk of bleeding. Targeting other aspects of Virchow’s Triad may provide safer strategies to treat and prevent VT; studies investigating such strategies are required.
The potential benefits of platelet antagonism in VT remain unclear, but may yield additional alternate strategies appropriate for certain risk groups. Increased use of mechanical compression to prevent blood stasis may also reduce VT development. Identifying the optimal timing and use of this approach may improve VT prophylaxis. The physical contributions of blood flow and fibrin viscoelastic properties in VT are only starting to be investigated.
Given the variety of pathologic mechanisms suspected in venous thrombogenesis, a multi-pronged approach simultaneously targeting several components of Virchow’s Triad may be optimal for reducing VT. Further study into these areas may offer insights into novel therapeutic strategies for reducing thrombus formation or accelerating lysis of existing thrombi.
Acknowledgments
Fundings
The authors are supported by funding from the National Institutes of Health (R01HL126974 to A.S.W.) and the National Science Foundation (DGE-1144081 to J.R.B.).
The authors thank A. Phillip Owens, Ph. D., for reading the manuscript.
References
Full text links
Read article at publisher's site: https://doi.org/10.5482/hamo-16-09-0034
Read article for free, from open access legal sources, via Unpaywall:
https://europepmc.org/articles/pmc5680039?pdf=render
Citations & impact
Impact metrics
Citations of article over time
Article citations
Transformative Deep Vein Thrombosis Prophylaxis With Sequential Compression Devices in the Care of Hospitalized Patients.
Cureus, 16(10):e70639, 01 Oct 2024
Cited by: 0 articles | PMID: 39483555 | PMCID: PMC11526840
Review Free full text in Europe PMC
Are Gamers Prone to eThrombosis during Long Gaming Sessions?
Life (Basel), 14(4):525, 18 Apr 2024
Cited by: 0 articles | PMID: 38672795 | PMCID: PMC11051545
Composition of thrombi in zebrafish: similarities and distinctions with mammals.
J Thromb Haemost, 22(4):1056-1068, 30 Dec 2023
Cited by: 0 articles | PMID: 38160724
Immune cell-mediated venous thrombus resolution.
Res Pract Thromb Haemost, 7(8):102268, 20 Nov 2023
Cited by: 3 articles | PMID: 38193054 | PMCID: PMC10772895
Review Free full text in Europe PMC
Extracellular vesicles in venous thromboembolism and pulmonary hypertension.
J Nanobiotechnology, 21(1):461, 30 Nov 2023
Cited by: 0 articles | PMID: 38037042 | PMCID: PMC10691137
Review Free full text in Europe PMC
Go to all (24) article citations
Similar Articles
To arrive at the top five similar articles we use a word-weighted algorithm to compare words from the Title and Abstract of each citation.
Procoagulant activity in hemostasis and thrombosis: Virchow's triad revisited.
Anesth Analg, 114(2):275-285, 21 Nov 2011
Cited by: 155 articles | PMID: 22104070 | PMCID: PMC3264782
Review Free full text in Europe PMC
Fibrinogen, red blood cells, and factor XIII in venous thrombosis.
J Thromb Haemost, 13 Suppl 1:S208-15, 01 Jun 2015
Cited by: 36 articles | PMID: 26149026 | PMCID: PMC5975093
Review Free full text in Europe PMC
Pathophysiology of Trousseau's syndrome.
Hamostaseologie, 35(1):52-59, 18 Nov 2014
Cited by: 12 articles | PMID: 25403091
Review
The aetiology of deep venous thrombosis.
QJM, 99(9):581-593, 12 Aug 2006
Cited by: 44 articles | PMID: 16905749
Review
Funding
Funders who supported this work.
NHLBI NIH HHS (1)
Grant ID: R01 HL126974
National Institutes of Health (1)
Grant ID: R01HL126974 to A.S.W.
National Science Foundation (1)
Grant ID: DGE-1144081 to J.R.B.