Abstract
Free full text

Senescence and aging: Causes, consequences, and therapeutic avenues
Abstract
Aging is the major risk factor for cancer, cardiovascular disease, diabetes, and neurodegenerative disorders. Although we are far from understanding the biological basis of aging, research suggests that targeting the aging process itself could ameliorate many age-related pathologies. Senescence is a cellular response characterized by a stable growth arrest and other phenotypic alterations that include a proinflammatory secretome. Senescence plays roles in normal development, maintains tissue homeostasis, and limits tumor progression. However, senescence has also been implicated as a major cause of age-related disease. In this regard, recent experimental evidence has shown that the genetic or pharmacological ablation of senescent cells extends life span and improves health span. Here, we review the cellular and molecular links between cellular senescence and aging and discuss the novel therapeutic avenues that this connection opens.
Introduction
Aging is characterized by a gradual functional decline. In mammals, aging occurs heterogeneously across multiple organ systems, causing a progressive deterioration that eventually results in tissue dysfunction. Consequently, age is a risk factor for many diseases (Niccoli and Partridge, 2012), such as cardiovascular disease (North and Sinclair, 2012), dementia (Querfurth and LaFerla, 2010), osteoporosis (Raisz, 1988), osteoarthritis (Raisz, 1988), cancer (de Magalhães, 2013), type 2 diabetes (Gunasekaran and Gannon, 2011), idiopathic pulmonary fibrosis (IPF; Nalysnyk et al., 2012), and glaucoma (Kwon et al., 2009). Despite these links with human pathology, our understanding of the aging process remains limited. Although its biological causes remain largely unknown, studies in the past few decades have identified common cellular and molecular traits associated with aging (López-Otín et al., 2013). The identification of so-called aging hallmarks has helped to conceptualize aging research and has hinted at the tantalizing prospect of delaying multiple age-related diseases by targeting the aging process.
Aging hallmarks can be divided into three categories: (1) primary, or the causes of age-associated damage; (2) antagonistic, or the responses to the damage; and (3) integrative, or the consequences of the responses and culprits of the aging phenotype. Senescence, a cellular response that limits the proliferation of aged or damaged cells (Muñoz-Espín et al., 2013; Muñoz-Espín and Serrano, 2014), belongs to the antagonistic class (Fig. 1). Although senescence plays physiological roles during normal development and it is needed for tissue homeostasis, senescence constitutes a stress response triggered by insults associated with aging such as genomic instability and telomere attrition, which are primary aging hallmarks themselves. There is also an intimate link between senescence and the other antagonistic hallmarks of aging. For example, senescent cells display decreased mitophagy, resulting in an “old,” defective mitochondrial network that may contribute to metabolic dysfunction in age (Sun et al., 2016).
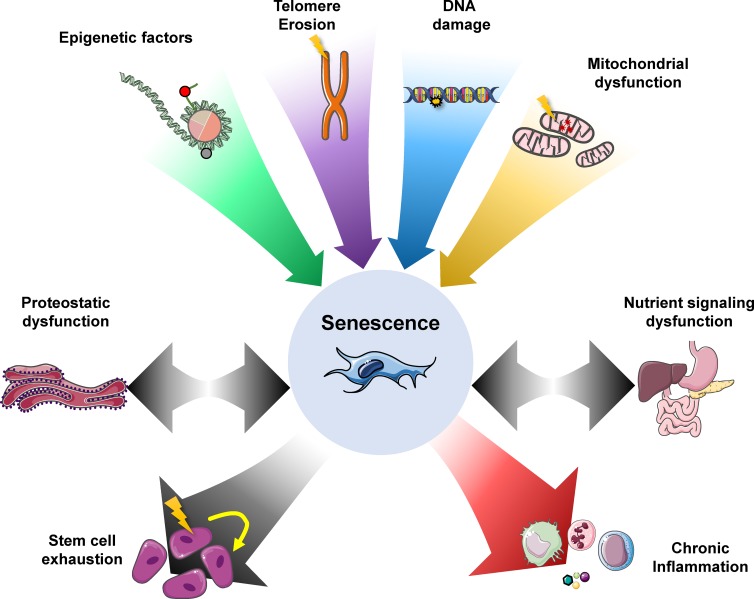
Senescence as a central hallmark of aging. Telomere damage, epigenetic dysregulation, DNA damage, and mitochondrial dysfunction are primary drivers of damage in aging. Several of these drivers of damage can induce senescence. Senescence can in turn drive the consequential aging hallmarks in response to damage: stem cell exhaustion and chronic inflammation. Other responses to damage, such as proteostatic dysfunction and nutrient signaling disruption, are also integrally linked with the senescence response. Adapted from López-Otín et al. (2013).
Senescence also influences the integrative aging hallmarks. Somatic multipotent stem cells facilitate tissue homeostasis; for example, hematopoietic stem cells (HSCs) renew the blood system. Stem cell exhaustion occurs with age, and the consequent decline in stem cell functionality and their capacity for renewal leads to tissue deterioration. For example, HSCs display a decreased success rate of transplantation when isolated from elderly patients (Kollman et al., 2001). This decline correlates with increased numbers of senescent HSCs (Chang et al., 2016) and diminished immunity (Geiger and Van Zant, 2002), decreased numbers of naive B and T cells (Min et al., 2005), and reduced natural killer cell activity (Mocchegiani and Malavolta, 2004). Somatic stem cell decline is not limited to high-turnover tissues. Neural stem cells (NSCs) experience reduced functionality, with limited neurogenesis capacity with age. This is marked by a twofold reduction in NSC numbers and a decreased proliferation, which correlates with increased expression of senescence markers in the regions where NSCs reside (Molofsky et al., 2006). Mesenchymal stem cells (Raggi and Berardi, 2012) and their descendants, satellite cells (Shefer et al., 2006; Lavasani et al., 2012; Sousa-Victor et al., 2014), chondrocytes (Loeser, 2009), adipocytes (Tchkonia et al., 2010), and osteoclasts (Chung et al., 2014), also display a reduced ability to self-renew with age that correlates with increased levels of senescence markers. This may have an impact in age-associated pathologies such as sarcopenia, cachexia, osteoporosis, and osteoarthritis (Fried et al., 2001).
Altered intracellular communication is another of the integrative hallmarks of aging. In particular, chronic low-level inflammation is a serious complicating factor for many diseases in which risk increases with age (López-Otín et al., 2013; Franceschi and Campisi, 2014). This detrimental role of inflammation is supported by inflammatory markers such as interleukin-1 (IL-1) and IL-6 acting as prognostic markers for diseases such as type 2 diabetes (Dandona et al., 2004), atherosclerosis (Libby, 2002), and breakdown in stem cell function (Doles et al., 2012; Pietras et al., 2016). Inflammatory responses are one of the major extrinsic effects of senescent cells (Coppé et al., 2010), which suggests that there is a link between senescence and altered intracellular communication. Aging influences a broad range of disease etiologies. Therefore, targeting the underlying aging machinery may provide broad-spectrum protection against many pathologies.
What is senescence?
Senescence is cellular program that induces a stable growth arrest accompanied by distinct phenotypic alterations, including chromatin remodeling, metabolic reprogramming, increased autophagy, and the implementation of a complex proinflammatory secretome (Kuilman et al., 2010; Salama et al., 2014). These complex changes to the cell largely serve to implement various aspects of senescence such as growth arrest and the senescence secretome. Despite the many facets of senescence, stable growth arrest is its defining characteristic. A permanent arrest is effective to ensure that damaged or transformed cells do not perpetuate their genomes. This growth arrest is implemented by the activation of p16INK4a/Rb and p53/p21CIP1 tumor suppressor networks (Fig. 2).
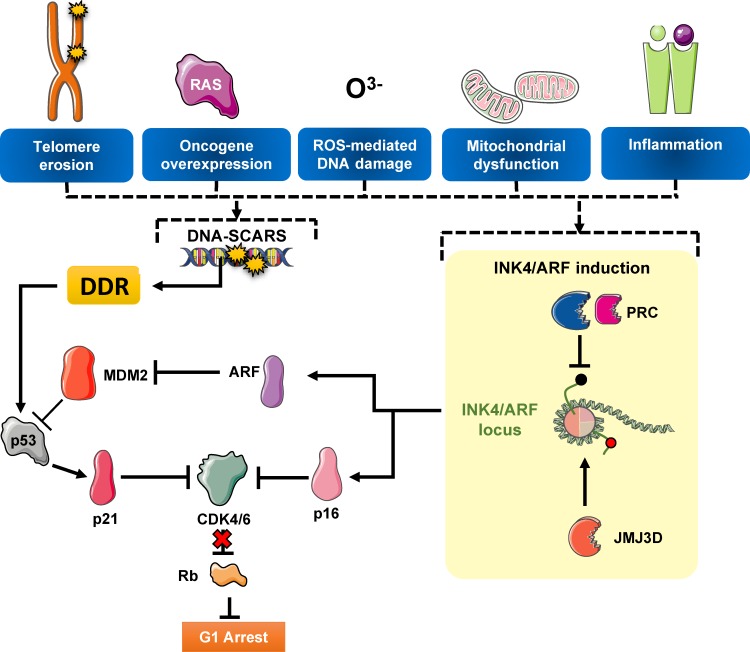
Pathways regulating senescence-mediated arrest. The senescence growth arrest is regulated through two main pathways, p16INK4a/Rb and p53/p21CIP1, both which converge on repression of CDK4/6. The INK4A/ARF locus is normally silenced by Polycomb repressive complexes (PRCs) and becomes activated during senescence. The p53/p21CIP1 pathway is activated downstream of the DNA damage response (DDR) from repair-resistant DNA segments with chromatin alterations reinforcing senescence (DNA-SCARS).
Historically, senescence was first identified by Hayflick and Moorhead (1961) during serial passage of human fibroblasts. The limit to proliferation that senescence imposes was hypothesized as a barrier to cancer initiation. Senescence is indeed a powerful mechanism of tumor suppression (Collado et al., 2007; Hanahan and Weinberg, 2011). Senescence has also physiological roles during normal development (Muñoz-Espín et al., 2013; Storer et al., 2013), acting in concert with apoptosis to facilitate embryonic morphogenesis. In adult tissues, senescence is triggered primarily as a response to damage, allowing for suppression of potentially dysfunctional, transformed, or aged cells. The aberrant accumulation of senescent cells with age results in potential detrimental effects. In balance, although senescence is a biologically necessary process, it may come at a cost. The early research of Hayflick and Moorhead (1961) hinted at a relationship between senescence and aging, but the consequent discovery that senescent cells accumulate in aged tissues has substantiated the hypothesis that senescence itself can drive aging.
Factors driving senescence
Telomere damage driving senescence in aging
In adult tissues, senescence is engaged in response to different types of damage. One of the insults causing senescence is damage of the telomeres, highly repetitive DNA structures located at the end of chromosomes. Telomeres are protected by a multiprotein complex known as shelterin. By coating the telomere, shelterin prevents the activation of a DNA damage response, thereby preventing end-to-end chromosome fusions that would result in a telomere crisis (Palm and de Lange, 2008). Moreover, cells lacking shelterin components, such as POT1 or TRF2, suffer an aberrant DNA damage response and premature induction of senescence (Denchi and de Lange, 2007). The end-replication problem is a consequence of the inability of DNA polymerases to synthesize DNA without a template, which occurs at telomeres. This results in telomeres that shorten progressively with each cell cycle division. Embryonic tissues circumvent this erosion by expressing telomerase, a ribonucleoprotein complex that serves to concatenate DNA to the ends of chromosomes, thus providing a template for DNA synthesis (Nandakumar and Cech, 2013). Repeated cell division in adult tissues that lack telomerase, however, results in progressive erosion of DNA, reduced shelterin binding, and senescence. As an organism ages, cells accumulate more divisions. This results in increased telomere erosion and senescence. But the extent to which telomere erosion drives senescence during aging and contributes to the aging process itself remains unknown.
Supporting the causative role of telomere erosion in aging, deletion of telomerase in mice eventually results in premature aging (Lee et al., 1998). This phenotype can be rescued by transient activation of telomerase reverse transcription expression in mice using a telomerase reverse transcription estrogen receptor construct. Cells isolated from these mice proliferate normally in vitro, and deterioration in multiple tissues is reduced (Jaskelioff et al., 2011). This evidence correlates with studies showing that fibroblasts or T cells derived from centenarians reset their telomeres, which results in rejuvenation and sustained proliferation (Lapasset et al., 2011). Similarly, stimulation of T cells derived from serially transplanted HSCs results in telomerase expression and rejuvenation (Allsopp et al., 2002). Shortened telomeres are associated with many pathologies such as liver cirrhosis (Rudolph, 2000) and correlate with an increase in mortality in people older than 60 years (Cawthon et al., 2003). Correlative evidence supports telomere erosion as a major driver of aging decline, yet this is challenged by mammals such as laboratory mice (Mus musculus), whose telomeres do not reach a critical limit during normal aging. Telomere length is also not predictive of aging deterioration in mice (Rudolph et al., 1999), highlighting that alternative factors could also drive aging.
Metabolic dysfunction as a driver of senescence
Several lines of evidence suggest that aging is the result of a complex amalgam of damages such as metabolic and proteostatic dysfunction (López-Otín et al., 2013). Metabolic dysfunction relates to aging at the organismal and molecular level. Multiple studies have demonstrated that caloric restriction can retard the aging decline (Mitchell et al., 2016). Molecularly, pathways fine-tuning metabolic regulation, such as the mTOR or insulin pathway, have also been linked to increased health span and life span (Selman et al., 2008; Harrison et al., 2009). mTORC1 integrates inputs from nutrient and growth signals to regulate general cellular processes such as protein and lipid synthesis, autophagy, and metabolism (Saxton and Sabatini, 2017). In this regard, mTOR is able to regulate the senescence-associated secretory phenotype (SASP), autophagy, and senescent growth arrest (Herranz et al., 2015; Laberge et al., 2015). The connection between autophagy and senescence is complex; although there is an increase in autophagy during senescence that serves to regulate SASP production (Narita et al., 2011), inhibition of autophagy can induce senescence through metabolic and proteostatic dysfunction (García-Prat et al., 2016), further emphasizing the intricate connection between metabolic stress and senescence in aging.
Sirtuins constitute another molecular link between metabolism and senescence. Sirtuins are ribosyltransferases with a wide array of functions, such as metabolism regulation and DNA repair (Houtkooper et al., 2012). Their role in senescence is antagonistic; SIRT1 deacetylates p53, promotes its degradation (Solomon et al., 2006), and facilitates senescence bypass, whereas SIRT6 deacetylates H3K18 to prevent mitotic errors and suppress senescence (Tasselli et al., 2016).
In addition to these forms of damage, general stress is sensed by other mechanisms such as activation of MAPK p38 or induction of p16INK4a. These pathways are up-regulated in response to oxidative stress, DNA damage, telomere attrition, or oncogene activation. Substantiating their role in aging, activation of MAPK p38 or induction of p16INK4a limits the proliferative potential of HSCs and yields proaging phenotypes (Ito et al., 2006; Baker et al., 2016). Overall, it is likely that the accumulation of senescent cells during aging reflects a gradual increase of different types of damage in different tissues.
Pathways regulating the senescence growth arrest
Despite the multifaceted nature of senescence, the induction of stable growth arrest is the defining characteristic of senescence. Moreover, stable arrest is paramount to halt the propagation of dysfunctional cells. Two tumor suppressor pathways, p53 and the p16/Rb, are responsible for the implementation of this growth arrest.
p53 and senescence
Senescence inducers such as telomeric attrition and oncogenic or oxidative stress cause DNA damage. DNA damage results in increased deposition of γH2Ax and 53BP1 in chromatin that in turn activates a kinase cascade involving first ATM and ATR and then CHK1 and CHK2, eventually resulting in p53 activation (d’Adda di Fagagna, 2008; Fumagalli et al., 2012). p53 induces transcription of the cyclin-dependent kinase inhibitor p21CIP1. In turn, p21CIP1 blocks CDK4/6 activity, resulting in hypophosphorylated Rb and cell cycle exit (d’Adda di Fagagna, 2008). Although transient increases in p53 levels can enact a quiescent state and activate DNA repair processes, during senescence, there is a sustained induction of p53 (Salama et al., 2014; Kruiswijk et al., 2015). This is a result of damage occurring in repair-resistant regions of the genome known as DNA segments with chromatin alterations reinforcing senescence, such as telomeres (Rodier et al., 2011; Fumagalli et al., 2012), that allow for a permanent arrest of the cell cycle by persistent induction of p21cip1. Given the key roles of p53, additional regulatory layers exist. For example, the induction of ARF, a product of the INK4/ARF locus, sequesters the ubiquitin ligase MDM2, contributing to increased levels of p53. Recently, the interaction between Forkhead box protein O4 (FOXO4) and p53 has been shown to play an important role in modulating p53 localization and transcriptional activity during senescence (Baar et al., 2017). Interestingly FOXO transcription factors regulate aging, with FOXO activity in Drosophila melanogaster leading to delayed aging in response to disrupted protein homeostasis and oxidative stress (Demontis and Perrimon, 2010).
The INK4/ARF locus in senescence
Three tumor suppressors reside in the INK4/ARF locus: p16INK4a and ARF, which are both encoded by the CDKN2A gene, and p15INK4b, which is encoded by CDKN2B. Two of these, p15INK4b and p16INK4a, are CDKIs, like p21CIP1, that affect the cell cycle by binding and inhibiting CDK4 and CDK6. In contrast, ARF inhibits MDM2, thereby allowing cross talk with the p53/p21CIP1 pathways. Conversely, p53 can regulate expression of ARF through a negative feedback loop, as demonstrated by elevated ARF expression in p53−/− mouse embryonic fibroblasts (Harris and Levine, 2005).
Given this unusual concentration of three tumor suppressors in barely 35 kb, this locus plays a key regulatory role and is frequently mutated in cancer (Gil and Peters, 2006; Kim and Sharpless, 2006). Genome-wide association studies have also identified various genomic variants occurring at the INK4/ARF locus as major risk factors for atherosclerosis, stroke, and diabetes, among other pathologies (Jeck et al., 2012). However, most of these are found in noncoding regions, and the precise mechanism of action is unclear. The INK4/ARF locus behaves as a senescence sensor. In young, normal cells, the INK4/ARF locus is epigenetically silenced through deposition of repressive H3K27me3 marks (Bracken et al., 2007). H3K27 methylation is controlled by Polycomb repressive complexes (PRC2 and PRC1). Disrupting PRC1 or PRC2 activity by depleting the expression of some of their components, such as BMI1, CBX7, or EZH2, derepresses p16INK4a and induces senescence (Jacobs et al., 1999; Gil et al., 2004; Bracken et al., 2007). There is still debate over how Polycomb is recruited to the INK4/ARF locus. It has been proposed that a long noncoding RNA, ANRIL, divergently transcribed from the INK4/ARF locus (Yap et al., 2010; Kotake et al., 2011), and transcription factors such as those of the homeobox family can contribute to recruiting PRCs (Martin et al., 2013).
Conversely, during senescence, the H3K27 histone demethylase JMJD3 plays a role in removing the repressive marks around the INK4/ARF locus, facilitating its induction (Agger et al., 2009; Barradas et al., 2009). INK4/ARF induction can be observed in tissues during natural aging (Krishnamurthy et al., 2004; Burd et al., 2013). In particular, p16INK4a is considered an aging biomarker. With exceptions (such as during senescence-induced during development), p16INK4a is also one of the best markers of senescence. An analysis of the pathways regulating p16INK4a shows coincidences with those controlling development. This has been argued to formulate the theory that aging might be driven by gradual functional decay of developmental pathways (Martin et al., 2014).
The SASP
Besides growth arrest, the production of a complex mixture of secreted factors, termed the SASP or senescence-messaging secretome, is the most relevant phenotypic program implemented in senescent cells. Senescent cells secrete hundreds of factors that include proinflammatory cytokines, chemokines, growth factors, and proteases (Kuilman and Peeper, 2009; Coppé et al., 2010).
Regulation of the SASP
The specific combination of secreted factors is thought to depend on the cell type and the senescent inducer. However, many of the key effectors of the SASP and its regulatory mechanism seemed to be shared. Nuclear factor κB (NF-κB) and CCAAT/enhancer–binding protein beta are the key transcriptional SASP regulators (Acosta et al., 2008; Kuilman et al., 2008). DNA damage (Rodier et al., 2009), p38α MAPK (Freund et al., 2011), mTOR (Herranz et al., 2015; Laberge et al., 2015), mixed lineage leukemia 1 (Capell et al., 2016), and GATA4 (Kang et al., 2015) are also able to regulate the SASP. Recently, sensing of cytoplasmic chromatin by the cGAS/STING pathway has been suggested as a trigger for SASP induction (Dou et al., 2017; Glück et al., 2017). There are additional layers of SASP regulation. For example, mTOR controls IL-1α translation to regulate the SASP (Laberge et al., 2015). In addition, mTOR indirectly regulates the activity of ZFP36L1, an RNA-binding protein that binds to AU-rich elements in the 5′-end of inflammatory transcripts, targeting them for degradation (Herranz et al., 2015). There is also a global remodeling of enhancers in senescent cells, and the recruitment of BRD4 to superenhancers adjacent to SASP genes is needed for their induction (Tasdemir et al., 2016).
The complex composition of the SASP means that different subsets of the SASP, such as the proinflammatory and TGF-β secretomes, can be regulated independently. The proinflammatory arm of the SASP is regulated by IL-1 signaling (Acosta et al., 2013). IL-1α partially recapitulates the inflammatory SASP in vitro, and inhibiting the NLRP3 inflammasome, which processes IL-1β, can blunt the SASP (Acosta et al., 2013). Conversely, the juxtacrine Notch signaling pathway promotes the secretion of a TGF-β–enriched secretome (Hoare et al., 2016).
Functions of the SASP
The SASP is responsible for many of the positive and negative functions attributed to senescent cells (Fig. 3) (Kuilman and Peeper, 2009). One of the major functions of the SASP is to recruit the immune system to eliminate senescent cells. The SASP mediates the activation and recruitment of both adaptive and innate immune cells (Xue et al., 2007; Kang et al., 2011). In general terms, the effects are positive. During tumor initiation, SASP-mediated immune recruitment acts as an extrinsic tumor suppressor mechanism (Xue et al., 2007; Kang et al., 2011), and the recruitment of macrophages is a key step in fibrosis resolution (Krizhanovsky et al., 2008). In contrast, SASP-mediated recruitment of immature myeloid cells has immune suppressive effects on prostate and liver cancer (Di Mitri et al., 2014; Eggert et al., 2016). In addition, the SASP can stimulate tumorigenesis by promoting angiogenesis (e.g., via VEGF and CCL5; Coppé et al., 2006; Eyman et al., 2009) or tumor growth (e.g., via GROα and Osteopontin; Krtolica et al., 2001; Pazolli et al., 2009), among other mechanisms. Specific components of the SASP have other physiological functions, such as contributing to fibrotic tissue remodeling, whereby matrix metalloproteinases (MMPs) contribute to degrade fibrotic plaques in the ECM that may be beneficial in the context of liver fibrosis and wound healing (Krizhanovsky et al., 2008; Demaria et al., 2014).
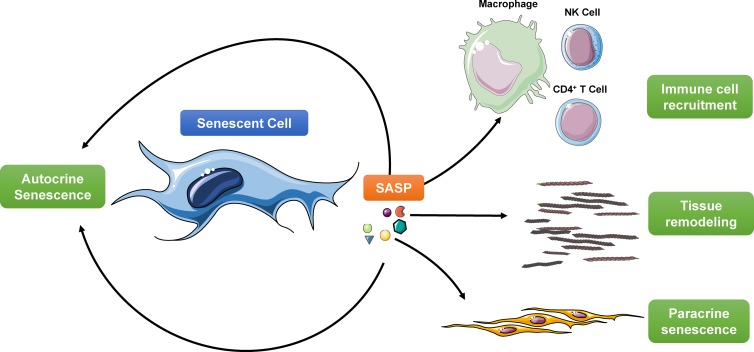
Functions of the SASP. The SASP mediates many of the cell-extrinsic functions of senescent cells. Among those it reinforces several aspects of senescence including growth arrest and the SASP itself via an autocrine loop. The SASP also recruits immune cells, such as macrophages, neutrophils, and natural killer (NK) cells to phagocytose and eliminate the senescent cell. Secretion of MMPs and factors such as VEGF can remodel the surrounding tissue, inducing angiogenesis and reducing fibrosis. Finally, secretion of molecules such as TGF-β can spread the senescence phenotype in a paracrine manner to surrounding cells.
Recently, it has been postulated that senescent cells accumulating in response to tissue damage can also promote stemness and reprogramming (Ritschka et al., 2017). However, how this fits with the increased number of senescent cells but decreased stemness potential observed during aging is unclear. On the other hand, factors secreted by senescent cells can reinforce the senescent phenotype, potentially exacerbating senescence during aging. IL-8, GROα, IL-6, and IGBP-7 are among the specific SASP components reinforcing senescence (Acosta et al., 2008; Kuilman et al., 2008; Wajapeyee et al., 2008). Moreover, senescent cells can also induce a so-called paracrine senescence response (Acosta et al., 2013). This autocrine reinforcement or paracrine transmission of senescence could potentially explain some of the detrimental effects of aberrant accumulation of senescent cells during aging. During aging, the SASP is thought to be partially responsible for persistent chronic inflammation, also known as inflammaging, that contributes to multiple age-related phenotypes. This contribution of SASP in inflammaging is beginning to be investigated using senolytic models. The direct elimination of senescent cells in aged kidney (Baker et al., 2016; Baar et al., 2017), heart, spleen, lung, liver (Baker et al., 2016), and osteoarthritic knee (Jeon et al., 2017) reduced levels of IL-6 and IL-1β (both markers of chronic inflammation). It would be pertinent in future aging therapies to understand how specific aspects of the SASP contribute to the deterioration or protection of tissues.
Senescence: Bystander or participant in age-related pathologies?
Although the contribution of senescence to aging has been long suspected, only recently has the connection been confirmed. This has been made possible by the use of molecular biomarkers of senescence and the establishment of novel genetic models to study the role of senescent cells in vivo.
Senescence regulatory networks and aging in vivo
Expression of the components of the INK4/ARF locus correlates with aging, and p16INK4a can be used as a prognostic marker for some age-related diseases, such as IPF and glomerulosclerosis (Melk et al., 2004; Lomas et al., 2012). Furthermore, p16INK4a accumulates during aging. For example, p16INK4a expression increases with age in the islet of Langerhans (Helman et al., 2016), renal cortex (Melk et al., 2004), and fat tissue (Xu et al., 2015; Baker et al., 2016), among other areas (Krishnamurthy et al., 2004). Its knockout also mitigates functional decline and proliferative exhaustion upon HSC transplantation (Janzen et al., 2006). Although these studies suggest that p16INK4a accumulation is detrimental during aging, increased gene dosage of INK4/ARF in super-p16 mice does not result in reduced life span. The possible detrimental effects cause by p16INK4a overexpression may be outweighed by their clear tumor suppressive benefits, with a threefold reduction in tumor incidence (Matheu et al., 2009). Similarly, p53−/− can ameliorate some of the effects of severe progeria mutants such as Ku80−/−, mTR−/−, and Zmpste24−/− (Chin et al., 1999; Lim et al., 2000; Varela et al., 2005), but the resulting increase in tumorigenesis obscures potential increases in life span. Mice with extra copies of p53 or p19ARF are more resistant to tumors and display delayed aging (García-Cao et al., 2002; Matheu et al., 2007).
Visualizing senescence in vivo
One of the biggest hindrances to investigating senescence in vivo has been the lack of robust, consistent markers. Most studies of senescence in aged tissues have relied on usage of senescence-associated β-galactosidase (SA-β-Gal) staining or the lack of proliferative markers such as Ki67. However, these may yield mixed results. For example, macrophages display elevated levels of SA-β-Gal activity. The use of additional senescence markers, such as lipofuscin, which accumulates in the cytoplasm of senescent cells, could be applied to bridge this gap (Sharpless and Sherr, 2015).
Another useful tool that has emerged is the use of bioluminescent senescence reporters. With the advent of p16INK4a-LUC mice expressing a luciferase reporter under the control of a p16INK4a promoter, there is now confirmation that multiple tissues show an exponential age-related increase in p16INK4a expression that correlates with higher levels of proinflammatory factors or SASP components (Yamakoshi et al., 2009; Burd et al., 2013).
Evaluating the consequences of targeting senescence during aging
Establishing causality of a gene in diseases such as cancer is usually a matter of generating appropriate knockout or overexpression mouse models. To evaluate the role of senescence in aging that approach gets complicated by the tumor suppressive roles of the INK4/ARF locus and p53 (see Pathways regulating the senescence growth arrest section). Seminal studies by Baker et al., first in progeroid BubR1 mice (Baker et al., 2011) and later in naturally aged mice (Baker et al., 2016), demonstrated that by expressing an inducible suicide gene under the control of the p16INK4a promoter it is possible to ablate senescent cells and improve health span. The elimination of senescent cells improved several age-associated conditions, delayed tumor formation, and ameliorated the side effects of chemotherapy (Baker et al., 2016; Demaria et al., 2016; Baar et al., 2017). The role of p16INK4a-positive senescent cells in age-related pathologies has been further confirmed by additional studies using other p16INK4a-based senescence ablation systems such as p16-3MR and INK-NTR mice (Demaria et al., 2014; Childs et al., 2016). These studies have finally confirmed that senescence causes, or at least contributes to, aging.
Senescence, the SASP, and age-related inflammation
There is clear evidence suggesting how the SASP participates in the clearance of premalignant cells or contributes to tumor progression (Kang et al., 2011; Eggert et al., 2016). Although it has been hypothesized that the SASP is responsible for tissue dysfunction during aging, we still lack direct evidence for the roles that the SASP may play in aging (Muñoz-Espín and Serrano, 2014).
IL-1α, IL-6, TNF, and NF-κB activity and other inflammatory factors have been found to increase in tissues with age and inhibition of NF-κB confers resistance to progeroid conditions (Tilstra et al., 2012). The detrimental role for chronic inflammation during aging is further supported by clinical data (Libby, 2002; Brunt et al., 2009; Dinarello et al., 2010; Balestro et al., 2016). Aging phenotypes such as frailty (Soysal et al., 2016) correlate with increased levels of proinflammatory factors. The increased levels of chronic inflammation in these instances are collectively termed inflammaging (Franceschi and Campisi, 2014). The reason for such increases in levels of proinflammatory molecules remains unknown. Although accumulated damage and lifelong antigenic load may undoubtedly contribute to this increase in inflammation, senescence may also help mediate inflammaging.
This contribution of senescence to inflammaging may be via several coalescing effects, the first being through the SASP. As damage accumulates in tissues, the number of senescent cells and their SASP also increases. This process is usually resolved by clearance of the senescent cells by the immune system (Kang et al., 2011). In aged individuals, however, senescence also contributes to a decline in immune function termed immunosenescence, thereby compromising the clearance of senescent cells and exacerbating inflammation. Emerging studies using genetic systems or drugs ablating senescent cells suggest that the elimination of senescent cells reduces inflammation across tissues (Baker et al., 2016; Jeon et al., 2017). Future studies will need to establish the causal link between the SASP, chronic inflammation, and tissue dysfunction. These might require the generation of novel mouse models that take advantage of our knowledge on SASP regulation.
Contribution of senescence to age-related diseases
Now that a general causative role for senescence during aging has been established, the next step is to identify how senescence contributes to different age-related pathologies such as glaucoma (Liton et al., 2005) or osteoarthitis (Jeon et al., 2017; Fig. 4). Thanks to the use of senolytic drugs and genetic models for senescence ablation, we are progressing quickly in that task.
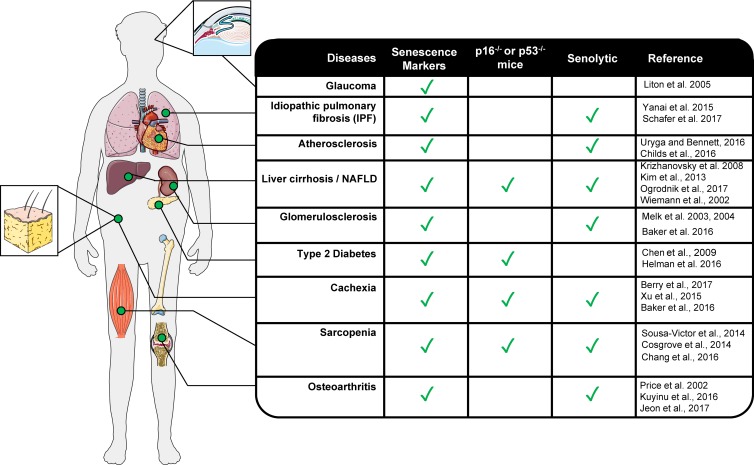
Involvement of senescence in disease. Establishment of robust biomarkers of senescence, usage of genetic knockout models and senolytic models are expanding our knowledge on the age-related diseases in which senescence plays a role.
Opposing roles for senescence in cancer
Age is a strong prognostic marker of reduced survival across many cancers (de Magalhães, 2013). Senescence is a strong tumor suppressor mechanism that limits cancer initiation through both cell-intrinsic (Collado and Serrano, 2010) and cell-extrinsic mechanisms (Kang et al., 2011). However, there is strong evidence suggesting that through the SASP, aged tissues provide a supportive niche for cancer (Coppé et al., 2010). Senescent cells can contribute to tumor progression by enhancing the proliferative potential of cancer cells (Krtolica et al., 2001) or contributing to epithelial to mesenchymal transition (Coppé et al., 2008). Therefore, the increased numbers of senescent cells present in aged tissues could contribute to the increased incidence of cancer with age. Supporting this, a delayed onset in tumor formation is observed when senescent cells are eliminated (Baker et al., 2016). Senolytic therapy also reduces the incidence of metastasis, the leading cause of cancer-related deaths (Demaria et al., 2016).
Renal dysfunction
Aged individuals often display a reduced glomerular filtration rate and cortical volume that can result in glomerulosclerosis and nephron atrophy, both of which are associated with increased expression of p16INK4a and p53 (Melk et al., 2003, 2004). Senescence has detrimental effects in most renal diseases analyzed (Sturmlechner et al., 2017). Ablation of senescent cells protects against glomerulosclerosis and improves kidney function in aged mice (Baker et al., 2016).
Type 2 diabetes
One of the largest risk factors for the development of type 2 diabetes is age. Several genome-wide association studies of type 2 diabetes have highlighted variants at the INK4/ARF locus, suggesting a possible link between senescence and diabetes (Zeggini et al., 2007; Jeck et al., 2012). In addition, senescence markers and IL-1β are elevated in β cells from diabetic mice (Sone and Kagawa, 2005; Dinarello et al., 2010). Surprisingly, although p16INK4a expression drove a decline in β cell regenerative capacity and predisposed mice to mild diabetes (Krishnamurthy et al., 2006; Chen et al., 2009), senescent β islets increased insulin secretion, making it unclear how senescence contributes to maintain glucose homeostasis (Helman et al., 2016).
IPF
Fibrosis is a pathological condition whereby tissue accumulates ECM proteins such as collagen, resulting in tissue scarification, usually in response to damage. Senescence appears to have both beneficial and detrimental roles during fibrosis and wound healing. Secretion of MMPs, which occurs as part of the SASP, could help in the resolution of fibrotic plaques (Craig et al., 2015). Conversely, fibroblasts and tissues isolated from IPF patients display increased levels of SA-β-Gal staining and p21CIP1, suggesting a link with senescence (Yanai et al., 2015; Schafer et al., 2017). The detrimental nature of senescence in IPF was recently demonstrated using senolytics. Elimination of senescent fibroblasts in a mouse model of lung fibrosis reduced expression of profibrotic SASP components and improved pulmonary function (Schafer et al., 2017).
Nonalcoholic fatty liver disease
Cirrhosis is the pathological outcome from liver fibrosis and nonalcoholic fatty liver disease, which in turn is a result of hepatic steatosis, the abnormal accumulation of lipids in hepatocytes (Pellicoro et al., 2014; Hardy et al., 2016). Senescence is associated with liver fibrosis (Kim et al., 2013) and cirrhosis (Wiemann et al., 2002). The risk of developing nonalcoholic fatty liver disease increases with age (Hardy et al., 2016) and is predicted by the presence of senescent hepatocytes (Pellicoro et al., 2014). The elimination of senescent cells using INK-ATTC mice reduces liver fat accumulation (Ogrodnik et al., 2017). The role of senescence in the liver is complex, however, because knocking out p53 or p16INK4a increases liver fibrosis (Krizhanovsky et al., 2008). Moreover, senescent hepatic stellate cells down-regulate collagen and up-regulate MMPs and cytokines that could remodel fibrotic plaques and recruit macrophages (Krizhanovsky et al., 2008).
Cardiovascular disease
The risk of developing atherosclerosis and cardiomyopathy and their respective conditions, coronary heart disease and heart failure, increases with age. In the case of atherosclerosis, the role of senescence has been confirmed using senolytic models (Childs et al., 2016). Ablation of senescent cells improved the stability of plaques and reduced both the incidence and progression of plaque formation. Senescent cells were initially identified in atherosclerosis in vascular smooth muscle cells at the site of the plaque (Uryga and Bennett, 2016). Subsequent studies showed that macrophages were the primary senescent cell present with higher levels of SA-β-Gal staining and SASP production, suggesting their key contribution to coronary heart disease (Childs et al., 2016). Cardiomyocyte atrophy is one of the underlying causes of myocardial infarction in the elderly (Niccoli and Partridge, 2012). It is unclear how ablation of senescent cells protects against cardiomyocyte hypertrophy in aged mice and provides resistance to cardiac stress (Baker et al., 2016).
Osteoarthritis
Lifelong wear and tear on ligaments is a significant risk factor for the development of arthritis. Failure of chondrocytes to produce cartilage results in degradation of joints and immobilization. Expression of p16INK4a in these cells correlates with severity and progression of the disease (Price et al., 2002). Moreover, when mice were subjected to an acute trauma to model osteoarthritis, senescent cells accumulated in the site of the injury (Kuyinu et al., 2016). Clearance of these senescent cells using senolytics resulted in the increased functionality of the remaining chondrocytes with rejuvenation of cartilage soon after (Jeon et al., 2017).
Decline in immune function with age
One of the primary risk factors for complications in end-of life care is infection. The inability of the body to raise a response to immune offenses is caused by a functional decline in HSCs. The accumulation of senescent HSCs with age contributes to immune decline and senescence bypass allows for stem cell rejuvenation. Interestingly, the removal of these cells restored the functionality of HSCs and increased myeloid, B, and T cell numbers in transplant experiments (Chang et al., 2016).
Sarcopenia
Muscle stem cells (MuSCs) undergo a decline in their ability to differentiate and facilitate repair of muscle tissue, which is hypothesized to be the underlying cause of age-dependent muscle wasting or sarcopenia. MuSCs are quiescent unless stimulated to repair muscle (Gopinath and Rando, 2008). However, with age, they become senescent, up-regulating p16INK4a (Sousa-Victor et al., 2014). The elimination of senescent MuSCs increases the ability of the remaining MuSCs to form muscle cell colonies (Chang et al., 2016). Additionally, inhibition of p38 or p16INK4a bypasses MuSC senescence and strengthens muscle in geriatric mice (Cosgrove et al., 2014; Sousa-Victor et al., 2014).
Age-related cachexia
Loss of adiposity and loss of muscle mass in aged individuals are primary contributors to age-dependent wastage or cachexia. White adipose tissue isolated from aged mice display SA-β-Gal activity (Baker et al., 2016). Removal of senescent cells from mice leads to increased adiposity and prevents mass loss in aged mice (Baker et al., 2016). Recently it has been shown that bypass of senescence or senolysis restores adipose beiging and adipogenesis and improves metabolic function in aged mice (Xu et al., 2015; Berry et al., 2017). This suggests that senescent cells prevent adipocyte differentiation and contribute to an age-dependent loss of adaptive thermogenic capacity and metabolic dysfunction.
Targeting senescence during aging
Because of the detrimental nature of senescence in the etiology of numerous diseases, disrupting or preventing senescence can delay health decline during aging. Inhibition of p38, disruption of p53 and p16, or lengthening of telomeres have all been shown to benefit aging phenotypes but carry a major caveat, as they can increase the incidence of cancer (Sharpless et al., 2001; Ito et al., 2006; Janzen et al., 2006; Shay, 2016). The selective elimination of senescent cells has revealed to be a safer route to target senescence during aging.
ABT-263, otherwise known as navitoclax, is a BH3 mimetic that blocks the interaction between antiapoptotic BCL-2 proteins and their targets, thereby releasing the brakes on the cell death machinery, and has been used to treat various cancers (Rudin et al., 2012; Chang et al., 2016). Its senolytic activity is explained by an overreliance of senescent cells on BCL-xL and BCL-w, both of which are up-regulated during senescence (Yosef et al., 2016). However, usage of navitoclax as a prophylactic senolytic drug is unlikely because of its severe thrombocytopenic and neutropenic effects (Rudin et al., 2012). Recently, it has been shown that localization of p53 to the nucleus by FOXO4 protects against p53 engaging the p53-mitochondrial signaling axis and apoptosis therein (Baar et al., 2017). Treatment of mice with a FOXO4 inhibitor peptide, FOXO4 D-retro inverso isoform, can delay different aging phenotypes. As with navitoclax, however, special attention must be paid to unintended effects of FOXO4 D-retro inverso isoform. For example, p21CIP1 expression fell markedly in senescent cells treated with the peptide.
Initial studies regarding senolytics are promising, but there are still lingering unknowns with regards to their effectivity as therapies. Senescent cells were observed to reappear after cessation of senolytic treatment in a model of osteoarthritis (Jeon et al., 2017). This could reflect unresolved damage from the anterior cruciate ligament injury surgery used in this study, although there is also the possibility that removal of senescent cells without targeting the causes that induce their accumulation could limit the benefits of senolytics. Another potential problem of using senolytics is an acceleration of stem cell exhaustion.
The clinical population in which senolytics could be used includes already-infirmed patients whose immune systems might be compromised. If senescent cells are eliminated and account for ~1–5% of cells in aged tissues (Sharpless and Sherr, 2015), where do they go? Apoptotic cells mark themselves for phagocytosis by the immune system with special “find me” and “eat me” signals. Can we expect an immune system that was unable to clear aberrantly accumulated senescent cells to infiltrate and clear apoptotic bodies? Without clearance of apoptotic bodies, secondary necrosis could result in the release of proinflammatory, danger-associated molecular patterns, further exacerbating systemic chronic inflammation. This could limit the effective therapeutic window for senolytic drugs. Although these are potential caveats for senolytic therapies, they may be significantly outweighed by their benefits. In addition, the senescence program varies across tissues, raising the possibility of identifying “tissue-specific” senolytics, which could minimize side effects. With these unknowns, it will be critical to validate the kinetics of senescent cells clearance using robust markers of senescence and improved methods for identifying senescent cells (Evangelou et al., 2017).
Exploring alternative approaches to target the detrimental effects of senescence without resorting to senolytics may also be worthwhile. One such approach would be targeting the SASP. Candidates to suppress or modulate the SASP include rapamycin, BRD4, NF-κB, or p38 inhibitors (Chien et al., 2011; Freund et al., 2011; Herranz et al., 2015; Laberge et al., 2015; Tasdemir et al., 2016). Possible side effects of this strategy could include blunting the senescent response, exacerbation of the accumulation of senescent cells, or immunosuppression. However, there are many potential routes for the development of new SASP modulators, which can be helped in no small part by improvements our understanding of how the SASP is regulated.
In summary, the past few years have unveiled a key role for senescence in aging. The advent of powerful genetic and pharmacological tools to dissect this relation should improve our understanding of the mechanisms through which the accumulation of senescence cells leads to age-related physiological decline. It should also inform in the development of new therapeutic approaches. Moreover, if targeting senescence using senolytics or by other strategies such as SASP-modulating drugs succeeds, it could not only contribute to the treatment of specific diseases but also improve the general health span of aged individuals.
Acknowledgments
The figures were designed using elements from Smart Servier medical art that are licensed under creative commons BY 3.0. terms.
Core support from the Medical Research Council (grants MC-A652-5PZ00 and MC_U120085810) funded the research in J. Gil’s laboratory. J. Gil is a consultant to Unity Biotechnology, which also funds research in his laboratory.
The authors declare no competing financial interests.
Footnotes
Abbreviations used:
- FOXO4
- Forkhead box protein O4
- HSC
- hematopoietic stem cell
- IL
- interleukin
- IPF
- idiopathic pulmonary fibrosis
- MMP
- matrix metalloproteinase
- MuSC
- muscle stem cell
- NF-κB
- nuclear factor κB
- NSC
- neural stem cell
- PRC
- Polycomb repressive complex
- SA-β-Gal
- senescence-associated β-galactosidase
- SASP
- senescence-associated secretory phenotype
References
- Acosta J.C., O’Loghlen A., Banito A., Guijarro M.V., Augert A., Raguz S., Fumagalli M., Da Costa M., Brown C., Popov N., et al. . 2008. Chemokine signaling via the CXCR2 receptor reinforces senescence. Cell. 133:1006–1018. 10.1016/j.cell.2008.03.038 [Abstract] [CrossRef] [Google Scholar]
- Acosta J.C., Banito A., Wuestefeld T., Georgilis A., Janich P., Morton J.P., Athineos D., Kang T.-W., Lasitschka F., Andrulis M., et al. . 2013. A complex secretory program orchestrated by the inflammasome controls paracrine senescence. Nat. Cell Biol. 15:978–990. 10.1038/ncb2784 [Europe PMC free article] [Abstract] [CrossRef] [Google Scholar]
- Agger K., Cloos P.A.C., Rudkjaer L., Williams K., Andersen G., Christensen J., and Helin K.. 2009. The H3K27me3 demethylase JMJD3 contributes to the activation of the INK4A-ARF locus in response to oncogene- and stress-induced senescence. Genes Dev. 23:1171–1176. 10.1101/gad.510809 [Europe PMC free article] [Abstract] [CrossRef] [Google Scholar]
- Allsopp R.C., Cheshier S., and Weissman I.L.. 2002. Telomerase activation and rejuvenation of telomere length in stimulated T cells derived from serially transplanted hematopoietic stem cells. J. Exp. Med. 196:1427–1433. 10.1084/jem.20021003 [Europe PMC free article] [Abstract] [CrossRef] [Google Scholar]
- Baar M.P., Brandt R.M.C., Putavet D.A., Klein J.D.D., Derks K.W.J., Bourgeois B.R.M., Stryeck S., Rijksen Y., van Willigenburg H., Feijtel D.A., et al. . 2017. Targeted Apoptosis of Senescent Cells Restores Tissue Homeostasis in Response to Chemotoxicity and Aging. Cell. 169:132–147.e16. 10.1016/j.cell.2017.02.031 [Europe PMC free article] [Abstract] [CrossRef] [Google Scholar]
- Baker D.J., Wijshake T., Tchkonia T., LeBrasseur N.K., Childs B.G., van de Sluis B., Kirkland J.L., and van Deursen J.M.. 2011. Clearance of p16Ink4a-positive senescent cells delays ageing-associated disorders. Nature. 479:232–236. 10.1038/nature10600 [Europe PMC free article] [Abstract] [CrossRef] [Google Scholar]
- Baker D.J., Childs B.G., Durik M., Wijers M.E., Sieben C.J., Zhong J., Saltness R.A., Jeganathan K.B., Verzosa G.C., Pezeshki A., et al. . 2016. Naturally occurring p16(Ink4a)-positive cells shorten healthy lifespan. Nature. 530:184–189. 10.1038/nature16932 [Europe PMC free article] [Abstract] [CrossRef] [Google Scholar]
- Balestro E., Calabrese F., Turato G., Lunardi F., Bazzan E., Marulli G., Biondini D., Rossi E., Sanduzzi A., Rea F., et al. . 2016. Immune inflammation and disease progression in idiopathic pulmonary fibrosis. PLoS One. 11:e0154516 10.1371/journal.pone.0154516 [Europe PMC free article] [Abstract] [CrossRef] [Google Scholar]
- Barradas M., Anderton E., Acosta J.C., Li S., Banito A., Rodriguez-Niedenführ M., Maertens G., Banck M., Zhou M.M., Walsh M.J., et al. . 2009. Histone demethylase JMJD3 contributes to epigenetic control of INK4a/ARF by oncogenic RAS. Genes Dev. 23:1177–1182. 10.1101/gad.511109 [Europe PMC free article] [Abstract] [CrossRef] [Google Scholar]
- Berry D.C., Jiang Y., Arpke R.W., Close E.L., Uchida A., Reading D., Berglund E.D., Kyba M., and Graff J.M.. 2017. Cellular Aging Contributes to Failure of Cold-Induced Beige Adipocyte Formation in Old Mice and Humans. Cell Metab. 25:166–181. 10.1016/j.cmet.2016.10.023 [Europe PMC free article] [Abstract] [CrossRef] [Google Scholar]
- Bracken A.P., Kleine-Kohlbrecher D., Dietrich N., Pasini D., Gargiulo G., Beekman C., Theilgaard-Mönch K., Minucci S., Porse B.T., Marine J.-C., et al. . 2007. The Polycomb group proteins bind throughout the INK4A-ARF locus and are disassociated in senescent cells. Genes Dev. 21:525–530. 10.1101/gad.415507 [Europe PMC free article] [Abstract] [CrossRef] [Google Scholar]
- Brunt E.M., Kleiner D.E., Wilson L.A., Unalp A., Behling C.E., Lavine J.E., and Neuschwander-Tetri B.A.; NASH Clinical Research Network . 2009. Portal chronic inflammation in nonalcoholic fatty liver disease (NAFLD): a histologic marker of advanced NAFLD-Clinicopathologic correlations from the nonalcoholic steatohepatitis clinical research network. Hepatology. 49:809–820. 10.1002/hep.22724 [Europe PMC free article] [Abstract] [CrossRef] [Google Scholar]
- Burd C.E., Sorrentino J.A., Clark K.S., Darr D.B., Krishnamurthy J., Deal A.M., Bardeesy N., Castrillon D.H., Beach D.H., and Sharpless N.E.. 2013. Monitoring tumorigenesis and senescence in vivo with a p16(INK4a)-luciferase model. Cell. 152:340–351. 10.1016/j.cell.2012.12.010 [Europe PMC free article] [Abstract] [CrossRef] [Google Scholar]
- Capell B.C., Drake A.M., Zhu J., Shah P.P., Dou Z., Dorsey J., Simola D.F., Donahue G., Sammons M., Rai T.S., et al. . 2016. MLL1 is essential for the senescence-associated secretory phenotype. Genes Dev. 30:321–336. 10.1101/gad.271882.115 [Europe PMC free article] [Abstract] [CrossRef] [Google Scholar]
- Cawthon R.M., Smith K.R., O’Brien E., Sivatchenko A., and Kerber R.A.. 2003. Association between telomere length in blood and mortality in people aged 60 years or older. Lancet. 361:393–395. 10.1016/S0140-6736(03)12384-7 [Abstract] [CrossRef] [Google Scholar]
- Chang J., Wang Y., Shao L., Laberge R.M., Demaria M., Campisi J., Janakiraman K., Sharpless N.E., Ding S., Feng W., et al. . 2016. Clearance of senescent cells by ABT263 rejuvenates aged hematopoietic stem cells in mice. Nat. Med. 22:78–83. 10.1038/nm.4010 [Europe PMC free article] [Abstract] [CrossRef] [Google Scholar]
- Chen H., Gu X., Su I., Bottino R., Contreras J.L., Tarakhovsky A., and Kim S.K.. 2009. Polycomb protein Ezh2 regulates pancreatic β-cell Ink4a/Arf expression and regeneration in streptozotocin-induced diabetes mellitus. Genes Dev. 23:975–985. 10.1101/gad.1742509 [Europe PMC free article] [Abstract] [CrossRef] [Google Scholar]
- Chien Y., Scuoppo C., Wang X., Fang X., Balgley B., Bolden J.E., Premsrirut P., Luo W., Chicas A., Lee C.S., et al. . 2011. Control of the senescence-associated secretory phenotype by NF-κB promotes senescence and enhances chemosensitivity. Genes Dev. 25:2125–2136. 10.1101/gad.17276711 [Europe PMC free article] [Abstract] [CrossRef] [Google Scholar]
- Childs B.G., Baker D.J., Wijshake T., Conover C.A., Campisi J., and van Deursen J.M.. 2016. Senescent intimal foam cells are deleterious at all stages of atherosclerosis. Science. 354:472–477. [Europe PMC free article] [Abstract] [Google Scholar]
- Chin L., Artandi S.E., Shen Q., Tam A., Lee S.L., Gottlieb G.J., Greider C.W., and DePinho R.A.. 1999. p53 deficiency rescues the adverse effects of telomere loss and cooperates with telomere dysfunction to accelerate carcinogenesis. Cell. 97:527–538. 10.1016/S0092-8674(00)80762-X [Abstract] [CrossRef] [Google Scholar]
- Chung P.L., Zhou S., Eslami B., Shen L., LeBoff M.S., and Glowacki J.. 2014. Effect of age on regulation of human osteoclast differentiation. J. Cell. Biochem. 115:1412–1419. 10.1002/jcb.24792 [Europe PMC free article] [Abstract] [CrossRef] [Google Scholar]
- Collado M., and Serrano M.. 2010. Senescence in tumours: evidence from mice and humans. Nat. Rev. Cancer. 10:51–57. 10.1038/nrc2772 [Europe PMC free article] [Abstract] [CrossRef] [Google Scholar]
- Collado M., Blasco M.A., and Serrano M.. 2007. Cellular senescence in cancer and aging. Cell. 130:223–233. 10.1016/j.cell.2007.07.003 [Abstract] [CrossRef] [Google Scholar]
- Coppé J.P., Kauser K., Campisi J., and Beauséjour C.M.. 2006. Secretion of vascular endothelial growth factor by primary human fibroblasts at senescence. J. Biol. Chem. 281:29568–29574. 10.1074/jbc.M603307200 [Abstract] [CrossRef] [Google Scholar]
- Coppé J.-P., Patil C.K., Rodier F., Sun Y., Muñoz D.P., Goldstein J., Nelson P.S., Desprez P.-Y., and Campisi J.. 2008. Senescence-associated secretory phenotypes reveal cell-nonautonomous functions of oncogenic RAS and the p53 tumor suppressor. PLoS Biol. 6:2853–2868. 10.1371/journal.pbio.0060301 [Europe PMC free article] [Abstract] [CrossRef] [Google Scholar]
- Coppé J.-P., Desprez P.-Y., Krtolica A., and Campisi J.. 2010. The senescence-associated secretory phenotype: the dark side of tumor suppression. Annu. Rev. Pathol. 5:99–118. 10.1146/annurev-pathol-121808-102144 [Europe PMC free article] [Abstract] [CrossRef] [Google Scholar]
- Cosgrove B.D., Gilbert P.M., Porpiglia E., Mourkioti F., Lee S.P., Corbel S.Y., Llewellyn M.E., Delp S.L., and Blau H.M.. 2014. Rejuvenation of the muscle stem cell population restores strength to injured aged muscles. Nat. Med. 20:255–264. 10.1038/nm.3464 [Europe PMC free article] [Abstract] [CrossRef] [Google Scholar]
- Craig V.J., Zhang L., Hagood J.S., and Owen C.A.. 2015. Matrix metalloproteinases as therapeutic targets for idiopathic pulmonary fibrosis. Am. J. Respir. Cell Mol. Biol. 53:585–600. 10.1165/rcmb.2015-0020TR [Europe PMC free article] [Abstract] [CrossRef] [Google Scholar]
- d’Adda di Fagagna F. 2008. Living on a break: cellular senescence as a DNA-damage response. Nat. Rev. Cancer. 8:512–522. 10.1038/nrc2440 [Abstract] [CrossRef] [Google Scholar]
- Dandona P., Aljada A., and Bandyopadhyay A.. 2004. Inflammation: the link between insulin resistance, obesity and diabetes. Trends Immunol. 25:4–7. 10.1016/j.it.2003.10.013 [Abstract] [CrossRef] [Google Scholar]
- de Magalhães J.P. 2013. How ageing processes influence cancer. Nat. Rev. Cancer. 13:357–365. 10.1038/nrc3497 [Abstract] [CrossRef] [Google Scholar]
- Demaria M., Ohtani N., Youssef S.A., Rodier F., Toussaint W., Mitchell J.R., Laberge R.M., Vijg J., Van Steeg H., Dollé M.E.T., et al. . 2014. An essential role for senescent cells in optimal wound healing through secretion of PDGF-AA. Dev. Cell. 31:722–733. 10.1016/j.devcel.2014.11.012 [Europe PMC free article] [Abstract] [CrossRef] [Google Scholar]
- Demaria M., O’Leary M.N., Chang J., Shao L., Liu S., Alimirah F., Koenig K., Le C., Mitin N., Deal A.M., et al. . 2016. Cellular Senescence Promotes Adverse Effects of Chemotherapy and Cancer Relapse. Cancer Discov. 7:165–176. [Europe PMC free article] [Abstract] [Google Scholar]
- Demontis F., and Perrimon N.. 2010. FOXO/4E-BP signaling in Drosophila muscles regulates organism-wide proteostasis during aging. Cell. 143:813–825. 10.1016/j.cell.2010.10.007 [Europe PMC free article] [Abstract] [CrossRef] [Google Scholar]
- Denchi E.L., and de Lange T.. 2007. Protection of telomeres through independent control of ATM and ATR by TRF2 and POT1. Nature. 448:1068–1071. 10.1038/nature06065 [Abstract] [CrossRef] [Google Scholar]
- Di Mitri D., Toso A., Chen J.J., Sarti M., Pinton S., Jost T.R., D’Antuono R., Montani E., Garcia-Escudero R., Guccini I., et al. . 2014. Tumour-infiltrating Gr-1+ myeloid cells antagonize senescence in cancer. Nature. 515:134–137. 10.1038/nature13638 [Abstract] [CrossRef] [Google Scholar]
- Dinarello C.A., Donath M.Y., and Mandrup-Poulsen T.. 2010. Role of IL-1beta in type 2 diabetes. Curr. Opin. Endocrinol. Diabetes Obes. 17:314–321. [Abstract] [Google Scholar]
- Doles J., Storer M., Cozzuto L., Roma G., and Keyes W.M.. 2012. Age-associated inflammation inhibits epidermal stem cell function. Genes Dev. 26:2144–2153. 10.1101/gad.192294.112 [Europe PMC free article] [Abstract] [CrossRef] [Google Scholar]
- Dou Z., Ghosh K., Vizioli M.G., Zhu J., Sen P., Wangensteen K.J., Simithy J., Lan Y., Lin Y., Zhou Z., et al. . 2017. Cytoplasmic chromatin triggers inflammation in senescence and cancer. Nature. 550:402–406; advance online publication 10.1038/nature24050 [Europe PMC free article] [Abstract] [CrossRef] [Google Scholar]
- Eggert T., Wolter K., Ji J., Ma C., Yevsa T., Klotz S., Medina-Echeverz J., Longerich T., Forgues M., Reisinger F., et al. . 2016. Distinct Functions of Senescence-Associated Immune Responses in Liver Tumor Surveillance and Tumor Progression. Cancer Cell. 30:533–547. 10.1016/j.ccell.2016.09.003 [Europe PMC free article] [Abstract] [CrossRef] [Google Scholar]
- Evangelou K., Lougiakis N., Rizou S.V., Kotsinas A., Kletsas D., Muñoz-Espín D., Kastrinakis N.G., Pouli N., Marakos P., Townsend P., et al. . 2017. Robust, universal biomarker assay to detect senescent cells in biological specimens. Aging Cell. 16:192–197. 10.1111/acel.12545 [Europe PMC free article] [Abstract] [CrossRef] [Google Scholar]
- Eyman D., Damodarasamy M., Plymate S.R., and Reed M.J.. 2009. CCL5 secreted by senescent aged fibroblasts induces proliferation of prostate epithelial cells and expression of genes that modulate angiogenesis. J. Cell. Physiol. 220:376–381. 10.1002/jcp.21776 [Europe PMC free article] [Abstract] [CrossRef] [Google Scholar]
- Franceschi C., and Campisi J.. 2014. Chronic inflammation (inflammaging) and its potential contribution to age-associated diseases. J. Gerontol. A Biol. Sci. Med. Sci. 69(Suppl. 1):S4–S9. 10.1093/gerona/glu057 [Abstract] [CrossRef] [Google Scholar]
- Freund A., Patil C.K., and Campisi J.. 2011. p38MAPK is a novel DNA damage response-independent regulator of the senescence-associated secretory phenotype. EMBO J. 30:1536–1548. 10.1038/emboj.2011.69 [Europe PMC free article] [Abstract] [CrossRef] [Google Scholar]
- Fried L.P., Tangen C.M., Walston J., Newman A.B., Hirsch C., Gottdiener J., Seeman T., Tracy R., Kop W.J., Burke G., and McBurnie M.A.. Cardiovascular Health Study Collaborative Research Group . 2001. Frailty in older adults: evidence for a phenotype. J. Gerontol. A Biol. Sci. Med. Sci. 56:M146–M156. 10.1093/gerona/56.3.M146 [Abstract] [CrossRef] [Google Scholar]
- Fumagalli M., Rossiello F., Clerici M., Barozzi S., Cittaro D., Kaplunov J.M., Bucci G., Dobreva M., Matti V., Beausejour C.M., et al. . 2012. Telomeric DNA damage is irreparable and causes persistent DNA-damage-response activation. Nat. Cell Biol. 14:355–365. 10.1038/ncb2466 [Europe PMC free article] [Abstract] [CrossRef] [Google Scholar]
- García-Cao I., García-Cao M., Martín-Caballero J., Criado L.M., Klatt P., Flores J.M., Weill J., Blasco M.A., and Serrano M.. 2002. “Super p53” mice exhibit enhanced DNA damage response, are tumor resistant and age normally. EMBO J . 21:6225–6235. [Europe PMC free article] [Abstract] [Google Scholar]
- García-Prat L., Martínez-Vicente M., Perdiguero E., Ortet L., Rodríguez-Ubreva J., Rebollo E., Ruiz-Bonilla V., Gutarra S., Ballestar E., Serrano A.L., et al. . 2016. Autophagy maintains stemness by preventing senescence. Nature. 529:37–42. 10.1038/nature16187 [Abstract] [CrossRef] [Google Scholar]
- Geiger H., and Van Zant G.. 2002. The aging of lympho-hematopoietic stem cells. Nat. Immunol. 3:329–333. 10.1038/ni0402-329 [Abstract] [CrossRef] [Google Scholar]
- Gil J., and Peters G.. 2006. Regulation of the INK4b-ARF-INK4a tumour suppressor locus: all for one or one for all. Nat. Rev. Mol. Cell Biol. 7:667–677. 10.1038/nrm1987 [Abstract] [CrossRef] [Google Scholar]
- Gil J., Bernard D., Martínez D., and Beach D.. 2004. Polycomb CBX7 has a unifying role in cellular lifespan. Nat. Cell Biol. 6:67–72. 10.1038/ncb1077 [Abstract] [CrossRef] [Google Scholar]
- Glück S., Guey B., Gulen M.F., Wolter K., Kang T.-W., Schmacke N.A., Bridgeman A., Rehwinkel J., Zender L., and Ablasser A.. 2017. Innate immune sensing of cytosolic chromatin fragments through cGAS promotes senescence. Nat. Cell Biol. 19:1061–1070. 10.1038/ncb3586 [Europe PMC free article] [Abstract] [CrossRef] [Google Scholar]
- Gopinath S.D., and Rando T.A.. 2008. Stem cell review series: aging of the skeletal muscle stem cell niche. Aging Cell. 7:590–598. 10.1111/j.1474-9726.2008.00399.x [Abstract] [CrossRef] [Google Scholar]
- Gunasekaran U., and Gannon M.. 2011. Type 2 diabetes and the aging pancreatic beta cell. Aging (Albany NY). 3:565–575. 10.18632/aging.100350 [Europe PMC free article] [Abstract] [CrossRef] [Google Scholar]
- Hanahan D., and Weinberg R.A.. 2011. Hallmarks of cancer: the next generation. Cell. 144:646–674. 10.1016/j.cell.2011.02.013 [Abstract] [CrossRef] [Google Scholar]
- Hardy T., Oakley F., Anstee Q.M., and Day C.P.. 2016. Nonalcoholic Fatty Liver Disease: Pathogenesis and Disease Spectrum. Annu. Rev. Pathol. 11:451–496. 10.1146/annurev-pathol-012615-044224 [Abstract] [CrossRef] [Google Scholar]
- Harris S.L., and Levine A.J.. 2005. The p53 pathway: positive and negative feedback loops. Oncogene. 24:2899–2908. 10.1038/sj.onc.1208615 [Abstract] [CrossRef] [Google Scholar]
- Harrison D.E., Strong R., Sharp Z.D., Nelson J.F., Astle C.M., Flurkey K., Nadon N.L., Wilkinson J.E., Frenkel K., Carter C.S., et al. . 2009. Rapamycin fed late in life extends lifespan in genetically heterogeneous mice. Nature. 460:392–395. [Europe PMC free article] [Abstract] [Google Scholar]
- Hayflick L., and Moorhead P.S.. 1961. The serial cultivation of human diploid cell strains. Exp. Cell Res. 25:585–621. 10.1016/0014-4827(61)90192-6 [Abstract] [CrossRef] [Google Scholar]
- Helman A., Klochendler A., Azazmeh N., Gabai Y., Horwitz E., Anzi S., Swisa A., Condiotti R., Granit R.Z., Nevo Y., et al. . 2016. p16(Ink4a)-induced senescence of pancreatic beta cells enhances insulin secretion. Nat. Med. 22:412–420. 10.1038/nm.4054 [Europe PMC free article] [Abstract] [CrossRef] [Google Scholar]
- Herranz N., Gallage S., Mellone M., Wuestefeld T., Klotz S., Hanley C.J., Raguz S., Acosta J.C., Innes A.J., Banito A., et al. . 2015. mTOR regulates MAPKAPK2 translation to control the senescence-associated secretory phenotype. Nat. Cell Biol. 17:1205–1217. 10.1038/ncb3225 [Europe PMC free article] [Abstract] [CrossRef] [Google Scholar]
- Hoare M., Ito Y., Kang T.-W., Weekes M.P., Matheson N.J., Patten D.A., Shetty S., Parry A.J., Menon S., Salama R., et al. . 2016. NOTCH1 mediates a switch between two distinct secretomes during senescence. Nat. Cell Biol. 18:979–992. 10.1038/ncb3397 [Europe PMC free article] [Abstract] [CrossRef] [Google Scholar]
- Houtkooper R.H., Pirinen E., and Auwerx J.. 2012. Sirtuins as regulators of metabolism and healthspan. Nat. Rev. Mol. Cell Biol. 13:225–238. [Europe PMC free article] [Abstract] [Google Scholar]
- Ito K., Hirao A., Arai F., Takubo K., Matsuoka S., Miyamoto K., Ohmura M., Naka K., Hosokawa K., Ikeda Y., and Suda T.. 2006. Reactive oxygen species act through p38 MAPK to limit the lifespan of hematopoietic stem cells. Nat. Med. 12:446–451. 10.1038/nm1388 [Abstract] [CrossRef] [Google Scholar]
- Jacobs J.J.L., Kieboom K., Marino S., DePinho R.A., and van Lohuizen M.. 1999. The oncogene and Polycomb-group gene bmi-1 regulates cell proliferation and senescence through the ink4a locus. Nature. 397:164–168. 10.1038/16476 [Abstract] [CrossRef] [Google Scholar]
- Janzen V., Forkert R., Fleming H.E., Saito Y., Waring M.T., Dombkowski D.M., Cheng T., DePinho R.A., Sharpless N.E., and Scadden D.T.. 2006. Stem-cell ageing modified by the cyclin-dependent kinase inhibitor p16INK4a. Nature. 443:421–426. [Abstract] [Google Scholar]
- Jaskelioff M., Muller F.L., Paik J.-H., Thomas E., Jiang S., Adams A.C., Sahin E., Kost-Alimova M., Protopopov A., Cadiñanos J., et al. . 2011. Telomerase reactivation reverses tissue degeneration in aged telomerase-deficient mice. Nature. 469:102–106. 10.1038/nature09603 [Europe PMC free article] [Abstract] [CrossRef] [Google Scholar]
- Jeck W.R., Siebold A.P., and Sharpless N.E.. 2012. Review: a meta-analysis of GWAS and age-associated diseases. Aging Cell. 11:727–731. 10.1111/j.1474-9726.2012.00871.x [Europe PMC free article] [Abstract] [CrossRef] [Google Scholar]
- Jeon O.H., Kim C., Laberge R.-M., Demaria M., Rathod S., Vasserot A.P., Chung J.W., Kim D.H., Poon Y., David N., et al. . 2017. Local clearance of senescent cells attenuates the development of post-traumatic osteoarthritis and creates a pro-regenerative environment. Nat. Med. 23:775–781. 10.1038/nm.4324 [Europe PMC free article] [Abstract] [CrossRef] [Google Scholar]
- Kang C., Xu Q., Martin T.D., Li M.Z., Demaria M., Aron L., Lu T., Yankner B.A., Campisi J., and Elledge S.J.. 2015. The DNA damage response induces inflammation and senescence by inhibiting autophagy of GATA4. Science. 349:aaa5612 10.1126/science.aaa5612 [Europe PMC free article] [Abstract] [CrossRef] [Google Scholar]
- Kang T.-W., Yevsa T., Woller N., Hoenicke L., Wuestefeld T., Dauch D., Hohmeyer A., Gereke M., Rudalska R., Potapova A., et al. . 2011. Senescence surveillance of pre-malignant hepatocytes limits liver cancer development. Nature. 479:547–551. 10.1038/nature10599 [Abstract] [CrossRef] [Google Scholar]
- Kim W.Y., and Sharpless N.E.. 2006. The regulation of INK4/ARF in cancer and aging. Cell. 127:265–275. 10.1016/j.cell.2006.10.003 [Abstract] [CrossRef] [Google Scholar]
- Kim K.-H., Chen C.-C., Monzon R.I., and Lau L.F.. 2013. Matricellular protein CCN1 promotes regression of liver fibrosis through induction of cellular senescence in hepatic myofibroblasts. Mol. Cell. Biol. 33:2078–2090. 10.1128/MCB.00049-13 [Europe PMC free article] [Abstract] [CrossRef] [Google Scholar]
- Kollman C., Howe C.W.S., Anasetti C., Antin J.H., Davies S.M., Filipovich A.H., Hegland J., Kamani N., Kernan N.A., King R., et al. . 2001. Donor characteristics as risk factors in recipients after transplantation of bone marrow from unrelated donors: the effect of donor age. Blood. 98:2043–2051. [Abstract] [Google Scholar]
- Kotake Y., Nakagawa T., Kitagawa K., Suzuki S., Liu N., Kitagawa M., and Xiong Y.. 2011. Long non-coding RNA ANRIL is required for the PRC2 recruitment to and silencing of p15(INK4B) tumor suppressor gene. Oncogene. 30:1956–1962. 10.1038/onc.2010.568 [Europe PMC free article] [Abstract] [CrossRef] [Google Scholar]
- Krishnamurthy J., Torrice C., Ramsey M.R., Kovalev G.I., Al-Regaiey K., Su L., and Sharpless N.E.. 2004. Ink4a/Arf expression is a biomarker of aging. J. Clin. Invest. 114:1299–1307. 10.1172/JCI22475 [Europe PMC free article] [Abstract] [CrossRef] [Google Scholar]
- Krishnamurthy J., Ramsey M.R., Ligon K.L., Torrice C., Koh A., Bonner-Weir S., and Sharpless N.E.. 2006. p16INK4a induces an age-dependent decline in islet regenerative potential. Nature. 443:453–457. 10.1038/nature05092 [Abstract] [CrossRef] [Google Scholar]
- Krizhanovsky V., Yon M., Dickins R.A., Hearn S., Simon J., Miething C., Yee H., Zender L., and Lowe S.W.. 2008. Senescence of activated stellate cells limits liver fibrosis. Cell. 134:657–667. 10.1016/j.cell.2008.06.049 [Europe PMC free article] [Abstract] [CrossRef] [Google Scholar]
- Krtolica A., Parrinello S., Lockett S., Desprez P.Y., and Campisi J.. 2001. Senescent fibroblasts promote epithelial cell growth and tumorigenesis: a link between cancer and aging. Proc. Natl. Acad. Sci. USA. 98:12072–12077. 10.1073/pnas.211053698 [Europe PMC free article] [Abstract] [CrossRef] [Google Scholar]
- Kruiswijk F., Labuschagne C.F., and Vousden K.H.. 2015. p53 in survival, death and metabolic health: a lifeguard with a licence to kill. Nat. Rev. Mol. Cell Biol. 16:393–405. 10.1038/nrm4007 [Abstract] [CrossRef] [Google Scholar]
- Kuilman T., and Peeper D.S.. 2009. Senescence-messaging secretome: SMS-ing cellular stress. Nat. Rev. Cancer. 9:81–94. 10.1038/nrc2560 [Abstract] [CrossRef] [Google Scholar]
- Kuilman T., Michaloglou C., Vredeveld L.C.W., Douma S., van Doorn R., Desmet C.J., Aarden L.A., Mooi W.J., and Peeper D.S.. 2008. Oncogene-induced senescence relayed by an interleukin-dependent inflammatory network. Cell. 133:1019–1031. 10.1016/j.cell.2008.03.039 [Abstract] [CrossRef] [Google Scholar]
- Kuilman T., Michaloglou C., Mooi W.J., and Peeper D.S.. 2010. The essence of senescence. Genes Dev. 24:2463–2479. 10.1101/gad.1971610 [Europe PMC free article] [Abstract] [CrossRef] [Google Scholar]
- Kuyinu E.L., Narayanan G., Nair L.S., and Laurencin C.T.. 2016. Animal models of osteoarthritis: classification, update, and measurement of outcomes. J. Orthop. Surg. 11:19 10.1186/s13018-016-0346-5 [Europe PMC free article] [Abstract] [CrossRef] [Google Scholar]
- Kwon Y.H., Fingert J.H., Kuehn M.H., and Alward W.L.M.. 2009. Primary open-angle glaucoma. N. Engl. J. Med. 360:1113–1124. 10.1056/NEJMra0804630 [Europe PMC free article] [Abstract] [CrossRef] [Google Scholar]
- Laberge R.-M., Sun Y., Orjalo A.V., Patil C.K., Freund A., Zhou L., Curran S.C., Davalos A.R., Wilson-Edell K.A., Liu S., et al. . 2015. MTOR regulates the pro-tumorigenic senescence-associated secretory phenotype by promoting IL1A translation. Nat. Cell Biol. 17:1049–1061. 10.1038/ncb3195 [Europe PMC free article] [Abstract] [CrossRef] [Google Scholar]
- Lapasset L., Milhavet O., Prieur A., Besnard E., Babled A., Aït-Hamou N., Leschik J., Pellestor F., Ramirez J.-M., De Vos J., et al. . 2011. Rejuvenating senescent and centenarian human cells by reprogramming through the pluripotent state. Genes Dev. 25:2248–2253. 10.1101/gad.173922.111 [Europe PMC free article] [Abstract] [CrossRef] [Google Scholar]
- Lavasani M., Robinson A.R., Lu A., Song M., Feduska J.M., Ahani B., Tilstra J.S., Feldman C.H., Robbins P.D., Niedernhofer L.J., and Huard J.. 2012. Muscle-derived stem/progenitor cell dysfunction limits healthspan and lifespan in a murine progeria model. Nat. Commun. 3:608 10.1038/ncomms1611 [Europe PMC free article] [Abstract] [CrossRef] [Google Scholar]
- Lee H.W., Blasco M.A., Gottlieb G.J., Horner J.W. II, Greider C.W., and DePinho R.A.. 1998. Essential role of mouse telomerase in highly proliferative organs. Nature. 392:569–574. 10.1038/33345 [Abstract] [CrossRef] [Google Scholar]
- Libby P. 2002. Inflammation in atherosclerosis. Nature. 420:868–874. 10.1038/nature01323 [Abstract] [CrossRef] [Google Scholar]
- Lim D.-S., Vogel H., Willerford D.M., Sands A.T., Platt K.A., and Hasty P.. 2000. Analysis of ku80-mutant mice and cells with deficient levels of p53. Mol. Cell. Biol. 20:3772–3780. 10.1128/MCB.20.11.3772-3780.2000 [Europe PMC free article] [Abstract] [CrossRef] [Google Scholar]
- Liton P.B., Challa P., Stinnett S., Luna C., Epstein D.L., and Gonzalez P.. 2005. Cellular senescence in the glaucomatous outflow pathway. Exp. Gerontol. 40:745–748. 10.1016/j.exger.2005.06.005 [Europe PMC free article] [Abstract] [CrossRef] [Google Scholar]
- Loeser R.F. 2009. Aging and osteoarthritis: the role of chondrocyte senescence and aging changes in the cartilage matrix. Osteoarthritis Cartilage. 17:971–979. 10.1016/j.joca.2009.03.002 [Europe PMC free article] [Abstract] [CrossRef] [Google Scholar]
- Lomas N.J., Watts K.L., Akram K.M., Forsyth N.R., and Spiteri M.A.. 2012. Idiopathic pulmonary fibrosis: immunohistochemical analysis provides fresh insights into lung tissue remodelling with implications for novel prognostic markers. Int. J. Clin. Exp. Pathol. 5:58–71. [Europe PMC free article] [Abstract] [Google Scholar]
- López-Otín C., Blasco M.A., Partridge L., Serrano M., and Kroemer G.. 2013. The hallmarks of aging. Cell. 153:1194–1217. 10.1016/j.cell.2013.05.039 [Europe PMC free article] [Abstract] [CrossRef] [Google Scholar]
- Martin N., Popov N., Aguilo F., O’Loghlen A., Raguz S., Snijders A.P., Dharmalingam G., Li S., Thymiakou E., Carroll T., et al. . 2013. Interplay between Homeobox proteins and Polycomb repressive complexes in p16INK4a regulation. EMBO J. 32:982–995. 10.1038/emboj.2013.37 [Europe PMC free article] [Abstract] [CrossRef] [Google Scholar]
- Martin N., Beach D., and Gil J.. 2014. Ageing as developmental decay: insights from p16(INK4a.). Trends Mol. Med. 20:667–674. 10.1016/j.molmed.2014.09.008 [Abstract] [CrossRef] [Google Scholar]
- Matheu A., Maraver A., Klatt P., Flores I., Garcia-Cao I., Borras C., Flores J.M., Viña J., Blasco M.A., and Serrano M.. 2007. Delayed ageing through damage protection by the Arf/p53 pathway. Nature. 448:375–379. 10.1038/nature05949 [Abstract] [CrossRef] [Google Scholar]
- Matheu A., Maraver A., Collado M., Garcia-Cao I., Cañamero M., Borras C., Flores J.M., Klatt P., Viña J., and Serrano M.. 2009. Anti-aging activity of the Ink4/Arf locus. Aging Cell. 8:152–161. 10.1111/j.1474-9726.2009.00458.x [Abstract] [CrossRef] [Google Scholar]
- Melk A., Kittikowit W., Sandhu I., Halloran K.M., Grimm P., Schmidt B.M.W., and Halloran P.F.. 2003. Cell senescence in rat kidneys in vivo increases with growth and age despite lack of telomere shortening. Kidney Int. 63:2134–2143. 10.1046/j.1523-1755.2003.00032.x [Abstract] [CrossRef] [Google Scholar]
- Melk A., Schmidt B.M.W., Takeuchi O., Sawitzki B., Rayner D.C., and Halloran P.F.. 2004. Expression of p16INK4a and other cell cycle regulator and senescence associated genes in aging human kidney. Kidney Int. 65:510–520. 10.1111/j.1523-1755.2004.00438.x [Abstract] [CrossRef] [Google Scholar]
- Min H., Montecino-Rodriguez E., and Dorshkind K.. 2005. Effects of aging on early B- and T-cell development. Immunol. Rev. 205:7–17. 10.1111/j.0105-2896.2005.00263.x [Abstract] [CrossRef] [Google Scholar]
- Mitchell S.J., Madrigal-Matute J., Scheibye-Knudsen M., Fang E., Aon M., González-Reyes J.A., Cortassa S., Kaushik S., Gonzalez-Freire M., Patel B., et al. . 2016. Effects of Sex, Strain, and Energy Intake on Hallmarks of Aging in Mice. Cell Metab. 23:1093–1112. 10.1016/j.cmet.2016.05.027 [Europe PMC free article] [Abstract] [CrossRef] [Google Scholar]
- Mocchegiani E., and Malavolta M.. 2004. NK and NKT cell functions in immunosenescence. Aging Cell. 3:177–184. 10.1111/j.1474-9728.2004.00107.x [Abstract] [CrossRef] [Google Scholar]
- Molofsky A. V, Slutsky S.G., Joseph N.M., He S., Pardal R., Krishnamurthy J., Sharpless N.E., and Morrison S.J.. 2006. Increasing p16INK4a expression decreases forebrain progenitors and neurogenesis during ageing. Nature. 443:448–452. [Europe PMC free article] [Abstract] [Google Scholar]
- Muñoz-Espín D., and Serrano M.. 2014. Cellular senescence: from physiology to pathology. Nat. Rev. Mol. Cell Biol. 15:482–496. 10.1038/nrm3823 [Abstract] [CrossRef] [Google Scholar]
- Muñoz-Espín D., Cañamero M., Maraver A., Gómez-López G., Contreras J., Murillo-Cuesta S., Rodríguez-Baeza A., Varela-Nieto I., Ruberte J., Collado M., and Serrano M.. 2013. Programmed cell senescence during mammalian embryonic development. Cell. 155:1104–1118. 10.1016/j.cell.2013.10.019 [Abstract] [CrossRef] [Google Scholar]
- Nalysnyk L., Cid-Ruzafa J., Rotella P., and Esser D.. 2012. Incidence and prevalence of idiopathic pulmonary fibrosis: review of the literature. Eur. Respir. Rev. 21:355–361. 10.1183/09059180.00002512 [Abstract] [CrossRef] [Google Scholar]
- Nandakumar J., and Cech T.R.. 2013. Finding the end: recruitment of telomerase to telomeres. Nat. Rev. Mol. Cell Biol. 14:69–82. 10.1038/nrm3505 [Europe PMC free article] [Abstract] [CrossRef] [Google Scholar]
- Narita M., Young A.R.J., Arakawa S., Samarajiwa S.A., Nakashima T., Yoshida S., Hong S., Berry L.S., Reichelt S., Ferreira M., et al. . 2011. Spatial Coupling of mTOR and Autophagy Augments Secretory Phenotypes. Science. 332:966–970. [Europe PMC free article] [Abstract] [Google Scholar]
- Niccoli T., and Partridge L.. 2012. Ageing as a risk factor for disease. Curr. Biol. 22:R741–R752. 10.1016/j.cub.2012.07.024 [Abstract] [CrossRef] [Google Scholar]
- North B.J., and Sinclair D.A.. 2012. The intersection between aging and cardiovascular disease. Circ. Res. 110:1097–1108. 10.1161/CIRCRESAHA.111.246876 [Europe PMC free article] [Abstract] [CrossRef] [Google Scholar]
- Ogrodnik M., Miwa S., Tchkonia T., Tiniakos D., Wilson C.L., Lahat A., Day C.P., Burt A., Palmer A., Anstee Q.M., et al. . 2017. Cellular senescence drives age-dependent hepatic steatosis. Nat. Commun. 8:15691 10.1038/ncomms15691 [Europe PMC free article] [Abstract] [CrossRef] [Google Scholar]
- Palm W., and de Lange T.. 2008. How shelterin protects mammalian telomeres. Annu. Rev. Genet. 42:301–334. 10.1146/annurev.genet.41.110306.130350 [Abstract] [CrossRef] [Google Scholar]
- Pazolli E., Luo X., Brehm S., Carbery K., Chung J.J., Prior J.L., Doherty J., Demehri S., Salavaggione L., Piwnica-Worms D., and Stewart S.A.. 2009. Senescent stromal-derived osteopontin promotes preneoplastic cell growth. Cancer Res. 69:1230–1239. 10.1158/0008-5472.CAN-08-2970 [Europe PMC free article] [Abstract] [CrossRef] [Google Scholar]
- Pellicoro A., Ramachandran P., Iredale J.P., and Fallowfield J.A.. 2014. Liver fibrosis and repair: immune regulation of wound healing in a solid organ. Nat. Rev. Immunol. 14:181–194. 10.1038/nri3623 [Abstract] [CrossRef] [Google Scholar]
- Pietras E.M., Mirantes-Barbeito C., Fong S., Loeffler D., Kovtonyuk L.V., Zhang S., Lakshminarasimhan R., Chin C.P., Techner J.-M., Will B., et al. . 2016. Chronic interleukin-1 exposure drives haematopoietic stem cells towards precocious myeloid differentiation at the expense of self-renewal. Nat. Cell Biol. 18:607–618. 10.1038/ncb3346 [Europe PMC free article] [Abstract] [CrossRef] [Google Scholar]
- Price J.S., Waters J.G., Darrah C., Pennington C., Edwards D.R., Donell S.T., and Clark I.M.. 2002. The role of chondrocyte senescence in osteoarthritis. Aging Cell. 1:57–65. 10.1046/j.1474-9728.2002.00008.x [Abstract] [CrossRef] [Google Scholar]
- Querfurth H.W., and LaFerla F.M.. 2010. Alzheimer’s disease. N. Engl. J. Med. 362:329–344. 10.1056/NEJMra0909142 [Abstract] [CrossRef] [Google Scholar]
- Raggi C., and Berardi A.C.. 2012. Mesenchymal stem cells, aging and regenerative medicine. Muscles Ligaments Tendons J. 2:239–242. [Europe PMC free article] [Abstract] [Google Scholar]
- Raisz L.G. 1988. Local and systemic factors in the pathogenesis of osteoporosis. N. Engl. J. Med. 318:818–828. 10.1056/NEJM198803313181305 [Abstract] [CrossRef] [Google Scholar]
- Ritschka B., Storer M., Mas A., Heinzmann F., Ortells M.C., Morton J.P., Sansom O.J., Zender L., and Keyes W.M.. 2017. The senescence-associated secretory phenotype induces cellular plasticity and tissue regeneration. Genes Dev. 31:172–183. 10.1101/gad.290635.116 [Europe PMC free article] [Abstract] [CrossRef] [Google Scholar]
- Rodier F., Coppé J.-P., Patil C.K., Hoeijmakers W.A., Muñoz D.P., Raza S.R., Freund A., Campeau E., Davalos A.R., and Campisi J.. 2009. Persistent DNA damage signalling triggers senescence-associated inflammatory cytokine secretion. Nat. Cell Biol. 11:973–979. 10.1038/ncb1909 [Europe PMC free article] [Abstract] [CrossRef] [Google Scholar]
- Rodier F., Muñoz D.P., Teachenor R., Chu V., Le O., Bhaumik D., Coppé J.P., Campeau E., Beauséjour C.M., Kim S.H., et al. . 2011. DNA-SCARS: distinct nuclear structures that sustain damage-induced senescence growth arrest and inflammatory cytokine secretion. J. Cell Sci. 124:68–81. 10.1242/jcs.071340 [Europe PMC free article] [Abstract] [CrossRef] [Google Scholar]
- Rudin C.M., Hann C.L., Garon E.B., Ribeiro de Oliveira M., Bonomi P.D., Camidge D.R., Chu Q., Giaccone G., Khaira D., Ramalingam S.S., et al. . 2012. Phase II study of single-agent navitoclax (ABT-263) and biomarker correlates in patients with relapsed small cell lung cancer. Clin. Cancer Res. 18:3163–3169. 10.1158/1078-0432.CCR-11-3090 [Europe PMC free article] [Abstract] [CrossRef] [Google Scholar]
- Rudolph K.L. 2000. Inhibition of Experimental Liver Cirrhosis in Mice by Telomerase Gene Delivery. Science. 287:1253–1258. [Abstract] [Google Scholar]
- Rudolph K.L., Chang S., Lee H.W., Blasco M., Gottlieb G.J., Greider C., and DePinho R.A.. 1999. Longevity, stress response, and cancer in aging telomerase-deficient mice. Cell. 96:701–712. 10.1016/S0092-8674(00)80580-2 [Abstract] [CrossRef] [Google Scholar]
- Salama R., Sadaie M., Hoare M., and Narita M.. 2014. Cellular senescence and its effector programs. Genes Dev. 28:99–114. 10.1101/gad.235184.113 [Europe PMC free article] [Abstract] [CrossRef] [Google Scholar]
- Saxton R.A., and Sabatini D.M.. 2017. mTOR Signaling in Growth, Metabolism, and Disease. Cell. 168:960–976. (published erratum appears in Cell. 2017. 169: 361–371) 10.1016/j.cell.2017.02.004 [Europe PMC free article] [Abstract] [CrossRef] [Google Scholar]
- Schafer M.J., White T.A., Iijima K., Haak A.J., Ligresti G., Atkinson E.J., Oberg A.L., Birch J., Salmonowicz H., Zhu Y., et al. . 2017. Cellular senescence mediates fibrotic pulmonary disease. Nat. Commun. 8:14532 10.1038/ncomms14532 [Europe PMC free article] [Abstract] [CrossRef] [Google Scholar]
- Selman C., Lingard S., Choudhury A.I., Batterham R.L., Claret M., Clements M., Ramadani F., Okkenhaug K., Schuster E., Blanc E., et al. . 2008. Evidence for lifespan extension and delayed age-related biomarkers in insulin receptor substrate 1 null mice. FASEB J. 22:807–818. 10.1096/fj.07-9261com [Abstract] [CrossRef] [Google Scholar]
- Sharpless N.E., and Sherr C.J.. 2015. Forging a signature of in vivo senescence. Nat. Rev. Cancer. 15:397–408. 10.1038/nrc3960 [Abstract] [CrossRef] [Google Scholar]
- Sharpless N.E., Bardeesy N., Lee K.H., Carrasco D., Castrillon D.H., Aguirre A.J., Wu E.A., Horner J.W., and DePinho R.A.. 2001. Loss of p16Ink4a with retention of p19Arf predisposes mice to tumorigenesis. Nature. 413:86–91. 10.1038/35092592 [Abstract] [CrossRef] [Google Scholar]
- Shay J.W. 2016. Role of telomeres and telomerase in aging and cancer. Cancer Discov. 6:584–593. 10.1158/2159-8290.CD-16-0062 [Europe PMC free article] [Abstract] [CrossRef] [Google Scholar]
- Shefer G., Van de Mark D.P., Richardson J.B., and Yablonka-Reuveni Z.. 2006. Satellite-cell pool size does matter: defining the myogenic potency of aging skeletal muscle. Dev. Biol. 294:50–66. 10.1016/j.ydbio.2006.02.022 [Europe PMC free article] [Abstract] [CrossRef] [Google Scholar]
- Solomon J.M., Pasupuleti R., Xu L., McDonagh T., Curtis R., DiStefano P.S., and Huber L.J.. 2006. Inhibition of SIRT1 catalytic activity increases p53 acetylation but does not alter cell survival following DNA damage. Mol. Cell. Biol. 26:28–38. 10.1128/MCB.26.1.28-38.2006 [Europe PMC free article] [Abstract] [CrossRef] [Google Scholar]
- Sone H., and Kagawa Y.. 2005. Pancreatic beta cell senescence contributes to the pathogenesis of type 2 diabetes in high-fat diet-induced diabetic mice. Diabetologia. 48:58–67. 10.1007/s00125-004-1605-2 [Abstract] [CrossRef] [Google Scholar]
- Sousa-Victor P., Gutarra S., García-Prat L., Rodriguez-Ubreva J., Ortet L., Ruiz-Bonilla V., Jardí M., Ballestar E., González S., Serrano A.L., et al. . 2014. Geriatric muscle stem cells switch reversible quiescence into senescence. Nature. 506:316–321. 10.1038/nature13013 [Abstract] [CrossRef] [Google Scholar]
- Soysal P., Stubbs B., Lucato P., Luchini C., Solmi M., Peluso R., Sergi G., Isik A.T., Manzato E., Maggi S., et al. . 2016. Inflammation and frailty in the elderly: A systematic review and meta-analysis. Ageing Res. Rev. 31:1–8. 10.1016/j.arr.2016.08.006 [Abstract] [CrossRef] [Google Scholar]
- Storer M., Mas A., Robert-Moreno A., Pecoraro M., Ortells M.C., Di Giacomo V., Yosef R., Pilpel N., Krizhanovsky V., Sharpe J., and Keyes W.M.. 2013. Senescence is a developmental mechanism that contributes to embryonic growth and patterning. Cell. 155:1119–1130. 10.1016/j.cell.2013.10.041 [Abstract] [CrossRef] [Google Scholar]
- Sturmlechner I., Durik M., Sieben C.J., Baker D.J., and van Deursen J.M.. 2017. Cellular senescence in renal ageing and disease. Nat. Rev. Nephrol. 13:77–89. 10.1038/nrneph.2016.183 [Abstract] [CrossRef] [Google Scholar]
- Sun N., Youle R.J., and Finkel T.. 2016. The Mitochondrial Basis of Aging. Mol. Cell. 61:654–666. 10.1016/j.molcel.2016.01.028 [Europe PMC free article] [Abstract] [CrossRef] [Google Scholar]
- Tasdemir N., Banito A., Roe J.-S., Alonso-Curbelo D., Camiolo M., Tschaharganeh D.F., Huang C.-H., Aksoy O., Bolden J.E., Chen C.-C., et al. . 2016. BRD4 connects enhancer remodeling to senescence immune surveillance. Cancer Discov. 6:612–629. 10.1158/2159-8290.CD-16-0217 [Europe PMC free article] [Abstract] [CrossRef] [Google Scholar]
- Tasselli L., Xi Y., Zheng W., Tennen R.I., Odrowaz Z., Simeoni F., Li W., and Chua K.F.. 2016. SIRT6 deacetylates H3K18ac at pericentric chromatin to prevent mitotic errors and cellular senescence. Nat. Struct. Mol. Biol. 23:434–440. 10.1038/nsmb.3202 [Europe PMC free article] [Abstract] [CrossRef] [Google Scholar]
- Tchkonia T., Morbeck D.E., Von Zglinicki T., Van Deursen J., Lustgarten J., Scrable H., Khosla S., Jensen M.D., and Kirkland J.L.. 2010. Fat tissue, aging, and cellular senescence. Aging Cell. 9:667–684. 10.1111/j.1474-9726.2010.00608.x [Europe PMC free article] [Abstract] [CrossRef] [Google Scholar]
- Tilstra J.S., Robinson A.R., Wang J., Gregg S.Q., Clauson C.L., Reay D.P., Nasto L.A., St Croix C.M., Usas A., Vo N., et al. . 2012. NF-κB inhibition delays DNA damage-induced senescence and aging in mice. J. Clin. Invest. 122:2601–2612. 10.1172/JCI45785 [Europe PMC free article] [Abstract] [CrossRef] [Google Scholar]
- Uryga A.K., and Bennett M.R.. 2016. Ageing induced vascular smooth muscle cell senescence in atherosclerosis. J. Physiol. 594:2115–2124. [Abstract] [Google Scholar]
- Varela I., Cadinanos J., Pendas A.M., Gutierrez-Fernandez A., Folgueras A.R., Sanchez L.M., Zhou Z., Rodriguez F.J., Stewart C.L., Vega J.A., et al. . 2005. Accelerated ageing in mice deficient in Zmpste24 protease is linked to p53 signalling activation. Nature. 437:564–568. [Abstract] [Google Scholar]
- Wajapeyee N., Serra R.W., Zhu X., Mahalingam M., and Green M.R.. 2008. Oncogenic BRAF induces senescence and apoptosis through pathways mediated by the secreted protein IGFBP7. Cell. 132:363–374. 10.1016/j.cell.2007.12.032 [Europe PMC free article] [Abstract] [CrossRef] [Google Scholar]
- Wiemann S.U., Satyanarayana A., Tsahuridu M., Tillmann H.L., Zender L., Klempnauer J., Flemming P., Franco S., Blasco M.A., Manns M.P., and Rudolph K.L.. 2002. Hepatocyte telomere shortening and senescence are general markers of human liver cirrhosis. FASEB J. 16:935–942. 10.1096/fj.01-0977com [Abstract] [CrossRef] [Google Scholar]
- Xu M., Palmer A.K., Ding H., Weivoda M.M., Pirtskhalava T., White T.A., Sepe A., Johnson K.O., Stout M.B., Giorgadze N., et al. . 2015. Targeting senescent cells enhances adipogenesis and metabolic function in old age. eLife. 4:e12997 10.7554/eLife.12997 [Europe PMC free article] [Abstract] [CrossRef] [Google Scholar]
- Xue W., Zender L., Miething C., Dickins R.A., Hernando E., Krizhanovsky V., Cordon-Cardo C., and Lowe S.W.. 2007. Senescence and tumour clearance is triggered by p53 restoration in murine liver carcinomas. Nature. 445:656–660. 10.1038/nature05529 [Europe PMC free article] [Abstract] [CrossRef] [Google Scholar]
- Yamakoshi K., Takahashi A., Hirota F., Nakayama R., Ishimaru N., Kubo Y., Mann D.J., Ohmura M., Hirao A., Saya H., et al. . 2009. Real-time in vivo imaging of p16Ink4a reveals cross talk with p53. J. Cell Biol. 186:393–407. 10.1083/jcb.200904105 [Europe PMC free article] [Abstract] [CrossRef] [Google Scholar]
- Yanai H., Shteinberg A., Porat Z., Budovsky A., Braiman A., Ziesche R., and Fraifeld V.E.. 2015. Cellular senescence-like features of lung fibroblasts derived from idiopathic pulmonary fibrosis patients. Aging (Albany NY). 7:664–672. 10.18632/aging.100807 [Europe PMC free article] [Abstract] [CrossRef] [Google Scholar]
- Yap K.L., Li S., Muñoz-Cabello A.M., Raguz S., Zeng L., Mujtaba S., Gil J., Walsh M.J., and Zhou M.M.. 2010. Molecular interplay of the noncoding RNA ANRIL and methylated histone H3 lysine 27 by polycomb CBX7 in transcriptional silencing of INK4a. Mol. Cell. 38:662–674. 10.1016/j.molcel.2010.03.021 [Europe PMC free article] [Abstract] [CrossRef] [Google Scholar]
- Yosef R., Pilpel N., Tokarsky-Amiel R., Biran A., Ovadya Y., Cohen S., Vadai E., Dassa L., Shahar E., Condiotti R., et al. . 2016. Directed elimination of senescent cells by inhibition of BCL-W and BCL-XL. Nat. Commun. 7:11190 10.1038/ncomms11190 [Europe PMC free article] [Abstract] [CrossRef] [Google Scholar]
- Zeggini E., Weedon M.N., Lindgren C.M., Frayling T.M., Elliott K.S., Lango H., Timpson N.J., Perry J.R.B., Rayner N.W., Freathy R.M., et al. Wellcome Trust Case Control Consortium (WTCCC) . 2007. Replication of genome-wide association signals in UK samples reveals risk loci for type 2 diabetes. Science. 316:1336–1341. 10.1126/science.1142364 [Europe PMC free article] [Abstract] [CrossRef] [Google Scholar]
Articles from The Journal of Cell Biology are provided here courtesy of The Rockefeller University Press
Full text links
Read article at publisher's site: https://doi.org/10.1083/jcb.201708092
Read article for free, from open access legal sources, via Unpaywall:
https://rupress.org/jcb/article-pdf/217/1/65/1378290/jcb_201708092.pdf
Citations & impact
Impact metrics
Article citations
The role of the dynamic epigenetic landscape in senescence: orchestrating SASP expression.
NPJ Aging, 10(1):48, 24 Oct 2024
Cited by: 0 articles | PMID: 39448585 | PMCID: PMC11502686
Review Free full text in Europe PMC
Cell senescence in cardiometabolic diseases.
NPJ Aging, 10(1):46, 21 Oct 2024
Cited by: 0 articles | PMID: 39433786 | PMCID: PMC11493982
Review Free full text in Europe PMC
D-galactose Induces Senescence in Adult Mouse Neural Stem Cells by Imbalanced Oxidant and Antioxidant Activity and Differential Expression of Specific Hub Genes.
Mol Neurobiol, 19 Oct 2024
Cited by: 0 articles | PMID: 39425831
Endoplasmic Reticulum Stress in Bronchopulmonary Dysplasia: Contributor or Consequence?
Cells, 13(21):1774, 26 Oct 2024
Cited by: 0 articles | PMID: 39513884 | PMCID: PMC11544778
Review Free full text in Europe PMC
Regulation of CD8+ T cells by lipid metabolism in cancer progression.
Cell Mol Immunol, 21(11):1215-1230, 14 Oct 2024
Cited by: 0 articles | PMID: 39402302 | PMCID: PMC11527989
Review Free full text in Europe PMC
Go to all (518) article citations
Other citations
Data
Data behind the article
This data has been text mined from the article, or deposited into data resources.
BioStudies: supplemental material and supporting data
Similar Articles
To arrive at the top five similar articles we use a word-weighted algorithm to compare words from the Title and Abstract of each citation.
Cellular senescence in aging and osteoarthritis.
Acta Orthop, 87(sup363):6-14, 23 Sep 2016
Cited by: 53 articles | PMID: 27658487 | PMCID: PMC5389431
Review Free full text in Europe PMC
Noncoding RNAs Controlling Telomere Homeostasis in Senescence and Aging.
Trends Mol Med, 26(4):422-433, 28 Feb 2020
Cited by: 20 articles | PMID: 32277935 | PMCID: PMC7152597
Review Free full text in Europe PMC
The Impact of Exercise on Telomere Length, DNA Methylation and Metabolic Footprints.
Cells, 11(1):153, 04 Jan 2022
Cited by: 6 articles | PMID: 35011715 | PMCID: PMC8750279
Review Free full text in Europe PMC
From cells to organisms: can we learn about aging from cells in culture?
Exp Gerontol, 36(4-6):607-618, 01 Apr 2001
Cited by: 166 articles | PMID: 11295503
Review
Funding
Funders who supported this work.
Medical Research Council (4)
Grant ID: 1609078
Grant ID: MC-A652-5PZ00
Grant ID: MC-A652-5PZ00
Senescence
Dr Jesus Gil, MRC London Institute of Medical Sciences
Grant ID: MC_U120085810