Abstract
Background
Stress is widely known to alter behavioral responses to rewards and punishments. It is believed that stress may precipitate these changes through modulation of corticostriatal circuitry involved in reinforcement learning and motivation, although the intervening mechanisms remain unclear. One candidate is inflammation, which can rapidly increase following stress and can disrupt dopamine-dependent reward pathways.Methods
Here, in a sample of 88 healthy female participants, we first assessed the effect of an acute laboratory stress paradigm on levels of plasma interleukin-6 (IL-6), a cytokine known to be both responsive to stress and elevated in depression. In a second laboratory session, we examined the effects of a second laboratory stress paradigm on reward prediction error (RPE) signaling in the ventral striatum.Results
We show that individual differences in stress-induced increases in IL-6 (session 1) were associated with decreased ventral striatal RPE signaling during reinforcement learning (session 2), though there was no main effect of stress on RPE. Furthermore, changes in IL-6 following stress predicted intraindividual variability in perceived stress during a 4-month follow-up period.Conclusions
Taken together, these data identify a novel link between IL-6 and striatal RPEs during reinforcement learning in the context of acute psychological stress, as well as future appraisal of stressful life events.Free full text

Association Between Interleukin-6 and Striatal Prediction-Error Signals Following Acute Stress in Healthy Female Participants
Associated Data
Abstract
Background
Stress is widely known to alter behavioral responses to rewards and punishments. It is believed that stress may precipitate these changes through modulation of corticostriatal circuitry involved in reinforcement learning and motivation, although the intervening mechanisms remain unclear. One candidate is inflammation, which can rapidly increase following stress, and can disrupt dopamine-dependent reward pathways.
Methods
Here, in a sample of 88 healthy female participants, we first assessed the effect of an acute laboratory stress paradigm on levels of plasma interleukin-6 (IL-6), a cytokine known to be both responsive to stress and elevated in depression. In a second laboratory session, we examined the effects of a second laboratory stress paradigm on reward prediction error (RPE) signaling in the ventral striatum.
Results
We show that individual differences in stress-induced increases in IL-6 (session 1) were associated with decreased ventral striatal RPE signaling during reinforcement learning (session 2), though there was no main effect of stress on RPE. Further, changes in IL-6 following stress predicted intra-individual variability in perceived stress during a 4-month follow-up period.
Conclusions
Taken together, these data identify a novel link between IL-6 and striatal reward prediction errors during reinforcement learning in the context of acute psychological stress, as well as future appraisal of stressful life events.
Introduction
Stress is a major risk factor for psychiatric disorders (1–3), though its effects are not fully understood. Stress exposure can initiate a neuroendocrine cascade that modulates how individuals perceive and respond to rewarding or threatening cues in their environment (4–11). Specifically, it has been shown that stress may reduce acquisition of reward-related information (8, 12, 13) as well as disrupt normal reinforcer devaluation (9, 14, 15), two phenomena that are commonly observed in stress-related psychiatric disorders (16–20).
In animal models, substantial research has suggested that stress may induce these behavioral changes to rewarding stimuli via effects on the mesocorticolimbic dopamine (DA) system. Acute stressors transiently increase DA release in the nucleus accumbens (NAcc) while also promoting longer-term DAergic increases in the medial prefrontal cortex (mPFC) (10, 21–23). Interestingly, more recent studies have suggested that stress may have selective effects on DAergic responses to reward receipt (24), raising the possibility that behavioral changes to reinforcers may be mediated by the effects of stress on DAergic reward prediction error (RPE) signaling, a core mechanism of reinforcement learning (25, 26).
While early research on the relationship between stress, DAergic function, and subsequent behavioral changes focused on the role of the Hypothalamic-Pituitary-Adrenal (HPA) Axis (27), recent work has increasingly recognized an important role for stress-induced immune responses (28–31). As with glucocorticoids, pro-inflammatory cytokines such as interleukin-6 (IL-6), interleukin1 (IL-1), and tumor-necrosis factor alpha (TNF-alpha) can be stimulated by acute stress exposure (32–34). Behaviorally, acute administration of these pro-inflammatory cytokines has been shown to reduce sensitivity to rewards while augmenting sensitivity to punishment (35), a pattern that is consistent with evolutionary models (31) and matches the effects of acute stress (12, 13, 36) (though see also (6, 37, 38)). Importantly, only IL-6 has been reliably shown in meta-analyses to be both elevated in depression (39–42) as well as sensitive to laboratory measures of acute stress (33), and is increasingly recognized as a playing an important role in mood disorders (43).
A growing body of evidence suggests that DA and cytokines may influence each other through multiple pathways. Both acute and chronic treatment with cytokine inducers–including direct administration of IL-6–has been shown to disrupt DA synaptic availability and synthesis in rodents (44–46), non-human primates (47, 48), and humans (49). Similarly, in human functional neuroimaging studies, both chronic and acute administration of cytokine-inducers has been associated with blunted ventral striatal responses to reward anticipation (49, 50), prediction-error signaling during reinforcement learning (35), and novelty-driven activity in the DAergic midbrain (51). Alternatively, however, there is also growing evidence that DA signaling may modulate cytokine responses. DA receptors have been identified on numerous components of the innate immune system (52), including lymphocytes and T-Cells. These studies have largely suggested that DA acts to inhibit the actions of activated T-Cells. In particular, dopamine receptor D2 (DRD2) knockout mice show a remarkable anti-inflammatory response, suggesting that DA signaling may be primarily anti-inflammatory in nature (53, 54), though not in all cases (52).
Given these potentially bi-directional pathways between inflammation on DA signaling pathways, we predicted that stress-induced increases in inflammatory cytokines would be associated with a reduction in DA-dependent RPE signals during reinforcement learning. To date, however, no study has tested the relationship between stress-induced IL-6 and stress-related changes in striatal prediction error signaling and whether these mechanisms predict future levels of stress appraisal.
In the present study, we sought to address this question through a combination of laboratory stress challenges, plasma measures of IL-6, and functional neuroimaging in a sample of 88 healthy female participants assessed across two study visits (Figure 1). Only women were investigated owing to elevated prevalence of depression in females (55), as well as significant sex differences in psychological and hormonal responses to stress (56) that could substantially reduce our power to detect individual differences. During the first session, participants were exposed to the Maastricht Acute Stress Task (MAST; (57)), a robust laboratory stress paradigm, while blood was sampled intravenously. During the second session, participants completed a functional neuroimaging session that included functional runs of a reinforcement learning task (58) interleaved with blocks of a well-validated neuroimaging stress-paradigm, the Montreal Imaging Stress Task (MIST) (59). Effects of both stressors on mood were assessed using visual analog mood scales (VAMS) (60). We hypothesized that larger increases in IL-6 following stress (as assessed in the first behavioral session) would predict a greater blunting of RPE signals during stress (as assessed in the second session). After these laboratory visits, participants were followed for a period of four months to assess self-reported stressful experiences in daily life. For these assessments, we predicted that greater biological responses to laboratory stressors would predict self-reported stressful experiences during the follow-up period.
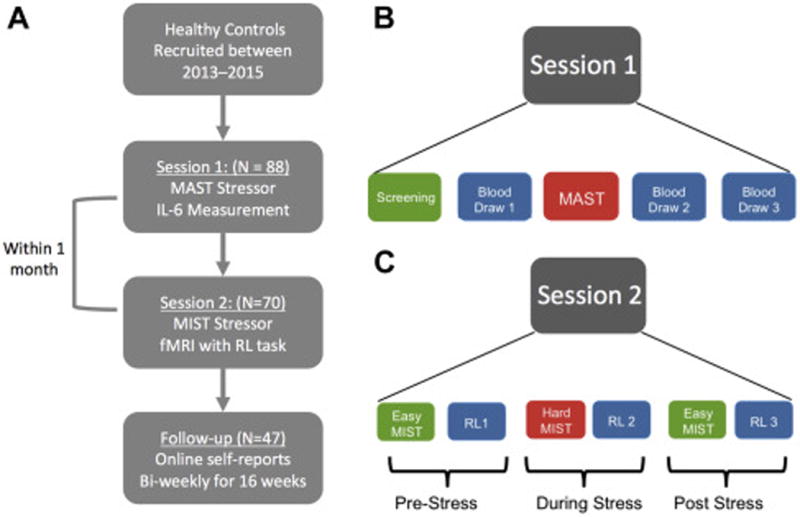
Schematic diagram illustrating the design of study sessions 1 and 2. A Overall flow of participants through the study. B. During session 1, participants first completed a SCID and other screening measures (see methods) a baseline blood draw, and then completed the MAST laboratory stress challenge. Following the MAST, two other blood draws were collected. C. During session 2, participants completed an fMRI scanning session in which they had to complete blocks of a reinforcement learning (RL) task that were interleaved between easy and hard (stressful) blocks of the Montreal Imaging Stress Task (MIST). For each of the 3 stress conditions (Pre-Stress, During Stress, Post-stress) runs of the MIST and RL were completed twice.
Methods and Materials
Participants and Study Description
A total of 88 healthy female participants were included in this study. For details on participant eligibility criteria, please see Supplemental Methods. All recruitment and testing procedures were approved by the Partners Institutional Review Board. The study was comprised of two laboratory visits followed by a 4-month period of self-report questions administered online every two weeks. Details of study procedures can be found in the Supplemental Methods. Subject demographic characteristics are summarized in Supplemental Table S1.
Session 1 – MAST Laboratory Stressor
To induce stress during the first session, participants completed the Maastricht Acute Stress Test (MAST; (57)). The MAST is a laboratory stress paradigm that combines alternating periods of well-validated stress-inducing procedures including a cold pressor and performance of serial subtraction in front of evaluators. For details of the MAST administration, please see Supplemental Methods.
Session 1: Sample collection and Analysis
To assess IL-6 responses, plasma samples were drawn intravenously at −10 minutes (before stressor), +45 minutes following stressor and +90 minutes following stressor. To assess salivary cortisol, saliva samples were collected at six time-points: −110 minutes (before stressor), −30 minutes, immediately before stressor, +20 minutes following stressor, +35 minutes, and +80 minutes. For details of collection and analysis, please see Supplemental Methods.
Session 2: Laboratory Stressor
For the session 2’s laboratory stressor (Figure 1), which was performed during an fMRI scan, we used a modified version of the Montreal Imaging Stress Task (MIST; (59)), a widely used and well-validated stress-paradigm. Briefly, this task requires participants to solve arithmetic problems while their performance is publicly evaluated. For details of the MIST administration, please see Supplemental Methods. To assess salivary cortisol during session 2, saliva samples were collected at four time-points: Prior to entry into the scanner, 3 minutes prior to onset of stress blocks, +25 minutes after the onset of the stress blocks, +40 minutes after the onset of the stress blocks.
Reinforcement Learning Task
To assess RPE signals participants were asked to complete a well-validated instrumental conditioning paradigm (58). Details of the task are presented in the Supplemental Methods). Briefly, subjects were instructed to choose between two visual stimuli displayed on a screen. Each of the stimuli in the pairs was associated with either an 80% or 20% probability of a given outcome (gain: win $1 or $0; loss: lose $1 or $0; neutral: look at grey square or nothing. There were a total of 6 RL runs across the experiment, with 2 runs for each stress condition (“Pre-Stress”, “During-Stress”, “Post-Stress”).
Primary analysis focused on a parametric modulation (pmod) contrast for RPE signals extracted from an anatomically defined NAcc mask. For details on the computational model, neuroimaging acquisition, processing and ROI analysis please see Supplemental Materials.
Follow-up Period
To examine the ecological validity of biological responses to laboratory stressors, all participants were asked to complete online self-report questionnaires every two-weeks for a 4-month follow-up period. Our primary measures of interest was the Perceived Stress Scale (PSS; (62)), which was used to assess ongoing perceptions of stress in daily life. We examined both mean-level of perceived stress as well as variability over time. To assess variability, we calculated mean sum of squared differences (MSSD), a standard metric used to capture variability in symptom experience (63).
Results
Session 1: Effects of Acute Stress on Plasma IL-6 and Salivary Cortisol
Using a 3 (Timepoints) repeated measures ANOVA, we found that the MAST induced a significant increase in plasma IL-6 (F(1.43,92) = 17.89, p = 8.0 × 10−6; partial η2 = 0.28) (Figure 2). This effect remained highly significant when controlling for menstrual cycle phase (70% follicular; 30% luteal) (F(1.43,90) = 16.77, p = 1.6 × 10−5; partial η2 = 0.27), and there was no Timepoints × Menstrual Cycle Phase interaction (F(1.43,90) = 0.89, p = 0.384). There was, however, a main effect of cycle phase such that participants in the luteal phase had lower levels of IL-6 than those in the follicular phase (F(1,45) = 5.24, p = 0.027; partial η2 = 0.10). Given prior studies (64), we also examined whether BMI was associated with change in IL-6, but did not find association between BMI and change in IL-6 following stress (see Supplemental Table S2). Baseline PSS scores were also unrelated to change in IL-6 levels (Spearman r = 0.10, p = 0.466), though we did observe baseline associations with the State-Trait Anxiety Inventory, (see Supplemental Materials).
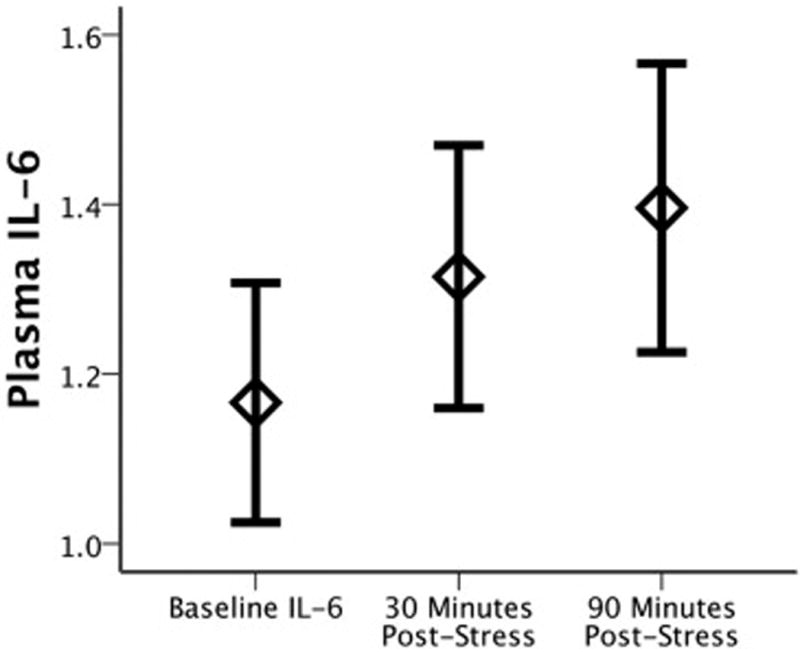
Change in plasma IL-6 levels (raw values) following the Maastricht Acute Stress Task (MAST). Error bars represent standard error.
Additionally, using a 6 (Timepoints) repeated measures ANOVA, we found that the MAST produced a significant increase in salivary cortisol (F(2.34,182.38.) = 27.87, p = 1.5 × 10−12), with a strong quadratic effect (F(1,78) = 33.14, p = 1.62 × 10−7) (see Supplemental Figure S1).
Session 1: Effects of Acute Stress on Mood and Relationships to IL-6
Using an 8 (Timepoints) × 5 (Questions) repeated-measures ANOVA, we found that the MAST stressor during session 1 induced a significant overall change in mood (F(3.28,553) = 70.78, p = 1.78 × 10−35), with the expected quadratic effect (F(1,79) = 125.05, p = 5.98 × 10−18) showing an increase in negative mood following the stressor (Figure 3A). This quadratic effect remained significant when controlling for Menstrual Cycle Phase (F(1,77) = 30.56, p = 4.26 × 10−7), and there was no interaction between this quadratic effect and Menstrual Cycle Phase (F(1,77) = 0.064, p = 0.801). For each individual VAMS question, quadratic effects revealed that participants felt immediately following the MAST less happy (F(1,80) = 113.84, p = 4.87 × 10−17), relaxed (F(1,80) = 98.01, p = 1.51 × 10−15), friendly (F(1,80) = 114.65, p = 4.11 × 10−17), sociable (F(1,80) = 66.79, p = 3.71 × 10−12), and quick witted (F(1,80) = 67.08, p = 3.71 × 10−12).
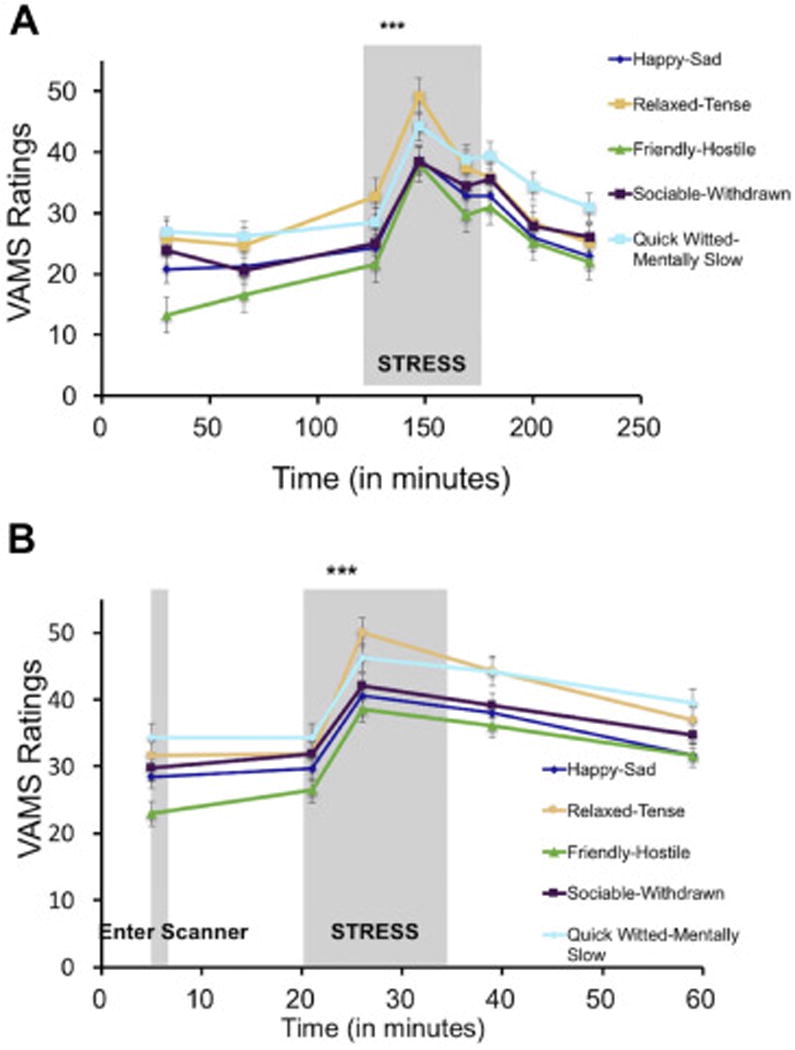
Stress manipulations increase negative affect. A. In session 1, the Maastricht Acute Stress Task (MAST) induced a significant increase in negative affect across all 5 VAMS questions (Happy-Sad, Relaxed-Tense, Friendly-Hostile, Sociable-Withdrawn, Quick Witted-Mentally Slow). B. Similarly, in session 2, the Montreal Imaging Stress Task (MIST) also induced a significant decrease in mood across all 5 VAMS questions. All VAMS items are scored such that higher scores indicate more negative affect.
There were no relationships between change in IL-6 levels in response to the MAST and change in mood ratings as assessed by any of the five VAMS questions: (happy: Spearman r = 0.06, p = 0.663; relaxed: Spearman r = 0.13, p =0.345; friendly: Spearman r = .10, p = 0.473; sociable: Spearman r = .001, p = 0.992; quick witted: Spearman r = 0.02, p = 0.868).
Session 2: Effects of Acute Stress on Mood and Salivary Cortisol
Using a 5 (Timepoints) × 5 (Questions) repeated-measures ANOVA, we found that the MIST stressor during session 2 also induced a significant overall decrease in mood (F(2.05,260) = 50.65, p = 2.46 × 10−17) with a quadratic effect (F(1,65) = 67.85, p = 1.10 × 10−11) (Figure 3B). This quadratic effect remained significant when controlling for Menstrual Cycle Phase (F(1,61) = 7.28, p = 0.009), and there was no interaction between this quadratic effect and Menstrual Cycle Phase (F(1,61) = 0.003, p = 0.960). Specifically, participants reported feeling immediately following the MIST less happy (F(1,65) = 46.60, p = 3.51 × 10−9), relaxed (F(1,65) = 39.75, p = 2.88 × 10−8), friendly (F(1,65) = 62.92, p = 3.85 × 10−11), sociable (F(1,65) = 36.48, p = 8.27 × 10−8), and quick witted (F(1,65) = 24.56, p = 5.0 × 10−6). In addition to these main effects, individual differences in mood responses to stress were significantly correlated between the MAST (Session 1) and MIST (Session 2) stressors for all 5 questions (happy: Pearson r = 0.48, p = 0.0002; relaxed: r =0.31, p = 0.019; friendly: r = 0.35, p = 0.007; sociable: r = 0.38, p = 0.004; quick-witted: r = 0.46, p = 0.0004).
For salivary cortisol, a 3 (Timepoints) Repeated Measures ANOVA revealed no main effect of the MIST stressor on cortisol (F(1.71,116.06) = 21.31, p = 0.437) (See Supplemental Figure S1). This null result was driven by the absence of a positive cortisol response in approximately half of the participants, which is consistent with other studies using the MIST (59, 65). Importantly however, %change in cortisol from pre-stress to post stress during session 1 was positively correlated with %change in cortisol from pre-stress to post-stress during session 2 (Pearson r = 0.40, p = 0.006).
Session 2: Effects of Acute Stress on Behavioral Performance
A 2 (Valence: win/loss) × 3 (Stress Condition: Pre-, During-, Post-Stress) ×2 (Run Number) repeated measures ANOVA with Menstrual Cycle Phase included as a between-groups variable revealed a main effect of the Stress Condition such that performance accuracy increased over the course of the experiment (F(2,106) = 3.30, p = 0.041). There was no main effect of Valence (win/loss) (F(1,53) = 2.5, p = 0.120) nor Stress Condition × Valence interaction (F(2,106) = 1.60, p = 0.21), though follow-up t-tests did reveal a significant improvement in performance on loss trials during stress as opposed to pre-stress (t(62) = 2.96, p = 0.004), with no change in accuracy for win-trials (t(62) = 0.20, p = 0.842).
There was no main effect of Menstrual Cycle Phase, nor any interactions with Menstrual Cycle Phase and Stress Condition, though there was a significant interaction between Menstrual Cycle Phase and Valence (F(1,52) = 7.94, p = 0.007) such that women in the luteal phase showed a greater overall accuracy for win trials relative to loss trials, while women in the follicular phase showed little difference between the two.
Session 2: Prediction Error Signaling
Averaging across all RL sessions, we observed a main effect of positive RPE signals in the NAcc using a small volume correction (SVC) with a bilateral NAcc anatomical mask drawn from the Harvard-Oxford probabilistic atlas (SVC Left NAcc: × = −6, y = 10, z = −6, t = 5.25, pFWE = 0.0005; SVC Right NAcc: × = 8, y = 6, z = −4, t = 4.69, pFWE = 0.003) (Figure 4A). For negative RPE, a whole-brain analysis revealed significant activity in bilateral anterior insula and areas of dorsal anterior cingulate and dorsomedial prefrontal cortex (for a full list of regions identified by RPE contrasts, please see Supplemental Table S3). There was no main effect (linear or quadratic) of the MIST stress manipulation on the magnitude of positive or negative RPE signals. Consistent with prior studies (58, 66), the strength of positive RPE signals in the NAcc was positively associated with performance accuracy across win and loss trials accuracy (see Supplemental Materials).
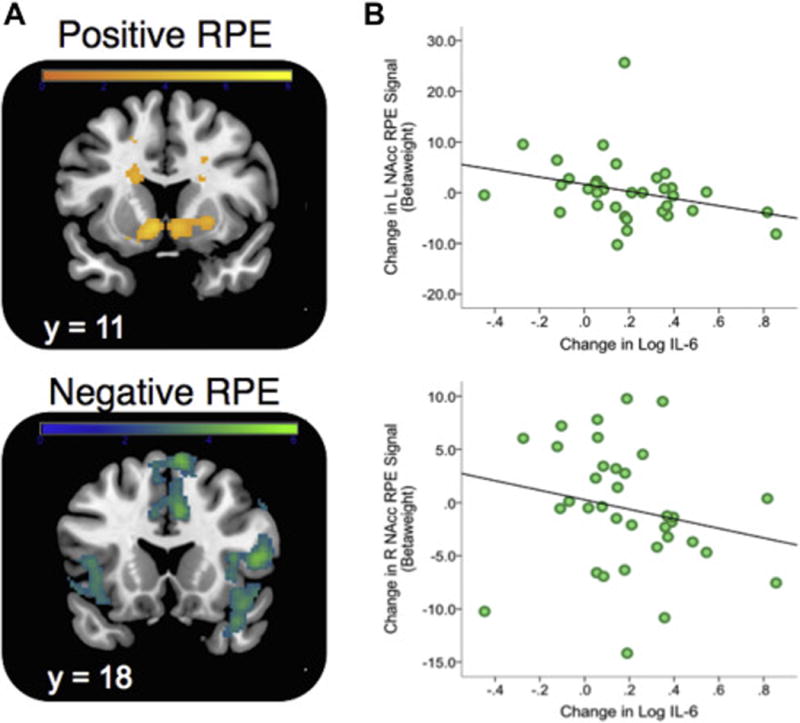
Positive and Negative RPE signals during reinforcement learning and relationship to stress-induced change in IL-6. A. Model-based PE signals averaged across all three stress conditions (Pre-, During- and Post-stress) and found to predict activity in ventral striatum (SVC; positive RPE) and bilateral insula/dorsal anterior cingulate cortex (negative RPE). All reported regions were corrected for multiple-comparisons. Activation patterns are shown using an uncorrected height threshold of t > 2.5 for visualization purposes. B. Association between stress-induced change in Left (top) and Right (bottom) NAcc Positive RPE beta weight (RPE contrast Pre Stress – During Stress) and change in plasma IL-6 following stress. Note: Extracted values for right and left nucleus accumbens (NAcc) ROI were defined anatomically to avoid statistical non-independence (see Methods). Note that for L NAcc, one subject was a univariate outlier (Z = 4.38), but the association with change in IL-6 was unchanged when including (r = −0.39, p = 0.019) or excluding this subject (r = −0.42, p = 0.014)
Session 2: Stress-induced change in RPE signals and IL-6 (assessed in session 1)
Using extracted RPE betaweights from an anatomically defined NAcc ROI, we examined the relationships between change in IL-6 during stress (assessed in session 1) and change in NAcc RPE betaweights following stress (assessed in session 2). We observed an inverse relationship such that larger increases in IL-6 following stress at times 2 and 3 were associated with larger decreases in NAcc PE beta weights following stress (see Table 1 and Figure 4B). This effect was strongest in the left NAcc for the comparison of Pre-Stress > Post-Stress RPE signals. Importantly, the association between IL-6 and RPE remained when controlling for change in cortisol (β = −0.60, t = −3.54, p = 0.002). This targeted ROI analysis was also followed by a whole-brain analysis for both positive and negative RPE contrasts, but no region showed a significant association after controlling for multiple comparisons. There were no significant associations with baseline IL-6 and NAcc RPE across the Pre, During and Post-Stress time points, though these associations were not significantly different from the correlations observed using difference scores (see Supplemental Table S4).
Table 1
Spearman correlations between stress-induced change in IL-6 and change in striatal RPE signals
Log IL-6 Increase Time 1 to Time 2 | Log IL-6 Increase Time 1 to Time 3 | |
---|---|---|
Change in L Nacc RPE from Pre-Stress to During-Stress | −0.08 | −0.10 |
Change in R Nacc RPE from Pre-Stress to During-Stress | −0.21 | −0.34* |
Change in L Nacc RPE from Pre-Stress to Post-Stress | −0.39** | −0.39* |
Change in R Nacc RPE from Pre-Stress to Post-Stress | 0.04 | −0.16 |
Nacc: Nucleus accumbens
Follow-up Data
To assess how well inflammatory responses to a laboratory stressor predicted perceived stress over the 4-month follow-up period, we examined associations between stress-induced IL-6 levels and mean PSS scores as well as mean sum of squared differences (MSSD) in PSS scores. The latter is a commonly used measure of symptom variability over time (63). There was no relationship between stress-induced change in IL-6 response and average PSS score over the 4-month time period. However, for participants followed for at least 1 month with available IL-6 data (N = 47), greater change in IL-6 following stress predicted heightened variability of perceived stress (r = 0.39, p = 0.007; Supplemental Figure S2). We detected a similar effect for participants followed for at least 2 months (N = 44, r = 0.37, p = 0.014), 3 months (N = 40, r = 0.46, p = 0.003), and for participants completing the full 4 months of follow-up data (N = 31, r = 0.48, p = 0.007).
To demonstrate these relationships were not driven solely due to the effects of mood during the MAST, multiple regression analyses were conducted to evaluate the relationship between change in IL-6 following stress and variability of perceived stress when controlling for changes in mood ratings. When controlling for VAMS rating changes, stress-induced change in IL-6 predicted perceived stress variability more strongly (β = 0.60, t = 4.53, p = 0.00005). As an additional control, we examined whether this association remained present when controlling for baseline PSS scores, and findings were confirmed (β = −0.60, t = −3.54, p = 0.002). Finally, we additionally examined whether changes in RPE signals were similarly predictive of PSS variability, but did not observe a significant relationship for either left (r = −0.20, p = 0.146) or right (r = 0.03, p = 0.829) NAcc ROIs.
Discussion
In this study we observed that stress-induced IL-6 was significantly predictive of subsequent stress-induced changes in NAcc RPE signals during reinforcement learning. In addition, stress-induced change in IL-6 predicted variability of perceived stress in daily life over the ensuing four months even when controlling for stress-induced changes in mood. To our knowledge, this is the first study with a prospective component to link IL-6 and striatal RPE responses to stress, suggesting that individual differences in immune responses to stress may be a marker of vulnerability for stress-related effects on reward processes.
The relationship between cytokines and DA signaling is complex, and prior work suggests possible bi-directional pathways that may account for our observed relationships. One possibility is that acute increases in IL-6 may suppress striatal DA, thereby disrupting RPE signals (67). Evidence for such rapid (< 30 minutes) effects of systemic IL-6 injections on striatal DA has been found in several rodent microdialysis studies (44, 45). Moreover, such effects appear somewhat specific to striatal DA levels, and have not been detected in other regions (e.g., (68)). This interpretation is also consistent with prior work in humans showing that acute administration of cytokine inducers leads to blunted ventral striatal activity following reward cues (50), RPE signals (35), and midbrain responses to novelty (51). Similarly, chronic exposure to cytokine induces has been shown to reduce DA availability and synthesis in primates (47, 49). One caveat to this interpretation is the timing of IL-6 changes. While a statistically significant increase was observed within 30-minutes of the MAST, the magnitude of the increase was small. It is unclear whether this small increase would be sufficient to have a major effect on striatal DA. Moreover, the MIST was a less potent stressor. Consequently, it may the that the relationship is better conceptualized as a marker of individual differences in immune-striatal interactions as compared to a casual description of the direct effects of increased IL-6 on striatal function.
An alternative possibility, however, is that lower levels of DA may influence cytokine responses to stress. As noted in the introduction, DA receptors have been identified on a variety of cells within the innate immune system, including T-cells and lymphocytes (52), and may regulate immune responses in the body and brain at multiple levels. Consequently, the observed relationship may be driven by the effects of stress-induced DA release on cytokine signaling. Additionally, it should be emphasized that while our analyses focused on the association between change in IL-6 and change in RPE following stress, these results should not be taken to suggest that baseline levels in either case are necessarily unrelated.
In addition to the association between inflammatory responses to stress and RPE signals, we also observed that the magnitude of IL-6 increases following stress were predictive of variability in perceived stress during a 4-month follow-up period, but not overall mean level of perceived stress. Initially, we had hypothesized that both mean and variability on PSS might be related to IL-6 responses. One explanation for this discrepancy from our hypotheses is that mean level of stress may be more determined by the presence or absence of external stressors than variability. Importantly, we found that this relationship was robust, and remained significant even when controlling for sample attrition, baseline PSS scores, and stress-induced change in mood, thereby helping extend the ecological validity of our laboratory-based stress paradigms as a means to probe neurobiological responses to stress. Variability of symptom and risk factor expression is increasingly recognized as an important marker of psychological disorders (69–72), and our data suggest that variability–rather than mean level–may be a critical factor.
An important potential caveat to our findings is the lack of concurrent assessment for all measures, particularly given the absence of main effect of the MIST stressor (session 2) on striatal RPE signals or salivary cortisol. This raises the possibility that the second stress manipulation (MIST) was not as effective as the first one (MAST), and could limit the interpretability of the Pre- vs. Post- stress change in RPE signals. Specifically, it is possible that changes in RPE signals were not due to stress, given the weakness of the MIST stressor and the use of a fixed-order design, which was chosen to maximize power for individual differences analysis. Arguing against this point is the fact that there were clear increases in negative affect, and individual differences in both salivary cortisol and mood reactivity to the MAST (session 1) and MIST (session 2) stressors were correlated, suggesting that while the session 2 stressor had a less potent effect overall, the examination of individual differences across the two session is still valid (64).
There are several other limitations worth noting. First, our sample included female participants only. This was done to limit sex-based heterogeneity in hormonal response to stress but it is unclear whether the current findings will extend to males. While possible sex differences is a critical question, the inclusion of both genders would likely have significantly reduced our statistical power for identifying individual differences. Additionally, our study design required multiple stress sessions, which may have produced some degree of habituation. Still, we observed clear affective responses to both stressors (Figure 3), and we likely reduced habituation by using two different stress manipulations. Additionally, caution is warranted in attributing the observed changes in RL performance accuracy to the stress manipulation due to the lack of a no-stress control group for the neuroimaging session. We also note that for collection of plasma samples, we used an intravenous catheter, which may have itself stimulated some degree of IL-6 production (73). That said, this effect has generally only been observed over longer time periods (e.g., > 3 hours) than were required for the current study (74). Additionally, we note that while IL-6 is generally conceptualized as being pro-inflammatory (43) it is important to note that it can also be, anti-inflammatory depending on the target (43, 75, 76).
In sum, we found that stress-induced changes in IL-6 levels were associated with both striatal reward prediction errors during reinforcement learning as well as stress sensitivity during a 4-month follow-up period. These data have important implications for understanding the relationships between stress, IL-6, and their impact on reward-related corticostriatal circuitry.
Supplementary Material
1
2
3
4
Acknowledgments
This work was support by the National Institutes of Mental Health (R01 and R37 MH068376, MH068376-09S1 to DAP). MTT was supported by K99/R00MH102355 and R01MH108605. RA was supported a Brain and Behavior Research Foundation Young Investigator award, respectively. The content is solely the responsibility of the authors and does not necessarily represent the official views of the National Institutes of Health. The authors also gratefully acknowledge support from Gary Bradwin, Dan Cole, Nancy Brooks, Dave Crowley, Laurie Scott, Randy Auerbach and Christian Webb as well as the staff of the Laboratory for Affective and Translational Neuroscience at McLean Hospital.
Footnotes
Publisher's Disclaimer: This is a PDF file of an unedited manuscript that has been accepted for publication. As a service to our customers we are providing this early version of the manuscript. The manuscript will undergo copyediting, typesetting, and review of the resulting proof before it is published in its final citable form. Please note that during the production process errors may be discovered which could affect the content, and all legal disclaimers that apply to the journal pertain.
Financial Disclosures
Over the past 3 years, MTT has served as a paid consultant to Avanir Pharmaceuticals, NeuroCog, BlackThorn Pharmaceuticals and the Boston Consulting Group. He has also received honoraria and royalties related to his writing. Over the past 3 years, Dr. Pizzagalli has received consulting fees from Akili Interactive Labs, BlackThorn Therapeutics, Pfizer, and PositScience for activities unrelated to the current research. No funding or sponsorship was provided by these companies for the current work, and all views expressed herein are solely those of the authors. All other authors report no biomedical financial interests or potential conflicts of interest.
References
Full text links
Read article at publisher's site: https://doi.org/10.1016/j.biopsych.2017.02.1183
Read article for free, from open access legal sources, via Unpaywall:
https://europepmc.org/articles/pmc5610086?pdf=render
Citations & impact
Impact metrics
Citations of article over time
Alternative metrics
Smart citations by scite.ai
Explore citation contexts and check if this article has been
supported or disputed.
https://scite.ai/reports/10.1016/j.biopsych.2017.02.1183
Article citations
Annual Research Review: Neuroimmune network model of depression: a developmental perspective.
J Child Psychol Psychiatry, 65(4):538-567, 01 Mar 2024
Cited by: 3 articles | PMID: 38426610
Review
Elevated serum leptin is associated with attenuated reward anticipation in major depressive disorder independent of peripheral C-reactive protein levels.
Sci Rep, 13(1):11313, 13 Jul 2023
Cited by: 1 article | PMID: 37443383
Reward and Immune Systems in Emotion (RISE) prospective longitudinal study: Protocol overview of an integrative reward-inflammation model of first onset of major depression in adolescence.
Brain Behav Immun Health, 30:100643, 29 May 2023
Cited by: 3 articles | PMID: 37304334 | PMCID: PMC10250584
The acute effects of stress on dishonesty are moderated by individual differences in moral default.
Sci Rep, 13(1):3984, 09 Mar 2023
Cited by: 0 articles | PMID: 36894617 | PMCID: PMC9998439
Increased Inflammation and Treatment of Depression: From Resistance to Reuse, Repurposing, and Redesign.
Adv Neurobiol, 30:387-416, 01 Jan 2023
Cited by: 2 articles | PMID: 36928859
Go to all (38) article citations
Data
Data behind the article
This data has been text mined from the article, or deposited into data resources.
BioStudies: supplemental material and supporting data
Similar Articles
To arrive at the top five similar articles we use a word-weighted algorithm to compare words from the Title and Abstract of each citation.
Aberrant Salience Is Related to Reduced Reinforcement Learning Signals and Elevated Dopamine Synthesis Capacity in Healthy Adults.
J Neurosci, 35(28):10103-10111, 01 Jul 2015
Cited by: 29 articles | PMID: 26180188 | PMCID: PMC6605337
Beta Oscillations in Monkey Striatum Encode Reward Prediction Error Signals.
J Neurosci, 43(18):3339-3352, 04 Apr 2023
Cited by: 4 articles | PMID: 37015808 | PMCID: PMC10162459
Chronic alcohol intake abolishes the relationship between dopamine synthesis capacity and learning signals in the ventral striatum.
Eur J Neurosci, 41(4):477-486, 26 Dec 2014
Cited by: 18 articles | PMID: 25546072 | PMCID: PMC4455879
Involvement of basal ganglia and orbitofrontal cortex in goal-directed behavior.
Prog Brain Res, 126:193-215, 01 Jan 2000
Cited by: 122 articles | PMID: 11105648
Review
Funding
Funders who supported this work.
NIDA NIH HHS (1)
Grant ID: K08 DA037465
NIMH NIH HHS (4)
Grant ID: R01 MH108605
Grant ID: R37 MH068376
Grant ID: R01 MH068376
Grant ID: R00 MH102355