Abstract
Free full text

IL-32γ promotes integrin αvβ6 expression through the activation of NF-κB in HSCs
Abstract
Hepatic stellate cell (HSC) activation is important in the pathogenesis of liver fibrosis. However, the molecular mechanism of HSC activation is not completely understood. In the present study, it was demonstrated that interleukin-32γ (IL-32γ) is capable of enhancing intefgrin αvβ6 expression by inducing integrin αvβ6 promoter activity in a dose-dependent manner in HSCs. Furthermore, it was determined that nuclear factor κB (NF-κB) activation is required for IL-32γ-induced integrin αvβ6 expression. Increased integrin αvβ6 expression is then able to activate HSCs. These results indicate that NF-κB activation is required for IL-32γ to induce integrin αvβ6 expression and consequently promote HSC activation. Therefore, IL-32γ activates HSCs and therefore may be associated with hepatic fibrogenesis. These results may enable the development of novel effective strategies to treat hepatic fibrosis.
Introduction
Hepatic fibrosis is the production of excessive amounts of connective tissue, defined by excessive accumulation of collagenous and non-collagenous extracellular matrix (ECM) in the liver. It is the result of wound-healing responses triggered by proinflammatory and profibrotic cytokines produced by cells following either acute or chronic liver injury (1,2). The livers of patients with hepatic fibrosis may lose their pliability and function. Numerous factors, including tissue damage arising from alcohol abuse (3), infections (hepatitis B/C virus), autoimmune diseases, foreign material (silicone mammary implants and gastric banding) and tumors (2), may cause liver injury and consequently stimulate the formation of liver fibrosis.
Morbidity and mortality rates of patients with hepatic fibrosis are high as there are currently no efficient methods of treating hepatic fibrosis. Therefore, hepatic fibrosis from chronic hepatitis is a major global health concern. Researchers have demonstrated that hepatic fibrosis could be reversed (4,5), but the molecular mechanism of hepatic fibrosis is not completely understood. Therefore, improving understanding regarding the molecular mechanisms of hepatic fibrosis is critical. This could provide novel targets for new antifibrotic therapies or even allow the development of novel techniques to reverse hepatic fibrosis.
Hepatic stellate cells (HSCs) are located in the space of Disse and closely interact with sinusoidal endothelial and hepatic epithelial cells (6). Vitamin A is present in the HSCs of a healthy liver; however, following liver injury, the amount of vitamin A in HSCs is decreased and HSCs are activated, leading to the deposition of excessive ECM (7). Compared with inactivated HSCs, activated HSCs exhibit a different phenotype, undergo proliferation and contractility and lose retinoid stores. Activated HSCs also secrete lipoproteins, growth factors and cytokines (8). Furthermore, activated HSCs are important in the progression from hepatitis to liver fibrosis (1). However, the precise mechanism of HSC activation remains unclear.
Integrins are a large family of transmembrane cellular protein receptors, which are composed of non-covalently linked α- and β-subunits (9) and can form ≥24 different combinations. Various cell types express different integrins that can recognize multiple ligands (9). For example, integrins can recognize the arginine-glycine-aspartic acid (RGD) sequence on their respective ligands. Following ligation of their relative ligands, integrins adhere to ECM and recruit various signal and adaptor proteins to form focal adhesions, organize the cytoskeleton and cell shape and influence cell migration (10). Furthermore, integrins affect the fate and function of cells by influencing their proliferation, differentiation and apoptosis (11).
Integrin αvβ6 binds to the RGD sequence (9) and to fibronectin. Additionally, it can activate non-receptor tyrosine kinases, focal adhesion kinase (FAK) on (Tyr397) and the Src family of kinases, and activate the FAK/extracellular signal-regulated kinase (ERK)/nuclear factor κB (NF-κB) signaling pathway (12). Osteopontin (OPN), an ECM cytokine, is expressed in HSCs and can activate the phosphoinositide 3-kinase/phosphorylated Akt (PI3K/pAkt)/NF-κB-signaling pathway and modulate the HSCs pro-fibrogenic phenotype and collagen I expression. Furthermore, integrin αvβ3 participates in the fibrogenesis process of OPN (13). Activated myofibroblastic rat and human stellate cells express integrin αvβ3 and it has been demonstrated that antagonizing integrin αvβ3 using neutralized antibodies, echistatin or small inhibitory RNA through the silence αv subunit expression inhibits stellate cell proliferation (14).
Interleukin (IL)-32, a multifunctional cytokine, is involved in multiple diseases, including infections, chronic inflammation and cancer (15). It contains an Arg-Gly-Asp (RGD) motif, important for cell adhesion processes (14). Furthermore, natural killer cells, T-cells, monocytes, primary peripheral blood mononuclear cells, fibroblasts, epithelial cells and keratinocytes are capable of producing interleukin (IL)-32. IL-32 has six major splice variant isoforms, known as IL-32α, IL-32β, IL-32γ, IL-32δ, IL-32ε and IL-32ζ (15), the most potent of which is IL-32γ (15). IL-32α can trigger the typical cytokine signaling pathways of NF-κB and p38 mitogen-activated protein kinase and induce expression of several proinflammatory cytokines, including tumor necrosis factor-α and IL-8 (16). Furthermore, the IL-32 RGD motif interacts with the extracellular part of integrin αVβ3 and αVβ6, and can bind to intracellular proteins including paxillin and FAK (17). Therefore, it serves a dual role in integrin signaling (18). Previous studies by our group determined that IL-32 expression is induced by hepatitis B virus protein X through the activation of NF-κB (19,20). IL-32 expression is increased in chronic hepatitis B virus-infected liver and is associated with HBV-related liver inflammation and fibrosis (19). Thus, it is hypothesized that IL-32 may participate in the pathogenesis of liver fibrosis through the integrin/FAK-signaling pathway. In the present study, the IL-32/integrin/FAK transcriptional network in HSCs was examined in order to understand the hepatic fibrogenesis mechanism.
Materials and methods
Cell culture, transfection and treatment
Cells from the well-established human HSC LX-2 cell line (purchased from BNCC, Suzhou, China) were cultured as previously described (21). LX-2 cells were plated at a density of 4×105 cells/well in a 6-well plate in RPMI-1640 (Gibco; Thermo Fisher Scientific, Inc., Waltham, MA, USA) containing 10% fetal bovine serum (FBS; Gibco; Thermo Fisher Scientific, Inc.), 100 µg/ml penicillin and 100 µg/ml streptomycin at 37°C in a 5% CO2 incubator. LX-2 cells were treated with SN50 (NF-κB SN50; 213546-43-3; Merck KGaA, Darmstadt, Germany) at different concentrations (0, 25, 50, 75 and 100 ng/ml) and then treated with IL-32γ (Accession no. P24001; R&D Systems, Inc., Minneapolis, MN, USA) at different concentrations, (0, 5, 10, and 20 ng/ml).
HepG2 cells (a human hepatocellular cell line) was obtained from the Central Laboratory of The Third Affiliated Hospital, Sun Yat-sen University (Guangzhou, China). HepG2 cells were plated at a density of 5×105 cells/well in a 6-well plate in Dulbecco's modified Eagle's medium (Gibco; Thermo Fisher Scientific, Inc.) supplemented with 10% FBS, 100 µg/ml penicillin and 100 µg/ml streptomycin at 37°C in a 5% CO2 incubator. The stable HepG2 cell line was transfected with IL-32γ plasmid pcDNA3.1-IL-32 and control empty pcDNA3.1 vector plasmid (provided by Dr Xingdong Cai, Department of Respiratory Medicine, The First Affiliated Hospital, Jinan University, Guangzhou, China). Cells were transfected using Lipofectamine® 2000 (Invitrogen; Thermo Fisher Scientific, Inc.) according to the manufacturer's instructions and selected with G418 (Gibco; Thermo Fisher Scientific, Inc.). The amount of IL-32 present in cell culture supernatant after the stable transfection of pcDNA3.1-IL-32 or the empty pcDNA3.1 vector plasmid cells was confirmed by ELISA (IL-32 ELISA kit; cat. no. 433504; BioLegend, Inc., San Diego, CA, USA) according to the manufacturer's instructions.
Plasmid construction
Promoter binding sites of integrin αvβ6 were analyzed using Genomatix Software Suite online (www.genomatix.de/index). Three binding sites were identified for NF-κB in the integrin αvβ6 promoter region (at −406 to −392, −139 to −125 and +431 to +445). Integrin αvβ6 promoter (604/+92) containing the sequence from −604 to +92 (including binding sites of NF-κB and relative to the transcriptional start site) was amplified from human genomic DNA [purified from normal human red blood cells obtained from a healthy donor using TM Blood DNA Midi kit (Omega Bio-Tek, Inc., Norcross, GA, USA)] by polymerase chain reaction (PCR) using a PCR Amplification kit (DR011; Takara Biotechnology Co., Ltd.), according to the manufacturer's protocol. The study was approved by the local scientific Ethics Committee. The following primers were used: 5′-GTTACGCGTTAGCCTTCCTTCTCATTTAC-3′ (forward) and 5′-GTTAAGCTTGAACGCAGGTCTTACCTTGT-3′ (reverse), in which the MluI and HindIII sites were introduced, respectively. Subsequently, the products were inserted into the pGL3-basic vector to generate the integrin αvβ6 promoter and luciferase gene fusion plasmid (integrin αvβ6-Luc). The pGL3-basic vector was kindly provided by Professor Guanxin Shen at the Department of Immunology, Tongji Medical College, Huazhong University of Science and Technology (Wuhan, China).
Transient transfection and luciferase reporter gene assays
LX-2 cells were plated at a density of 4×105 cells/well in 6-well plates in RPMI-1640 containing 10% FBS, 100 µg/ml penicillin and 100 µg/ml streptomycin at 37°C in a 5% CO2 incubator. Following 24 h, cells were transfected with p50 or p65 expressing plasmids. Lipofectamine® 2000 (Invitrogen; Thermo Fisher Scientific, Inc.) was used to transfect the plasmids. For the luciferase assay, cells were co-transfected with expression, promoter reporter and the pRL-TK plasmids (Promega Corporation, Madison, WI, USA). After 6 h, the cells were washed and allowed to recover in fresh medium supplemented with 1% FBS (Gibco; Thermo Fisher Scientific, Inc.). After 48 h, luciferase activity was detected using the Dual Luciferase® Reporter assay system (Promega Corporation), following the manufacturer's instructions. Relative luciferase activity was determined using a Modulus Laboratory Luminometer (Turner Biosystems; Promega Corporation). Finally, transfection efficiency was normalized using the renilla luciferase activity in each transfection as an internal control.
RNA extraction and reverse transcription-quantitative polymerase chain reaction (RT-qPCR)
Total RNA was prepared following TRIzol (Takara Biotechnology Co., Ltd.) extraction and treatment with DNaseI (Life Technologies; Thermo Fisher Scientific, Inc.). Complementary DNA synthesis was performed with PrimeScript RT reagent kit (Takara Biotechnology Co., Ltd.) according to the manufacturer's instructions. qPCR was performed in technical triplicates using the Takara-Real Time PCR SYBR® Premix Ex Taq™ kit (Takara Biotechnology Co., Ltd.) and a relative standard curve method was used for quantification (LightCycler480; Roche Diagnostics, Basel, Switzerland) (22). Expression was calculated by normalization to the housekeeping gene β-actin.
The sequences of the primers used were as follows: Integrin subunit β6 (ITGB6): 5′-CTGCTTTGCCTGTTCTTTCTATTTC-3′ (forward) and 5′-GTTTCTGCACCTCCCAGGG-3′ (reverse); α-smooth muscle actin (α-SMA): 5′-GGCTCTGGGCTCTGTAAGG-3′ (forward) and 5′-CTCTTGCTCTGGGCTTCATC-3′ (reverse); β-actin: 5′-TGTTACCAACTGGGACGACA-3′ (forward) and 5′-GGGGTGTTGAAGGTCTCAAA-3′ (reverse) (23); collagen I: 5′-CCCAGAACATCACATATCAC-3′ (forward) and 5′-CAAGAGGAACACATATGGAG-3′ (reverse) (24); tissue inhibitor of metalloproteinase 1 (TIMP1): 5′-CTGTTGTTGCTGTGGCTGATA-3′ (forward) and 5′-CCGTCCACAAGCAATGAG-3′ (reverse) (24); integrin αVβ6: 5′-TCCAAGTGCGGCAGGTGG-3′ (forward) and 5′-CAGACTGTAGCCTGCATGATGG-3′ (reverse); matrix metalloproteinase (MMP) 2; MMP2: 5′-CAAGTTCCCCGGCGATGTC-3′ (forward) and 5′-TTCTGGTCAAGGTCACCTGTC-3′ (reverse) (23); MMP9: 5′-CTGGACAGCCAGACACTAAAG-3′ (forward) and 5′-CTCGCGGCAAGTCTTCAGAG-3′ (reverse) (23). The reaction conditions of qPCR were applied according to the manufacturer's instructions: 5 min at 93°C, followed by 45 cycles of 95°C for 10 sec, 60°C for 20 sec and 72°C for 30 sec.
Western blotting
LX-2 Cells were lysed on ice for 5 min with a lysis buffer containing 2% phosphatase inhibitor and proteinase inhibitor (Nanjing KeyGen Biotech Co., Ltd., Nanjing, China). The supernatant was obtained following centrifugation at 3,000 × g for 5 min at 4°C, and the protein concentration was determined with a BCA Protein Assay kit (Nanjing KeyGen Biotech Co., Ltd.) Equal amounts (30 µg/well) of protein were separated on 8–12% SDS-polyacrylamide gels and transferred to PVDF membranes. Non-specific binding sites were blocked with 5% non-fat milk for 1 h at room temperature. Membranes were incubated overnight at 4°C with a 1:1,000 dilution of the primary antibodies, washed three times for 5 min in PBS-Tween-20 and incubated for 1 h at room temperature with 1:5,000 dilution of anti-rabbit or anti-mouse IgG HRP-conjugated secondary antibodies (cat. no. 689202; Biolegend, Inc.). The immunoreactive bands were visualized using an ECL reagent (Santa Cruz Biotechnology, Inc., Dallas, TX, USA), according to the manufacturer's protocol. Primary antibodies were as follows: Anti-integrin αVβ6 (cat. no. ab97588; Abcam, Cambridge, MA, USA), anti-GAPDH (cat. no. KGAA002-2; Nanjing KeyGen Biotech Co., Ltd.), anti-α-SMA (cat. no. G6669; Sigma-Aldrich; Merck KGaA), collagen type I antibody (cat. no. 600-402-103; Rockland, Limerick, PA, USA), TIMP1 (cat. no. 8946), MMP2 (cat. no. 87809), MMP9 (cat. no. 13667), NF-κB: p65 (cat. no. 8242), p50 (cat. no. 3035) (all Cell Signaling Technology, Inc., Danvers, MA, USA) and IL-32γ (cat. no. 513501; Biolegend, Inc.). IL-32γ proteins (RD), cDNA 3.1, p50 or p65 expressing plasmids (pCMV-p50, pCMV-p65) and mock plasmid (pCMV-tag2) were kindly provided by Professor Guanxin Shen (Department of Immunology, Tongji Medical College, Huazhong University of Science and Technology). IL-32γ plasmid and the inhibitor of nuclear factor-κB, SN50, were kindly provided by Dr Xingdong Cai (Department of Respiratory, the First Affiliated Hospital, Jinan University, Guangzhou, China).
Viability assay
LX-2 cells were seeded into a 96-well plate at a density of 2,000 cells/well in RPMI-1640 containing 10% FBS, 100 µg/ml penicillin and 100 µg/ml streptomycin at 37°C in a 5% CO2 incubator, and deprived of serum for 24 h prior to the experiment. LX-2 cells were treated with IL-32γ at different concentrations (0, 5, 10, and 20 ng/ml). For every well containing 200 µl culture medium, 20 µl cell counting kit 8 solution (Dojindo Molecular Technologies, Inc., Kumamoto, Japan) was added. After 1 h incubation at 37°C, the optical density value of each well was measured at 450 nm. A group of wells without seeded cells served as a blank control group. Furthermore, each group included three repeated wells. The experiment was performed for 5 days and the proliferation of cells was observed at a certain time point every day.
Statistical analysis
Data were expressed as the mean ± standard deviation of three experiments. Statistical analysis data were analyzed using the Statistical Package for Social Sciences (SPSS) software (version 16.0; SPSS, Inc., Chicago, IL, USA). P<0.05 was determined to indicate a statistically significant difference.
Results
IL-32γactivates HSCs
In order to address whether IL-32 could active LX-2 cells, LX-2 cells were treated with IL-32γ at different concentrations, (0, 5, 10, and 20 ng/ml). After 48 h, LX-2 cell viability was detected using the CCK-8 kit. Compared with the control group, LX-2 cells treated with 20 ng/ml IL-32γ had a significantly faster growth rate (Fig. 1A). To further evaluate LX-2 cell activation, levels of α-SMA [a marker of HSC activation (25)], collagen I (representing ECM deposition), TIMP1 (preventing the degradation of ECM via inhibiting metalloproteinase), MMP2 (important factors for normal tissue remodeling) and MMP9 (important factors for normal tissue remodeling) mRNA and protein were detected. Results from RT-qPCR indicated that following LX-2 stimulation with IL-32γ, α-SMA (Fig. 1B), collagen I (Fig. 1C), TIMP1 (Fig. 1D), MMP2 (Fig. 1E) and MMP9 (Fig. 1F) mRNA expression increased compared with the control group (P<0.01). Results from western blot indicated that following LX-2 stimulation with IL-32γ, α-SMA, collagen I, TIMP1, MMP2, and MMP9 protein levels also increased compared with the control group (Fig. 1G). These results indicated that IL-32γ could activate HSCs.
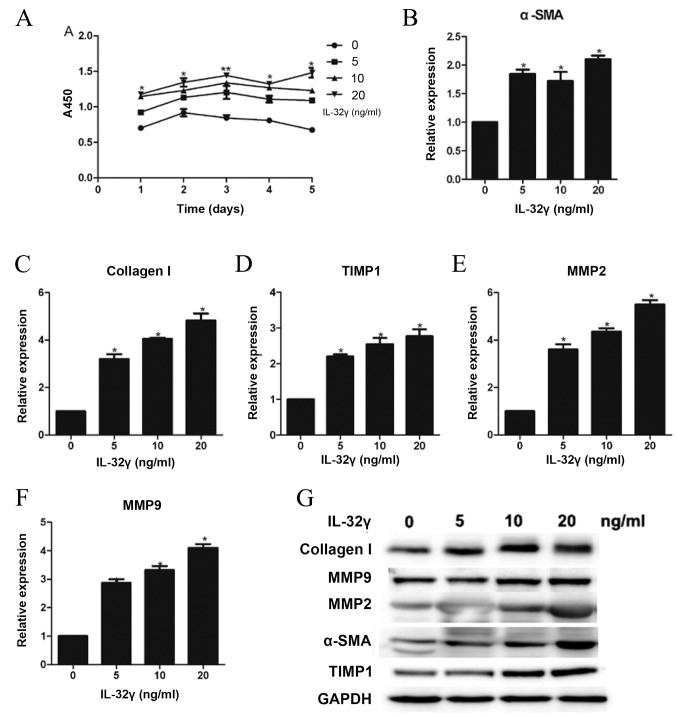
Effect of different concentrations (0, 5, 10 and 20 ng/ml) of IL-32γ on LX-2 activation phenotypes. (A) Growth curves of LX-2 demonstrated that upregulation of IL-32γ promoted proliferation of LX-2. (B) Reverse transcription-quantitative polymerase chain reaction assessing mRNA levels of α-SMA, (C) collagen I, (D) TIMP1, (E) MMP2 and (F) MMP9, representing the activation level of LX-2. (G) Western blot analysis was used to measure collagen I, MMP9, MMP2, α-SMA, TIMP1 and GAPDH expression in whole-cell extracts. Data are presented as the mean ± standard deviation of three experiments. *P<0.01 and **P<0.05 vs. 0 ng/ml IL-32γ. IL-32γ, interleukin-32γ; α-SMA, α-smooth muscle actin; TIMP1, tissue inhibitor of metalloproteinase 1; MMP, matrix metalloproteinases; GAPDH, glyceraldehyde 3-phosphate dehydrogenase; RT-qPCR, reverse transcription-quantitative polymerase chain reaction.
IL-32γ promotes integrin αvβ6 expression
LX-2 cells were treated with IL-32γ at different concentrations, (0, 5, 10 and 20 ng/ml; Fig. 2). Integrin αvβ6 expression significantly increased during LX-2 activation by IL-32γ compared with the control group (Fig. 2A and B; P<0.01). These data indicated that IL-32γ could promote integrin αvβ6 expression in HSCs.
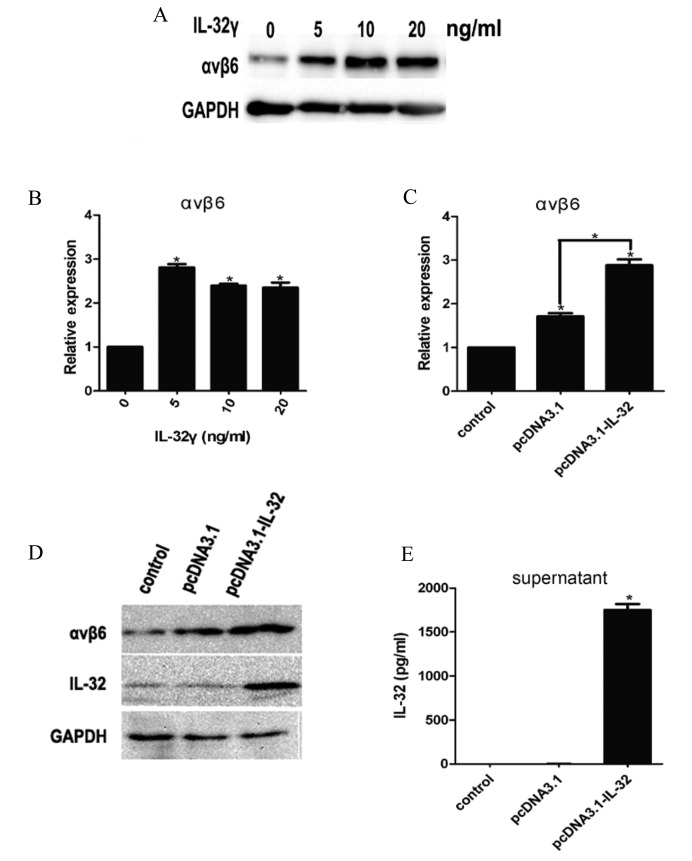
IL-32γ induces integrin αvβ6 expression. (A and B) Different concentrations of IL-32γ (0, 5, 10 and 20 ng/ml) were added to the LX-2 culture medium. (C and D) IL-32γ stably expressing cell lines pcDNA3.1-IL-32 or mock plasmids pcDNA3.1 cell line culture supernatant were added to the LX-2 culture medium. (A and D) Western blot analysis was used to determine the integrin αvβ6 protein expression of whole-cell extracts. (B and C) Reverse transcription quantitative-polymerase chain reaction measuring integrin αvβ6 mRNA levels. (E) Quantitative analysis of IL-32γ in corresponding cell line culture supernatant by ELISA. Results are representative of three independent experiments. Data are presented as the mean ± standard deviation of the mean. *P<0.01 vs. 0 ng/ml IL-32γ or control group. IL-32γ, interleukin-32γ; GAPDH, glyceraldehyde 3-phosphate dehydrogenase.
Subsequently, IL-32γ stable cell lines were constructed by transfecting HepG2 cells with the IL-32γ plasmid to investigate whether stably expressing IL-32γ HepG2 cell lines pcDNA3.1-IL-32 affects HSC activation. Cell culture supernatant of stably expressing IL-32γ HepG2 cell lines pcDNA3.1-IL-32 were used in the present study in order to treat HSCs. The results indicated that IL-32γ had been successfully introduced into the HepG2 cells (Fig. 2E). Integrin αvβ6 mRNA (Fig. 2C; P<0.01) and protein (Fig. 2D and E) levels were significantly enhanced compared with the control pcDNA3.1 groups.
NF-κB activation is required for IL-32γ-induced integrin αvβ6 expression
Subsequently, the mechanism by which IL-32γ promoted integrin αvβ6 expression was assessed. It was determined that the expression of p65 and p50 proteins increased following LX-2 activation by IL-32γ (Fig. 3A), indicating that IL-32γ may lead to NF-κB activation. To further address this possibility, LX-2 cells were co-transfected with p65 and/or p50 expressing plasmids (NF-κB subunit plasmids). Integrin αvβ6 expression was higher in the cells transfected with p65- or p50-expressing plasmids compared with the control group (Fig. 3B and C; P<0.01) and integrin αvβ6 expression was highest in LX-2 cells co-transfected with p65 and p50 expressing plasmids (Fig. 3B and C). Following treatment of IL-32γ-induced LX-2 cells with SN50 at different concentrations (0, 25, 50, 75 and 100 ng/ml), αvβ6 integrin expression gradually decreased in a dose-dependent manner compared with the control (Fig. 3D and E; P<0.01). These results clearly demonstrate that exogenous IL-32γ in LX-2 cells leads to activation of the NF-κB signaling transduction pathway, and that NF-κB activation is required for IL-32γ-induced integrin αvβ6 expression.
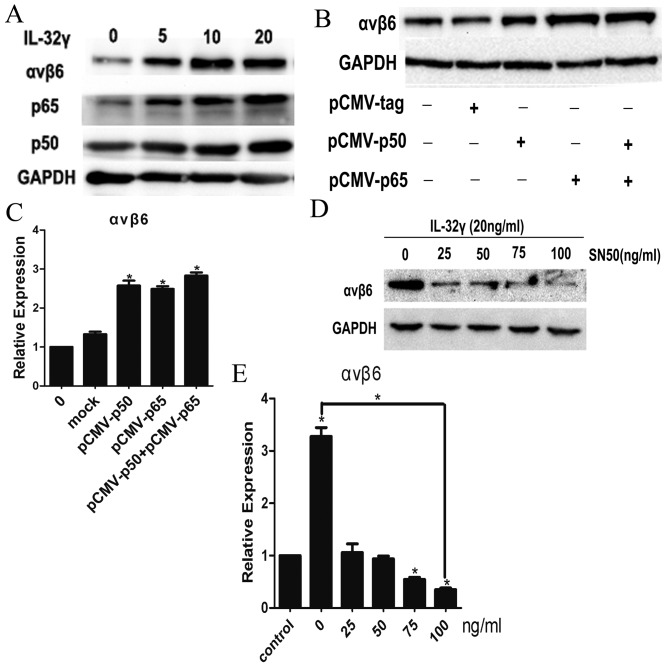
NF-κB activation is required for IL-32γ-induced integrin αvβ6 expression. Different concentrations of IL-32γ were added to the LX-2 culture medium and (A) western blot analysis was performed for integrin αvβ6, p65, p50 and GAPDH in whole-cell extracts from LX-2 cells. LX-2 cells were transfected with mock plasmids, p50 and/or p65 expression construct plasmids. (B) Integrin αvβ6 protein was measured by western blot analysis. (C) Integrin αvβ6 gene expression levels were measured by RT-qPCR. SN50 was used to treat LX-2 cells at different concentrations. After 48 h, relative integrin αvβ6 protein and gene expression level were determined by (D) western blot analysis and (E) RT-qPCR. Results are representative of three experiments. Data are represented as the mean ± standard deviation of the mean. *P<0.01 vs. control group. IL-32γ, interleukin-32γ; GAPDH, glyceraldehyde 3-phosphate dehydrogenase; RT-qPCR, reverse transcription-quantitative polymerase chain reaction; NF-κB, nuclear factor κB.
IL-32γ induces integrin αvβ6 promoter activity in a dose-dependent manner
In order to determine whether IL-32γ could induce αvβ6 integrin gene transcription through a promoter, a construction containing the sequence from −604 to +92 (including binding sites of NF-κB and relative to the transcriptional start site) of the 5′-flanking region of the human αvβ6 integrin gene was transfected into LX-2 cells. Subsequently, LX-2 cells were treated with IL-32γ at different concentrations (0, 5, 10 and 20 ng/ml). Following 48 h, luciferase activity was detected using a dual luciferase reporter assay system (Fig. 4). The results demonstrated that integrin αvβ6 promoter activity was significantly activated by IL-32γ compared with the control group (0 ng/ml IL-32γ) and the levels of luciferase activity increased as the concentration of IL-32γ increased (Fig. 4; P<0.01). The results indicated that IL-32γ is a direct regulator of integrin αvβ6 promoter that induces integrin αvβ6 expression by activating NF-κB.
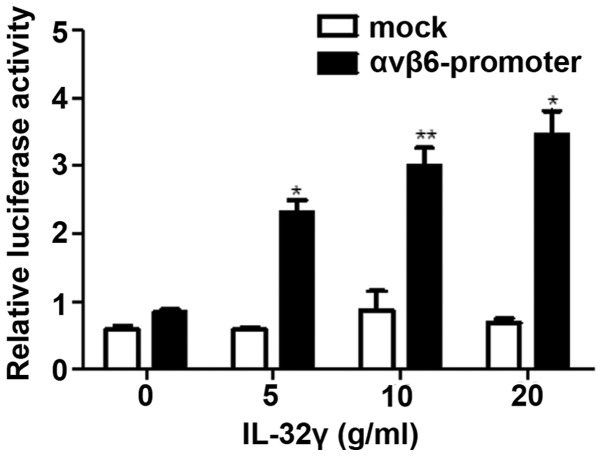
IL-32γ induces integrin αvβ6 promoter activity. Different concentrations of IL-32γ were added and co-transfected with mock plasmids or plasmids expressing αvβ6 integrin promoter luciferase reporter vector LX-2 cells culture medium. Following 48 h, relative luciferase activity was determined. Data are presented as the mean ± standard deviation of the mean. *P<0.01 and **P<0.05 vs. 0 ng/ml IL-32γ. IL-32γ, interleukin-32γ.
Discussion
Liver fibrosis is a reversible wound-healing response to either chronic or acute cellular injury and reflects a balance between scar formation and liver repair (26). HSCs, the major producers of the ECM, are the primary effector cells for the fibrotic liver (27) and activated HSCs may promote hepatic fibrogenesis (26). However, the precise mechanism of HSC activation remains unknown. It is accepted that numerous soluble factors, including cytokines, chemokines, growth factors and products of oxidative stress, may regulate the activation of HSCs (28). For example, OPN, an oxidant stress sensitive cytokine, contributes to transforming growth factor β1-mediated HSC activation.
The present study demonstrated that IL-32γ is capable of inducing HSC activation (Fig. 1). Therefore, it is presumed that IL-32 can promote liver fibrosis by inducing HSC activation. However, to the best of our knowledge, there have been no mechanistic studies on how IL-32γ induces HSC activation. HSCs express numerous integrins. Integrin αvβ6 is also known as the ‘epithelial specific cell surface receptor’ and can influence HSC activation and proliferation by binding to the relevant ligands. Silencing the integrin αv subunit expression using small interfering RNA or disengagement of integrin αvβ3 using echistatin or neutralizing antibodies inhibits HSC proliferation (14). Integrins are minimally expressed in normal adult tissues, but integrin expression increases in cancer cells, including pancreatic, cervical, lung and colon cancers (29). Integrin αvβ6 also contributes to the promotion of the metastatic potential and the survival of cancer cells in the liver (30). In the present report, it was determined that IL-32γ could significantly induce integrin αvβ6 expression in HSCs (Fig. 2). Therefore, it is assumed that IL-32 can stimulate the expression of integrin αvβ6 thus influencing HSC activation.
IL-32 and integrins both contain an RGD motif (9,17) and integrins are able to activate the FAK/ERK/NF-κB signaling pathway (12). NF-κB acts as a central link between hepatic injury, fibrosis and hepatocellular carcinoma, and the activation of NF-κB in HSCs appears to promote hepatic fibrosis (31). Furthermore, there are three binding sites of NF-κB in the integrin αvβ6 promoter region (at −406 to −392, at −139 to −125 and at +431 to +445). In the present study, it was determined that p65 and p50 expression increased following LX-2 activation induced by IL-32γ (Fig. 3A). Integrin αvβ6 expression increased following the transfection of LX-2 cells with the NF-κB expressing plasmids, p65 and p50 (Fig. 3B and C). SN50, an inhibitor of NF-κB, was able to inhibit integrin αvβ6 expression (Fig. 3D and E). Subsequently, an integrin αvβ6 promoter expression vector was constructed and it was validated that IL-32γ induced integrin αvβ6 expression by activating the NF-κB signaling transduction pathway (Fig. 4). These results indicate that IL-32γ can promote integrin αvβ6 expression by activating NF-κB.
The present study demonstrated that IL-32γ can induce integrin αvβ6 expression and activate HSCs. However, the cytokine network cascade reaction of hepatic fibrosis and detailed signal transduction pathway following integrin αvβ6 expression remains unknown and requires further investigation. Future studies should identify the detailed signal transduction pathway in liver cells and investigate its role.
In summary, the present study identified the profibrogenic molecule mechanisms of IL-32. Following different types of liver injury, IL-32γ expression was increased. It was determined that increased levels of IL-32γ protein may activate the integrin αvβ6 promoter and induce integrin αvβ6 expression, thus activating HSCs. Hepatic fibrosis was achieved and it was concluded that the identification of the mechanism and mediators involved in the profibrogenic actions of IL-32γ may allow the development of novel strategies for targeted therapy.
Acknowledgements
The present study was supported by research grants from the National Natural Science Foundation of China (grant nos. 81401306, 81401191 and 81102531).
References
Articles from Experimental and Therapeutic Medicine are provided here courtesy of Spandidos Publications
Full text links
Read article at publisher's site: https://doi.org/10.3892/etm.2017.4956
Read article for free, from open access legal sources, via Unpaywall:
https://www.spandidos-publications.com/etm/14/4/3880/download
Citations & impact
Impact metrics
Citations of article over time
Smart citations by scite.ai
Explore citation contexts and check if this article has been
supported or disputed.
https://scite.ai/reports/10.3892/etm.2017.4956
Article citations
Role of interleukin‑32 in cancer progression (Review).
Oncol Lett, 27(2):54, 12 Dec 2023
Cited by: 0 articles | PMID: 38192653 | PMCID: PMC10773214
Review Free full text in Europe PMC
Wild Bitter Melon Extract Regulates LPS-Induced Hepatic Stellate Cell Activation, Inflammation, Endoplasmic Reticulum Stress, and Ferroptosis.
Evid Based Complement Alternat Med, 2021:6671129, 22 Jun 2021
Cited by: 15 articles | PMID: 34239589 | PMCID: PMC8241502
Effect of NLRC5 on activation and reversion of hepatic stellate cells by regulating the nuclear factor-κB signaling pathway.
World J Gastroenterol, 25(24):3044-3055, 01 Jun 2019
Cited by: 8 articles | PMID: 31293340 | PMCID: PMC6603813
Role of Metabolism in Hepatic Stellate Cell Activation and Fibrogenesis.
Front Cell Dev Biol, 6:150, 12 Nov 2018
Cited by: 44 articles | PMID: 30483502 | PMCID: PMC6240744
The Active Components of Fuzheng Huayu Formula and Their Potential Mechanism of Action in Inhibiting the Hepatic Stellate Cells Viability - A Network Pharmacology and Transcriptomics Approach.
Front Pharmacol, 9:525, 24 May 2018
Cited by: 12 articles | PMID: 29881350 | PMCID: PMC5976863
Data
Data behind the article
This data has been text mined from the article, or deposited into data resources.
BioStudies: supplemental material and supporting data
Genes & Proteins
- (1 citation) UniProt - P24001
Similar Articles
To arrive at the top five similar articles we use a word-weighted algorithm to compare words from the Title and Abstract of each citation.
2,3,7,8-Tetrachlorodibenzo-p-dioxin (TCDD) induces hepatic stellate cell (HSC) activation and liver fibrosis in C57BL6 mouse via activating Akt and NF-κB signaling pathways.
Toxicol Lett, 273:10-19, 14 Mar 2017
Cited by: 13 articles | PMID: 28302560
Inhibition of skin carcinogenesis by suppression of NF-κB dependent ITGAV and TIMP-1 expression in IL-32γ overexpressed condition.
J Exp Clin Cancer Res, 37(1):293, 28 Nov 2018
Cited by: 14 articles | PMID: 30486830 | PMCID: PMC6263970
Interleukin-32γ attenuates ethanol-induced liver injury by the inhibition of cytochrome P450 2E1 expression and inflammatory responses.
Clin Sci (Lond), 128(10):695-706, 01 May 2015
Cited by: 8 articles | PMID: 25583360
Liuweiwuling tablets attenuate BDL-induced hepatic fibrosis via modulation of TGF-β/Smad and NF-κB signaling pathways.
J Ethnopharmacol, 210:232-241, 31 Aug 2017
Cited by: 13 articles | PMID: 28864168