Abstract
Free full text

Regulation of Macrophage Immunometabolism in Atherosclerosis
Abstract
Cells of the myeloid lineage undergo robust metabolic transitions after activation, as well as discrete epigenetic changes, that can dictate both ongoing and future inflammatory responses. In atherosclerosis, where macrophages play central roles in the initiation, growth and ultimately, rupture of arterial plaques, altered metabolism is a key feature that dictates macrophage function and subsequent disease progression. This review explores how factors central to the plaque’s microenvironment (e.g., altered cholesterol metabolism, oxidative stress, hypoxia, apoptotic and necrotic cells, and hyperglycemia) shape the metabolic rewiring of macrophages in atherosclerosis, and how these metabolic shifts, in turn, alter macrophage immune effector and tissue reparative functions. Finally, this overview offers insight into the challenges and opportunities of harnessing metabolism to modulate aberrant macrophage responses in disease.
Introduction
Macrophages are essential components of mammalian tissues, and are strategically positioned throughout the body to maintain tissue homeostasis and act as immune sentinels. It is now appreciated that macrophages can be either embryonically seeded in tissues where they are maintained through self-renewal, or derived from monocyte precursors that infiltrate tissues and differentiate in response to their microenvironment. However, regardless of their origin, macrophages exhibit remarkable plasticity and can change their functional phenotype in response to local environmental cues. Macrophages execute a diverse set of functions essential to host defense and tissue repair, including phagocytosis of apoptotic cells and pathogens, elaboration of immune effector molecules and growth factors for other cell types in tissues, and remodeling of the extracellular matrix. In order to fulfill these effector functions, macrophages must coordinate a plethora of intrinsic cellular processes to meet environmental demand. It is becoming increasingly appreciated that central to this intrinsic coordination is the rewiring of metabolic pathways and the repurposing of metabolic intermediates to facilitate appropriate extrinsic cellular responses.
Early studies of metabolic reprogramming in cancer cells provided a window into the understanding of how metabolism can regulate cell fate. Elevated uptake of glucose and secretion of lactate are metabolic hallmarks of highly proliferative tumor cells, which rely heavily on glycolysis even when sufficient oxygen is present to support mitochondrial oxidative phosphorylation – so called ‘aerobic glycolysis’ or the ‘Warburg effect’1. While aerobic glycolysis is less efficient at generating ATP than oxidative phosphorylation (2 vs 32 molecules of ATP/ molecule of glucose), this metabolic pathway is nonetheless well suited for proliferation as it allows metabolic intermediates to be siphoned off for biosynthesis of nucleotides, lipids and proteins needed in the dividing cell. Similar metabolic alterations have now been identified in some immune cells, including macrophages, as they transition from rested to activated states1. It is now appreciated that metabolic reprogramming of immune cells is not only critical for energy homeostasis, but can directly influence immune cell differentiation and function.
Initiation and perpetuation of immune responses to external cues requires transformation of immune cells from a relatively quiescent to a highly active state, which necessitates an appropriate metabolic shift to sustain the needs of activation. Metabolic machinery of macrophages can be specifically modulated to match cellular demand to external stimuli, such as phagocytosis, proliferation, and cytokine production2,3. This metabolic modulation can result in energy generation, building block production for cell maintenance/proliferation, and modulation of cellular signaling4. For example, the transformation of macrophages to either classically [stimulated with lipopolysaccharide (LPS) and interferon-gamma (IFN-γ)] or alternatively [stimulated with interleukin (IL)-4] activated phenotypes requires aligning of metabolism to anabolic or catabolic processes, respectively. In general, classically activated cells rely on anabolic metabolism for balancing energy needs with high rates of glycolysis and the need for macromolecular building blocks with an active (albeit partially broken) mitochondrial tricarboxylic acid cycle (TCA) cycle5. Alternatively activated macrophages, on the other hand, channel degraded nutrients through oxidative phosphorylation for efficient ATP production5,6. These in vitro phenotypes have been useful models to study, however, considerably less is known about how macrophage metabolism changes in vivo, where cells encounter a multitude of signals in the tissue microenvironment during either pathogen challenge or sterile inflammatory responses.
During atherosclerosis, sterile inflammation is set in motion by the intramural retention of cholesterol-rich lipoproteins in large and medium-sized arteries (reviewed in 7). Imbalances in cellular and systemic cholesterol homeostasis promote the deposition of apoB-lipoproteins in the artery wall, particularly in areas of altered blood flow that disturb the normally quiescent endothelium. These sequestered lipoproteins are susceptible to various modifications (e.g., oxidation, enzymatic cleavage, aggregation) that render them pro-inflammatory, triggering the recruitment of monocyte-derived cells into the subendothelial space. There, monocytes differentiate into macrophages that clear the accumulated lipoproteins, which although initially beneficial, ultimately results in the accumulation of cholesterol-laden macrophage foam cells that promote plaque formation. These macrophage foam cells have a diminished migratory capacity8,9 and elicit pro-inflammatory cytokines and chemokines that amplify the immune response by recruiting reinforcements, including additional monocytes, T cells, and neutrophils (reviewed in 10). For reasons that remain poorly understood, the resulting low-grade inflammation is non-resolving and plaque progression can continue over decades of life11. As plaques advance, macrophages proliferate12, secrete inflammatory mediators (e.g., cytokines and proteases), and ultimately die from lipid- and metabolic-stresses13, thereby releasing their lipid contents and other inflammatory debris that contributes to the formation of a necrotic core. In the plaque, macrophages are exposed to a variety of pro-inflammatory cytokines, oxidized lipids, cholesterol crystals, oxidative stress, and danger-associated molecular patterns (DAMPs) derived from dying cells that create a complex microenvironment that perpetuates macrophage inflammation7.
The progression of atherosclerosis is influenced by risk factors such as smoking, hypertension, dyslipidemia, sedentary lifestyle, and diabetes. Despite optimal medical treatment with anti-hypertensive and cholesterol lowering drugs (e.g., statins), there remains a significant residual cardiovascular risk14. There is, thus, significant interest in targeting the inflammatory component of atherosclerosis, and developing strategies to alter the inflammatory phenotype of plaque macrophages10,15. The encouraging finding, in a recent large clinical trial, that antibody-mediated inhibition of IL-1β can reduce cardiovascular events in high risk populations16, has provided optimism that such a strategy would work. This has fueled interest in understanding the immunometabolic pathways that influence macrophage inflammatory responses during atherosclerosis, and whether these in turn, might represent new therapeutic targets for intervention. Recent studies have begun to elucidate how macrophage metabolism of glucose, cholesterol, fatty acids and amino acids is rewired in atherosclerosis, and how these changes shape both ongoing and future inflammatory responses. It is already clear that the complex microenvironment of the plaque results in a variety of activation modes in macrophages and, in turn, a more complex and heterogenous rewiring of metabolic pathways than seen in classically or alternatively activated macrophage phenotypes.
This review will provide a framework for understanding the metabolic programs that influence macrophage activation and inflammatory phenotypes, how these programs are impacted by microenvironmental factors in the atherosclerotic plaque, the role of metabolism in epigenetic programming of future inflammatory responses, and whether these various pathways can be targeted for therapeutic intervention in atherosclerosis.
An Overview of Cellular Metabolic Programs
Overview of cellular metabolic programs
Metabolic pathways play a major role in immune cell function. The intertwined pathways of glycolysis, the tricarboxylic acid (TCA) cycle, the pentose phosphate pathway, fatty acid oxidation, fatty acid synthesis and amino acid metabolism serve to generate energy, produce building blocks necessary for cellular maintenance and proliferation, and modulate cellular signalling5. Below we provide a brief description of these pathways, how they are interconnected, and how they intersect in regulating macrophage activation and effector function.
Glucose Utilization, Glycolysis and Pentose Phosphate Pathway
In macrophages, the glucose transporter-1 (GLUT-1) initiates the uptake of glucose, which is then phosphorylated by hexokinase to glucose-6-phosphate (G6P), to be further metabolized in glycolysis or in the pentose phosphate pathway (PPP) (reviewed in 5). During glycolysis, processing of glucose occurs in the cytosol, yielding 2 net ATP alongside pyruvate, which is either converted by lactate dehydrogenase to lactate or enters the TCA cycle. Glycolysis is a comparably inefficient way of generating ATP compared to the TCA cycle, which nets 32 ATPs from the oxidation of glucose; however, it produces ATP rapidly, and can provide biosynthetic intermediates that fuel the PPP and fatty acid synthesis. The final glycolytic enzyme, pyruvate kinase (PKM1/PKM2), regulates glycolytic flux by alternating between catalytically active tetrameric or slow dimeric states, which regulate funneling of earlier glycolytic intermediates into the PPP, or for use in serine synthesis17.
The PPP is an anabolic pathway that runs parallel to glycolysis and generates ribose-5-phosphate for synthesis of nucleotides, and NADPH, an essential cofactor in lipid biosynthesis and the production of anti-oxidants, nitric oxide and reactive oxygen species (ROS) (reviewed in 5). Macrophages require high levels of PPP flux and NADPH during respiratory bursts to both eliminate extracellular bacteria and to synthesize antioxidants such as glutathione and thioredoxin, which limit oxidative damage to the cell. By contrast, PPP flux is limited in alternatively activated macrophages18, which perform tissue reparative functions and produce less ROS. PPP-derived NADPH is also used for de novo fatty acid synthesis to expand the endoplasmic reticulum (ER) and Golgi to support enhanced cytokine secretion.
The TCA Cycle and Oxidative Phosphorylation
Oxidative phosphorylation diverges from glycolysis after pyruvate production, where pyruvate is shuttled to the mitochondria and enters the TCA cycle (reviewed in 5). The TCA cycle converts either pyruvate or fatty acids into acetyl coenzyme A (acetyl-CoA), which is condensed with oxaloacetate to form citrate. This important intermediate is then either further oxidized by the TCA cycle or hydrolyzed by ATP-citrate lyase (ACLY) to generate oxaloacetate and cytosolic acetyl-coA, which contributes to protein acetylation and the synthesis of cholesterol and fatty acids needed for new membranes. The TCA cycle produces both NADH and Flavin adenine dinucleotide (FADH2), which transfers electrons to the electron transport chain to support oxidative phosphorylation yielding ATP. The TCA cycle and oxidative phosphorylation are more often associated with quiescent or non-proliferative cells.
Fatty Acid Metabolism: Oxidation and Synthesis
Fatty acid oxidation is the most fruitful producer of cellular ATP, where a single fatty acid molecule such as palmitate can yield over 100 molecules of ATP5. Short chain fatty acids can diffuse passively into the mitochondria for oxidation, whereas medium and long chain fatty acids are imported into the mitochondria by conjugation to carnitine palmitoyltransferase 1 (CPT1). Fatty acid oxidation yields a number of products including acetyl-CoA, NADH and FADH2, which can used by the TCA cycle and electron transport chain to generate ATP. Conversely, fatty acid synthesis utilizes products derived from other metabolic pathways, including glycolysis, the TCA cycle and PPP, to provide lipids necessary for differentiation and proliferation19,20. Fatty acid synthesis involves the activity of enzymes including sterol regulatory element binding protein (SREBP), fatty acid synthase (FASN) and acetyl CoA carboxylase (ACC) to convert metabolic intermediates, such as the TCA cycle-derived citrate or glycolysis-derived glycerol, into triacylglycerols and phospholipids, which are key components of cellular structures.
Amino Acid Metabolism
Metabolism of amino acids can have important roles in cell effector functions. For example, glutamine feeds the TCA cycle where it can either be used for ATP production or as a source of citrate for fatty acid synthesis, while arginine and tryptophan can be metabolized to support cellular proliferation and anabolic growth. Indeed, metabolism of arginine by either inducible nitric oxide synthase (iNOS) or arginase-1 was initially used to define classically and alternatively activated macrophage subsets, respectively21.
The Metabolic Phenotypes of Acutely Activated Macrophages
The aforementioned metabolic pathways are intricately dependent on one another during macrophage activation to synergistically fulfill cell effector functions. For many years, in vitro and in vivo activation of macrophages, and the dissection of their metabolic and effector responses, focused on two activation phenotypes: “M1” macrophages classically activated by LPS and IFN-γ stimulation and alternatively activated “M2” macrophages activated by IL-4 stimulation (hereafter referred to as M[LPS+IFNγ] and M[IL-4]. While it has become clear that these macrophage phenotypes represent extremes, their mechanistic exploration provides a starting ground for understanding how macrophage activation and metabolism intersect to dictate cellular responses (Figure 1).
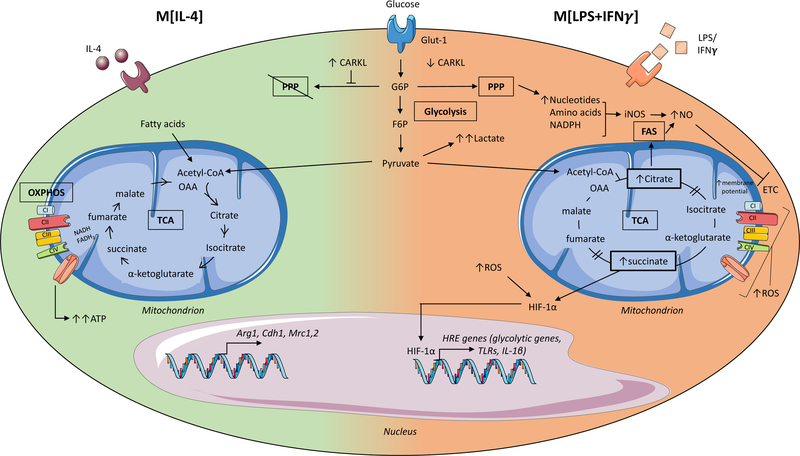
Glycolysis involves the conversion of glucose molecules into various metabolic byproducts, culminating in the end product pyruvate as well as 2 net ATP. In macrophages treated with interleukin (IL)-4, (M[IL-4], left side of the cell) pyruvate (and fatty acids) enters the intact tricarboxylic acid (TCA) cycle, as acetyl coenzyme A (Acetyl-CoA), resulting in sustained ATP production via oxidative phosphorylation (OXPHOS) and leading to the upregulation of genes associated with tissue repair. Conversely, in macrophages treated with lipopolysaccharide (LPS) and interferon-gamma (IFN-γ) (M[LPS+IFN-γ] right side of the cell) the majority of pyruvate is converted into lactate and secreted. Furthermore, the enzyme carbohydrate kinase-like protein (CARKL) is downregulated, and as a result, glycolysis also feeds the pentose phosphate pathway (PPP) generating nucleotides, amino acids and NADPH. The TCA cycle is broken in two places in M[LPS+IFN-γ] macrophages resulting in the accumulation of citrate that is used to drive fatty acid synthesis (FAS) and succinate that stabilizes the transcription factor hypoxia-inducible factor-1-alpha (HIF-1α). HIF-1α enters the nucleus and promotes the expression of hypoxia response element (HRE) containing genes, which include both glycolytic and pro-inflammatory genes such as interleukin-1beta (IL-1β). The disturbed TCA cycle and reduced oxidative phosphorylation (OXPHOS) also result in increased levels of reactive oxygen species (ROS), which further stabilize HIF-1α to drive glycolysis and inflammatory gene expression. Glut-1, glucose transporter-1; G6P, glucose-6-phosphate; F6P, fructose-6-phosphate; OAA, oxaloacetate; NADPH, Nicotinamide adenine dinucleotide phosphate.
Classically activated macrophages
LPS induces a profound metabolic rewiring of macrophages and dendritic cells characterized by increased glucose uptake and enhanced aerobic glycolysis, coincident with impaired oxidative phosphorylation via the TCA cycle22,23, that resembles the ‘Warburg effect’ of highly proliferative tumor cells1. In these cells, pyruvate produced by the glycolytic pathway is metabolized to lactate and secreted, while TCA cycle intermediates accumulate, resulting in reduced oxidative phosphorylation and direct effects on immune responses23,24. For example, succinate accumulation leads to the stabilization of the hypoxia-inducible factor-1α (HIF-1α) transcription factor and upregulation of enzymes involved in glycolysis and the pro-inflammatory cytokine IL-1β23. Citrate is shuttled to the cytosol where it is used to generate fatty acids for membrane biogenesis and prostaglandin production25, and to produce the antimicrobial metabolite itaconic acid26. Another TCA cycle intermediate, fumarate, contributes to macrophage “innate immune memory” by modifying epigenetic remodeling by KDM5 demethylases27, influencing future immune responses as discussed later in this review.
The PPP is enhanced in M[LPS+IFNγ] macrophages through increased flux of glucose intermediates through the pathway, and downregulation of CARKL18, allowing for increased NADPH synthesis. NADPH availability from the PPP is essential for cholesterol metabolism and fatty acid synthesis28,29, which supports phagocytosis19,20, and the expansion of the Golgi and ER required for production of inflammatory cytokines30,31. M[LPS+IFNγ] also exhibit increased lipid accumulation via enhanced fatty acid uptake via CD36 and diminished triglyceride lipolysis29. In addition, amino acid metabolism modulates immune cell functions in M[LPS+IFNγ] macrophages: glutamine is required for LPS-induced IL-1β secretion32, while arginine is metabolized by iNOS to produce NO which acts both as an anti-microbial agent and as a signal for vasodilation, angiogenesis, and insulin secretion. The extent to which other metabolites can alter the functional state of the macrophage is only beginning to be appreciated.
Alternatively Activated Macrophages
Compared to M[LPS+IFNγ] macrophages, the metabolic phenotype of M[IL-4] macrophages is less well understood. M[IL-4] macrophages see a precipitous increase in fatty acid oxidation (FAO) and oxidative phosphorylation that is thought to contribute to anti-inflammatory responses24. CD36-mediated uptake of triglycerides and subsequent lipolysis via lysosomal lipase feeds the TCA cycle and increases oxidative phosphorylation to sustain M[IL-4] polarization6. The specific role of FAO in M[IL-4] macrophages, however, has recently been questioned by studies showing that etomoxir-mediated inhibition of FAO or deficiency of CPT2 required for fatty acid import did not alter the M[IL-4] phenotype33,34. Notably, M[IL-4] macrophages do depend on glucose to drive oxidative phosphorylation24, as inhibition of glycolysis suppressed IL-4-induced gene expression35, however, the downregulation of the PPP limits glucose flux into this pathway18. In terms of amino acid metabolism, glutamine flux into both the TCA cycle and hexosamine pathway promotes M[IL-4] macrophage polarization24. Furthermore, arginine metabolism by arginase-1 produces L-ornithine, which is broken down to produce polyamines and L-proline, to support macrophage growth or division, and also to provide essential building blocks for collagen production used in wound healing and tissue repair36,37.
Beyond M[LPS+IFNγ] and M[IL-4] Metabolic Responses
Recent studies have revealed that the metabolic rewiring of M[LPS+IFNγ] and M[IL-4] macrophages does not represent a metabolic blueprint for all activated macrophages. Different microbial stimuli, endogenous danger ligands, and tissue microenvironmental signals lead to specific and complex metabolic rewiring of macrophages. As these unique metabolic signatures of macrophages are unraveled, it has become clear that the type and fate of nutrients used are linked not only to the macrophage’s need for energy, but also for its generation of metabolites that act as signaling molecules and building blocks (e.g., lipids and nucleic acids) needed for cellular maintenance or proliferation.
Studies using agonists of TLRs other than TLR4 or whole microbial lysates, suggest that the ‘Warburg’ metabolism of M[LPS+IFNγ] macrophages is not a universal response to pathogenic stimuli2. In vitro stimulation of human CD14+ monocytes with TLR2 (Pam3Cys or P3C), TLR3 (poly (I:C)), or whole pathogen lysates from Escherichia coli, Staphylococcus aureus or Mycobacterium tuberculosis induced increases in glycolysis and lactate production2. These changes were accompanied by increased production of cytokines, such as tumor necrosis factor alpha (TNFα), IL-1β, IL-6 and IL-10, although the degree of induction varied across stimuli. Interestingly, OXPHOS, as measured by oxygen consumption rate (OCR) and spare respiratory capacity (SRC), was increased in TLR2, TLR3, E. coli, S. aureus and M. tuberculosis stimulated macrophages. These metabolic responses were connected to specific functional activities; blocking glycolysis with 2-DG restricted cytokine production (TNFα, IL1β, IL-6) in response to LPS and P3C, while blocking OXPHOS with rotenone lowered cytokine production and phagocytosis only in P3C stimulated cells.
Cytokine stimulation of monocytes and macrophages also induces distinct metabolic rewiring, that is only beginning to be understood. As described for IL-4, IL-13 stimulation, which also polarizes macrophages towards alternative activation, increases mitochondrial biogenesis, fatty acid oxidation and oxidative phosphorylation38,39. Stimulation of macrophages with the myeloid growth factors M-CSF or GM-CSF increased 3H-2-deoxyglucose uptake and OCR. Interestingly in mouse peritoneal macrophages, M-CSF was a stronger inducer of glycolytic extracellular acidification rate (ECAR) and lactate production than GM-CSF, whereas, the opposite was true in human monocyte-derived40 and murine bone marrow-derived macrophages41. The functional consequences of M-CSF- and GM-CSF-induced macrophage metabolism on cytokine production, phagocytosis, and cell migration remain to be determined. Interestingly, in human monocytes, IFNγ alone can enhance glycolysis and lactate production42, yet stimulation of peritoneal macrophages with IFNγ in combination with other cytokines (e.g., TNFα39 or TNFα+IL1β43) did not alter 3H-2-deoxyglucose uptake. Additionally, IL-10 can promote anti-inflammatory effects by suppressing mTOR44, regulating GLUT-1 translocation to inhibit glycolytic flux and promoting mitophagy of dysfunctional mitochondria to help preserve respiratory capacity.
The divergent macrophage effector responses to in vitro TLR, pathogen, and cytokine stimuli described above, highlight that macrophage cellular responses to environmental cues likely require different functional outputs accomplished through specific metabolic rewiring. While these studies of macrophage metabolic responses in vitro have led to an appreciation of the versatility and extent to which metabolic rewiring regulates inflammatory function of these cells, macrophages in vivo are exposed to multiple stimuli simultaneously and must mount a coordinated response to balance a multitude of cellular demands – each dependent on specific energy and metabolic intermediate requirements. In the next section, we discuss how macrophage metabolism is dynamically regulated within the multifaceted microenvironment of the atherosclerotic plaque. Specifically, we consider how priming of macrophage precursors by the systemic atherosclerotic milieu may alter cell function prior to recruitment to the plaque, and how plaque microenvironmental stimuli such as lipoproteins, cholesterol, hypoxia and oxidative stress, apoptotic and necrotic cell death, and hyperglycemia may regulate macrophage metabolism within this dynamic microenvironment (Figure 2).
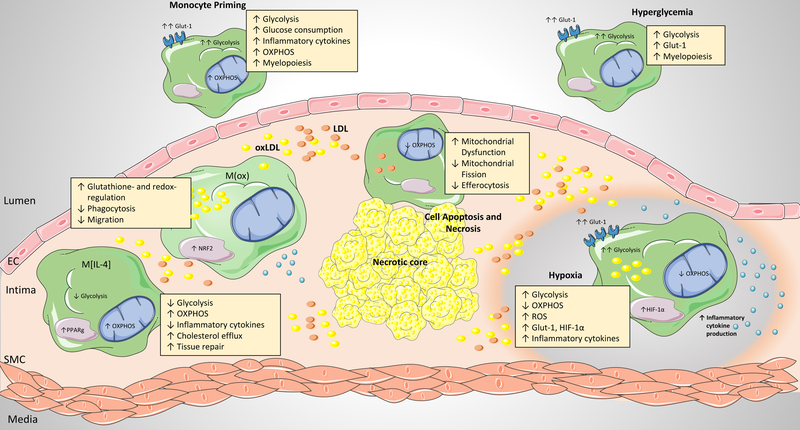
Prior to recruitment to the atherosclerotic plaque, monocytes exhibit a ‘primed’ inflammatory phenotype, characterized by enhanced inflammatory responses that correspond to increases in both glycolysis and oxidative phosphorylation. Within the plaque microenvironment, a variety of stimuli including lipids, cytokines, cell apoptosis and necrosis, hypoxia, and hyperglycemia can all regulate macrophage metabolic reprogramming and subsequent function. Distinct macrophage phenotypes have been identified within the plaque, including M[IL-4]-like, M(Ox), M[LPS+IFNg]-like, and hypoxia-associated macrophages. These different flavors of macrophages are each associated with distinct metabolic signatures and inflammatory functions. PPP, pentose phosphate pathway; FAO, fatty acid oxidation; TCA, tricarboxylic acid cycle, OXPHOS, oxidative phosphorylation; GLUT-1, glucose transporter-1; HIF-1α, hypoxia-inducible factor-1-alpha; PPARγ, Peroxisome proliferator-activated receptor gamma; NRF2, nuclear factor 2
Modulation of Hematopoietic and Myeloid Precursor Cell Metabolism in Atherosclerosis
Monocyte Priming in Hematopoietic Reservoirs and the Circulation
The signals that myeloid cells experience in vivo as they transit from hematopoietic reservoirs into different tissues, where they undergo differentiation into macrophages in unique microenvironments, are complex. Monocytes, derived from either reservoir populations in the bone marrow and spleen, and released into the circulation, are the major precursors of the atherosclerotic plaque macrophage population (reviewed in 7). Prior to their arrival in the artery wall, these monocytes are exposed to a variety of pathologic stimuli within the systemic milieu. Evidence from studies of monocytes isolated from human atherosclerotic patients and mouse models of atherosclerosis indicate that this exposure modulates both cellular metabolism and function, which primes monocytes toward an inflammatory phenotype well before their differentiation within the plaque microenvironment.
Levels of monocytes in the circulation, particularly those of the CD14+ subpopulation in humans and the Ly6C high subpopulation in mice, are strongly correlated with atherosclerosis progression10. Multiple factors in the atherosclerotic systemic milieu have been shown to drive an increase in myelopoiesis, including cholesterol homeostasis45, chronic inflammation, sympathetic nervous system activation46,47, and defective cholesterol efflux48. Glucose uptake is thought to contribute to enhanced myelopoiesis, as inhibition of GLUT-1 in mice lacking the cholesterol efflux transporters ABCA1 and ABCG1, or overexpression of ApoA1, which increases high density lipoprotein (HDL) levels and decreases GLUT-1 expression, both reduce myleoproliferation49. Enhanced glucose uptake by monocytes and hematopoietic precursor cells has been observed in mouse models of atherosclerosis. Using a transplant approach in which atherosclerosis susceptible Apoe–/– mice were reconstituted with either wild type or Apoe–/– bone marrow, Sarrazy et al50 showed enhanced uptake of 2-deoxy [14C] glucose in the bone marrow, spleen and plaque of Apoe–/– bone marrow transplanted mice. Within the bone marrow compartment, mice with Apoe–/– bone marrow had increased glucose uptake by multipotent progenitors, granulocytic-macrophage progenitors (GMPs), as well as increased GLUT-1 expression and uptake of the fluorescent D-glucose analog 2-NBDG by hematopoietic stem and progenitor cells (HSPCs). These alterations in glucose utilization were accompanied by enhanced oxygen consumption and increased mitochondrial membrane potential. Mechanistically, it was determined that the upregulation of GLUT-1 was dependent on IL-3Rβ signaling, which drove subsequent glycolytic substrate utilization by the mitochondria and accelerated myelopoiesis in the Apoe–/– mice.
These metabolic alterations also extend to monocytes isolated from atherosclerotic patients. In a study comparing human monocytes isolated from healthy individuals or individuals with atherosclerosis, Shirai et al51 mechanistically explored the concept of ‘monocyte priming’ in relation to cellular metabolic status. Following ex vivo stimulation with LPS and IFNγ, monocytes isolated from atherosclerotic patients were found to produce more IL-6 and IL-1β compared to monocytes from control individuals, and this “primed” phenotype was maintained after their in vitro differentiation into macrophages. Accompanying these differences, LPS+IFNγ-stimulated monocytes from atherosclerotic patients exhibited increased OCR and respiratory reserve capacity, as well as increased ECAR and glycolytic flux, compared to monocytes from healthy individuals. Notably, the enhanced glucose uptake of atherosclerotic monocytes was shown to be proportional to mitochondrial ROS production, oxidative stress, and subsequent inflammatory signaling. Molecular dissection of the underlying mechanisms identified a glucose-ROS-PKM2-STAT3 pathway through which glucose utilization led to unbalanced ROS generation from the mitochondrial respiratory chain, which in turn induced the redox sensitive enzyme PKM2 (one of the isoforms of the enzyme that catalyzes the final step of glycolysis and converts phosphoenolpyruvate (PEP) to pyruvate) to translocate from the cytoplasm to the nucleus, resulting in STAT3 activation and IL6 and IL1B transcription.
Cumulatively, the preclinical and clinical evidence to date provides direct evidence that the atherosclerotic systemic milieu primes both reservoir and circulating hematopoietic myeloid populations prior to recruitment to the plaque microenvironment. Specifically, these cells utilize more glucose, have enhanced rates of glycolysis and oxidative phosphorylation and are hyperinflammatory prior to recruitment to the plaque.
Microenvironmental Cues That Shape Macrophage Metabolism and Function in The Plaque
In atherosclerotic vessels, as in other tissue sites, distinct microenvironmental factors influence macrophage metabolism and phenotype, and these forces change as the plaque evolves and progresses. As described below, the environment of the atherosclerotic plaque is influenced by hyperlipidemia, oxidative stress, hypoxia, and cell death. In addition, in patients with diabetes, a co-morbid condition that accelerates atherosclerosis, hyperglycemia is also a major factor influencing macrophage metabolism. Together, these microenvironmental signals are important drivers of the metabolic reprogramming of macrophages, and of their inflammatory responses, which ultimately impact disease progression and plaque stability. Below, we summarize in vivo evidence and its support by in vitro mechanistic work, of plaque microenvironmental features that drive changes in macrophage function alongside metabolic adaptations to facilitate cellular effector response in atherosclerosis.
Macrophage uptake of modified lipoproteins and resulting phenotypes
Monocyte-derived and resident tissue macrophage populations lead the response to lipoproteins that are retained and modified in the artery wall. These macrophages attempt to clear the inflammatory lipids, and are transformed into “foamy” macrophages as they store the excess cholesterol and triglyceride in cytoplasmic lipid droplets. Cholesterol loading of macrophages diminishes their migratory capacity, and for reasons that are still unclear, these macrophage foam cells persist in the artery wall, fostering chronic inflammation. In vitro studies mimicking macrophage foam cell formation using oxidized LDL (oxLDL) have shown that oxLDL is a potent inducer of macrophage glycolysis52, inflammation53, and mitochondrial oxidative damage54. OxLDL, and its associated oxidized phospholipids, have been shown to induce inflammatory signaling via TLR4 homodimers or TLR4/TLR6 heterodimers, leading to cytokine and chemokine expression, as well as changes in actin cytoskeleton55,56. In addition, the unregulated uptake of oxLDL via CD36 results in the nucleation of intracellular cholesterol crystals that destabilize lysosomes, impairing cholesterol and fatty acid metabolism, and activating the NLRP3 inflammasome and production of IL-1β57,58. Numerous cellular metabolic disturbances, all present in plaque macrophages, have been linked to NLRP3 inflammasome activation, including the release of lysosomal cathepsins, mitochondrial ROS, and extracellular ATP59. Furthermore, free cholesterol enrichment of macrophage foam cell membranes can enhance inflammatory signaling from lipid rafts, particularly via activation of TLR4 signaling and nuclear factor-κB (NF-κB), independent of exogenous ligand stimulation60–62. In addition, oxLDL can activate HIF-1α, which fuels increased glucose uptake, glycolysis and macrophage inflammatory responses52,63. Thus, macrophage foam cells generated in vitro exhibit multiple aspects of M[LPS+IFNg] macrophages, including increased glycolysis, TLR4 activation, and increased IL-1β production.
Attempts to characterize the atherosclerotic macrophage inflammatory phenotype in vivo have revealed the presence of multiple subsets of macrophages in plaques, which are dynamically regulated during the progression and regression of disease. Macrophages with general markers of the M[LPS+IFNg] phenotype are present in early atherosclerotic lesions, and the proportion of macrophages with this phenotype increases as plaques progress to more complex, inflammatory lesions64. These M[LPS+IFNg]-like macrophages are enriched in lipid, consistent with an increase in glycolysis, and populate different regions of the plaque than macrophages with markers of the M[IL-4] phenotype65. During atherosclerosis regression, the balance of M[LPS+IFNg]-like and M[IL-4]-like macrophages switches, with M[IL-4]-like macrophages becoming more prominent66–68. Plaque macrophages resembling the M[IL-4] phenotype show little lipid accumulation, consistent with a high level of FAO, and these cells are thought to be atheroprotective due to their anti-inflammatory and profibrotic properties. Indeed, treatment of Ldlr−/− mice with the M[IL-4]-polarizing cytokine IL-13 inhibits atherosclerosis progression69. In addition, a third macrophage phenotype, termed M(ox), has been identified in atherosclerotic plaques and is induced in vitro in response to oxidized phospholipids70. M(ox) macrophages are characterized by high expression of NRF2-dependent genes, including HO1, Srdxn1 and Txdn1, and a glutathione- and redox-regulating phenotype. These macrophages have decreased phagocytic and migratory capacity compared to macrophages stimulated with M[IL-4] or M[LPS+IFNg], and this may contribute to their persistence in plaques. Attempts to quantify these macrophage populations in advanced plaques of Ldlr–/– mice indicate a distribution of ~40% classically activated (CD86high), ~20% alternatively activated (CD206+), and ~30% M(ox) macrophages.
To date, metabolic phenotyping of plaque macrophages beyond cell surface markers has been limited. This is due not only to the challenges of isolating macrophages from plaques (typically done by aortic digestion, which can alter the cellular metabolic state), but also by previous technological limitations in understanding cellular phenotypes at the single cell level. Within the plaque, it is likely that discrete phenotypic populations and/or a phenotypic spectrum of atherosclerosis-induced macrophage activation states exist, driven by the various plaque microenvironmental signals encountered in a spatiotemporal fashion. Indeed, emerging and established single cell and imaging technologies hold promise to identify, characterize, and understand these spatiotemporal activation states within the plaque, enabling the ability to subsequently target either distinct phenotypic populations, or shift the phenotypic spectrum for therapeutic benefit.
Macrophage cholesterol storage and efflux
Under homeostatic conditions, lipoproteins taken up by macrophages are transported to late endo-lysosomal compartments where cholesterol esters are hydrolyzed. Niemann-Pick disease, type C (NPC) protein 1 and 2 act in concert to transport free cholesterol to the cytoplasm, where it is trafficked to the cell membrane for export or delivered to the ER for re-esterification and storage in lipid droplets. Macrophages in advanced plaques show evidence of massive free cholesterol accumulation, suggesting a breakdown in these processes that maintain cholesterol homeostasis. Excess free cholesterol accumulation is damaging to membranes, and causes dysregulation of metabolic processes in the ER and mitochondria that are essential for maintaining macrophage cholesterol homeostasis and reducing inflammation.
Cholesterol efflux via the lipid transporters ABCA1 and ABCG1 is key to maintaining cholesterol homeostasis in plaque macrophages and promoting reverse cholesterol transport to the liver for excretion71, and this process relies on proper mitochondrial function. A sustained supply of ATP is required to fuel the activity of ABC transporters to move their substrates (free cholesterol and phospholipids) across the membrane to an acceptor, and treatment of macrophages with oligomycin, which blocks the production of ATP from oxidative phosphorylation, markedly reduces cholesterol efflux to to apolipoprotein A1 (apoA1)72. Furthermore, macrophages from mice lacking PGC1α, a master regulator of mitochondrial biogenesis, have impaired cholesterol efflux72, and accordingly Ldlr–/– mice deficient in hematopoietic Pgc1a expression exhibit accelerated progression of atherosclerosis73. Mitochondria are also an important source of oxysterol ligands for the liver X receptor (LXR) transcription factors, which coordinate the expression of genes involved in the response to cholesterol excess, including ABCA1 and ABCG1. The mitochondrial sterol 27-hydroxylase CYP27A1 converts excess cholesterol to 27-hydroxycholesterol, and mitochondrial transport of cholesterol from the outer to inner membrane is rate-limiting in the CYP27A1 generation of oxysterols.
Studies of hypercholesterolemic mouse models suggest that mitochondrial metabolism is disturbed in atherosclerotic plaque macrophages. In the REVERSA mouse model of genetically reversible hypercholesterolemia, mitochondrial genes were found to be significantly downregulated in the aorta during progression of atherosclerosis, which coincided with rapid lesion expansion and elevated CD68+ macrophage content within the plaque74. Functional enrichment analysis showed an overrepresentation of mitochondrial and ROS homeostasis pathways, with genes involved in mitochondrial biogenesis and the antioxidant response (e.g., SOD2, UCP1, and UCP3) markedly diminished. Notably, upon genetic reversal of hypercholesterolemia, over 200 genes were upregulated in the aorta, 30% of which were nuclear encoded mitochondrial genes. Of those mitochondrial cholesterol responsive genes, 70% corresponded to ones downregulated during hypercholesterolemia and lesion expansion, suggesting that this effect can be reversed. While this study did not examine isolated macrophages, given their prominence in mouse atherosclerotic plaques, it can be speculated that macrophage mitochondrial function drives this response.
Strategies that increase mitochondrial biogenesis or function have been shown to enhance the export of cholesterol from macrophages. In atherosclerotic plaque macrophages, the microRNA (miR)-33 appears to be a nexus for repressing mitochondrial function, cholesterol efflux and fatty acid oxidation. miR-33a and miR-33b are upregulated in human and mouse atherosclerotic plaque68,72, and these non-coding RNAs post-transcriptionally inhibit genes in networks regulating mitochondrial biogenesis and function (Pgc1a, Slc25a3, Pdk4, Nrf1)72, intracellular cholesterol transport and efflux [NPC1 (humans only), OSBPL6, ABCA1, Abcg1 (mouse only)]75,76, autophagy and lipid droplet catabolism (ATG5, ATG12, LC3B, LAMP1)77,78, and fatty acid oxidation [HADHB, CPT1A, CROT, PRKAA1]79. Antagonism of miR-33 in mouse models of atherosclerosis has been shown to be atheroprotective through raising plasma levels of HDL-C, promoting macrophage cholesterol efflux and reverse cholesterol transport, enhancing mitochondrial function, and reducing macrophage inflammatory polarization68,72,80,81.
Oxidative Stress and Hypoxia
Oxidative stress and inflammation are interrelated processes which form a robust feed-forward cycle that fuels atherosclerotic plaque progression. During atherosclerosis, macrophage reactive oxygen species (ROS) are generated through the actions of mitochondrial oxidative metabolism, NADPH-oxidases, peroxidases, NO-synthases, cyclooxygenases, and lipoxygenases. The macrophage antioxidant response is key to decreasing cellular levels of ROS and protecting mitochondria and other organelles, proteins, and nucleic acids from oxidative damage, however the transcription of antioxidant genes and mitochondrial transport of the antioxidant glutathione (GSH) are suppressed in plaque macrophages, which can magnify inflammation in the artery wall. For example, NADPH-oxidase derived ROS from macrophages has been shown to promote oxidation of LDL in the artery wall, amplifying macrophage foam cell formation82. Furthermore, excessive mitochondrial ROS (mtROS) generated as a byproduct of electron transport chain reactions can damage mitochondrial DNA (mtDNA), proteins and lipids, and these have been shown to increase during atherosclerosis in mice83. The oxidation or release of mtDNA can also serve as a danger-associated molecular pattern that activates multiple innate immune signaling pathways in macrophages, including the CpG DNA receptor TLR9, the NLRP3 inflammasome84,85, and the cyclic GMP-AMP synthase (cGAS)-stimulator of interferon genes (STING) pathway86–88. Reducing mitochondrial oxidative stress in macrophages can prevent inflammation in plaques and reduce atherosclerotic burden in mice54.
In addition to cellular oxidative stress, the imbalanced demand and supply of oxygen in the plaque microenvironment leads to hypoxia in human and mouse atherosclerotic plaques, and is a key contributor to atherogenesis89. Local hypoxia within the plaque environment arises due to a combination of increased cellular metabolic demand coupled with impaired oxygen delivery due to increased diffusion distance within plaques90. In humans, hypoxic regions in the plaque microenvironment are rich in both macrophages and foam cells91, which show activated HIF-1α, and are associated with angiogenesis, and plaque hemorrhage90. In atherosclerotic mice, reduction of plaque hypoxia by hyperoxic carbogen gas administration (95% O2, 5% CO2) resulted in favorable changes in plaque characteristics, including an increase in M[IL-4]-like macrophages, decreased IL-6 production and reduced necrotic core size92.
ROS and hypoxia are potent drivers of macrophage metabolic reprogramming that results in enhanced reliance on glycolysis. Critical to this metabolic switch is activation of the HIF-1α transcription factor, which induces the expression of GLUT-1 and glycolytic enzymes (e.g., HK, PFK, and PFKB3) limits OXPHOS, and protects from increased acidification from the production of lactic acid93. HRE genes also include a variety of inflammatory genes, such TLR2, TLR4, IL-1β, and CXCR4, resulting in a coordinated inflammatory response. As such, HIF-1α pathway activation, particularly in macrophages, is critically involved in modulating both the metabolic and inflammatory effector responses. Increased glucose uptake associated with HIF pathway activation has been demonstrated in mouse and human atherosclerotic lesions. In human plaques, expression of HIF-1α colocalizes with high expression of GLUT1, GLUT3, HK1 and HK2 in macrophage-rich regions94, suggesting enhanced glycolysis in these cells. Consistent with this, expression of the HIF-1α-regulated cytokine IL-1β was also increased within macrophage-rich hypoxic plaque regions94, and hypoxia potently induced NLRP3 activation and IL-1β expression in human macrophages. Similarly, in the plaques of Ldlr–/– and Apoe–/– mice, there is an abundance of hypoxic, HIF-1α expressing macrophages90,95, and laser capture of HIF-1α-positive macrophages revealed significantly greater Glut1 expression compared to macrophages from normoxic regions. In vitro studies of macrophage foam cells support these in vivo observations. Hypoxia markedly potentiates macrophage glycolytic flux in the presence of proatherogenic mediators such as oxidized LDL and pro-inflammatory cytokines (IL-1β, IFNγ, TNFα)63, and this was proportional to enhanced inflammatory activity of these cells, and dependent on HIF-1α and PFKFB3. Interestingly, the glycolytic shift in response to hypoxia appears to be dependent on mitochondria for appropriate oxygen sensing, as macrophages that lack mitochondria fail to accumulate HIF-1α in response to hypoxia96. HIF-1α also increases macrophage sterol and triglyceride content by stimulating sterol synthesis and suppressing cholesterol efflux via ABCA195, which further exacerbates cholesterol accumulation in plaque macrophages. Macrophages deficient in HIF-1α exhibit reduced expression of inflammatory genes (e.g., monocyte chemoattractant protein-1, osteopontin), and apoptosis. Consistent with these findings, hematopoietic deficiency of HIF-1α90 or GLUT-150 reduces atherosclerotic burden in mice. Cumulatively, these studies implicate hypoxia as a driver of macrophage phenotype in atherosclerosis progression, highlighting that HIF-1α activation and increased glycolysis are central to this pathologic response.
Macrophage Death and Efferocytosis
Prolonged defects in cholesterol efflux and/or free cholesterol esterification by the ER can ultimately lead to macrophage death in the plaque. ER stress has been documented in human and mouse atherosclerotic plaque macrophages, particularly in the advanced stages of disease, and reducing ER stress can mitigate atherosclerosis97,98. ER stress leads to the induction of the unfolded protein response (UPR), which aims to re-establish homeostasis in the ER to maintain its functions in folding and secretion of proteins, calcium storage, and lipid synthesis for membrane biogenesis or energy storage. However, if prolonged, ER stress can trigger apoptosis, through the ER resident protein inositol requiring protein-1 (IRE1) or downstream effectors such as CHOP (C/EBP homologous protein)7. In the advanced plaque, clearance of apoptotic cells by surrounding macrophages can become compromised as these cells undergo metabolic dysfunction. Efficient clearance of apoptotic cells by macrophages requires intact lysosomal function and lipid metabolism to deal with the dramatic increase in lipids, protein, nucleotide and carbohydrate content that the cell undergoes after ingesting apoptotic bodies. Thus, the combination of defective macrophage cholesterol efflux, enhanced apoptotic cell death, and defective efferocytosis by plaque macrophages can result in secondary necrosis and the release of cellular components into the plaque milieu, which is thought to contribute to the formation of the lipid-rich, acellular necrotic core, which characterizes vulnerable plaques.
Efferocytosis is dependent on a coordinated metabolic cellular response. In vitro, FAO is enhanced during efferocytosis and altering mitochondrial membrane potential by depriving or providing excess glucose can increase and decrease efferocytosis, respectively99. Thus, mitochondrial membrane potential within macrophages is a critical determinant of phagocytic capacity and continued apoptotic cell uptake99. Mitochondrial morphology operates in a dynamic flux, changing rapidly through fission or fusion in response to external stimuli and cellular metabolic needs. In settings of nutrient deprivation, increased demand for mitochondrial respiration leads to mitochondrial fusion, which is associated with increased ATP production and resistance to autophagy100. In atherosclerosis, mitochondrial fission is required in response to apoptotic cell uptake for effective efferocytosis by macrophages in the atherosclerotic plaque101. Interestingly, M[IL-4] macrophages, which have high OXPHOS, have a greater efferocytosis capacity than M[LPS+IFNγ] macrophages, suggesting that this difference in metabolism could determine macrophage efferocytosis ability in atherosclerotic plaques. Consistent with this, factors associated with M[LPS+IFNγ] macrophages, such as oxidative stress and hypoxia, can inhibit efferocytosis in atherosclerotic plaques92,102.
Hyperglycemia
Hyperglycemia associated with type 1 or type 2 diabetes mellitus is an independent risk factor for atherosclerosis and cardiovascular events103, and is associated with increased inflammatory monocyte and macrophage activation and hematopoiesis104. Type 1 diabetic-induced hyperglycemia results in proliferation and expansion bone marrow myeloid cell progenitors and release of inflammatory monocytes into the circulation, coinciding with impairment of atherosclerosis resolution, which is mitigated with treatment of hyperglycemia104. Interestingly, overexpression of the glucose transporter GLUT1 in myeloid cells was found to increase glycolysis and flux through the PPP, coupled with a compensatory reduction in FAO; however, it alone did not induce cytokine production105. Furthermore, in Apoe–/– mice, myeloid-specific overexpression of GLUT-1 did not promote atherosclerosis. Thus, macrophage glycolytic flux alone is not sufficient to drive atherosclerosis, and the athero-promoting macrophage response to a hyperglycemic systemic milieu is driven by factors beyond increased rates of glycolysis. The metabolic changes in myeloid cells in the diabetic setting remains an area of active investigation.
Epigenetic and Metabolic Memory Shape Macrophage Responses in Atherosclerosis
While metabolic modulation is integral to the macrophage activation response, recent discoveries have also unveiled its role in ‘learned’ innate functional responses. Macrophages exposed to acute or chronic stimuli, both in vitro and in vivo, can remodel their chromatin to mount a ‘learned’ response to subsequent stimulation with the same or different stimuli, termed innate immune memory. These memory responses are characterized phenotypically by cellular inflammatory responses that are regulated, in part, by changes in metabolic machinery. While these effects have predominately been defined in the context of pathogen response, emerging evidence suggests that innate immune memory also regulates the metabolic and effector response to sterile inflammatory disease states, such as atherosclerosis.
Two categories of innate immune memory responses to pathogenic stimuli have been described: ‘trained’ or ‘tolerant’ effector responses. Innate immune training, which results in a potentiated inflammatory response of monocytes and macrophages, occurs in response to pathogenic stimuli such as Bacillus Calmette-Guéri (BCG) vaccination and β-glucan (Figure 3). Innate immune tolerance, which results in a dampened response to secondary stimuli, occurs following exposure to LPS. These divergent responses are facilitated, in part, by metabolic cellular adaptations. For example, β-glucan induced training is accompanied by a metabolic switch from OXPHOS to aerobic glycolysis via activation of the mTOR-HIF1α pathway. Epigenetic analysis of cells after β-glucan stimulation revealed an increase in H3K4me3 and H3K27Ac in the promoter region of EIF4EBP1, the main target of mTOR, and highly increased expression of genes involved in the mTOR signaling and glycolytic pathways106. The increase in H3K27ac in β-glucan trained monocytes has been linked to a reduction in the NAD+-dependent class III histone deactylase sirtuin-1, and early treatment with the sirtuin-1 activator resveratrol during β-glucan training partially reduced IL-6 production106. Integration of the transcriptome and metabolome of β-glucan-trained monocytes/macrophages also revealed upregulation of metabolic pathways, including glucose metabolism, glutaminolysis, and cholesterol synthesis27,106. By contrast, in macrophages undergoing innate immune tolerance by LPS there is a metabolic switch from glycolysis to oxidative phosphorylation and increases in NAD+/NADH, which activate the histone deactylases sirtuin-1 and sirtuin-6 to inhibit inflammatory gene transcription107. Collectively, these findings have established the paradigm that the innate immune memory of macrophages is linked to their metabolic programs and can influence their subsequent inflammatory response to microbial, and likely, endogenous inflammatory ligands.
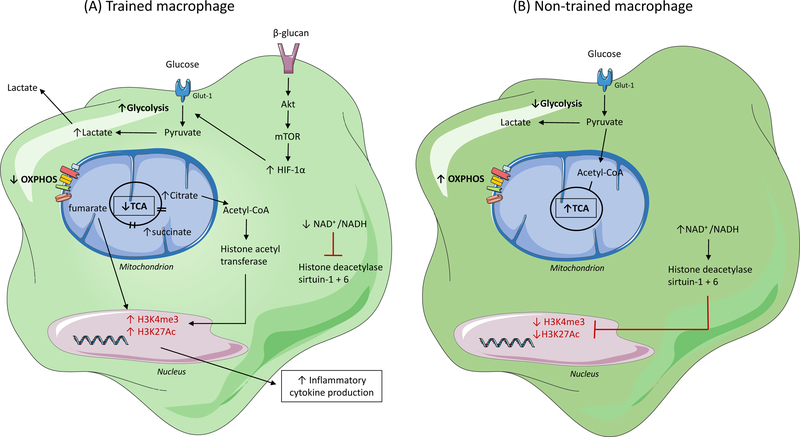
Stimuli that activate immune cells and induce metabolic rewiring are also capable of inducing epigenetic remodeling, through histone modifications, which results in innate immune memory. Histone modifications, including methylation and acetylation of specific residues within promotor regions of genes, which occur via the activity of histone-modifying enzymes, determine chromatin structure and enable transcription factor recruitment. The effect of methylation on transcription depends on the targeted residue and the degree of methylation; for example, trimethylation of histone H3 at lysine-4 (H3K4me3) is associated with transcriptional activation, whereas trimethylation of histone H3 at lysine-27 (H3K27me3) is linked with gene repression. Acetylation on lysine residues leads to a more open accessible chromatin state and is associated with gene expression. In trained macrophages (A) (i.e. those stimulated with BCG or β-glucan), a broken tricarboxylic acid (TCA) cycle results in the accumulation of citrate and succinate. These metabolites activate histone-modifying enzymes, such as histone acetyl transferase, which leads to an increase in H3K4me3 and acetylation of histone H3 at lysine-27 (H3K27Ac) at promoter sites of inflammatory cytokine genes and subsequently results in an increased inflammatory response. Additionally, a decreased nicotinamide adenine dinucleotide (NAD+) to NAD plus hydrogen (NADH) ratio in trained cells inhibits the activity of NAD+-dependent histone deacetylase sirtuin-1 and −6 supporting the increased inflammatory response. This response is absent in non-trained macrophages (B), which have lower basal levels of H3K4me3 and H3K27Ac due to an intact TCA cycle (preventing the accumulation of citrate and succinate), and an increased NAD+/NADH ratio which activates histone deacetylase sirtuin-1 and sirtuin-6. OXPHOS, oxidative phosphorylation; Glut-1, glucose transporter-1; Acetyl-CoA, acetyl-coenzyme A; HIF-1α, hypoxia-inducible factor-1-alpha; Akt; protein kinase B.
Innate immune memory occurs not only in monocytes and macrophages, but also within myeloid progenitor populations in the bone marrow. β-glucan treated mice showed increased HSPC populations, including long term hematopoietic stem cells (LT-HSCs), up to 28 days post-treatment, which was transferrable by bone marrow transplantation of LT-HSCs into naïve mice108. RNA-Seq of LT-HSCs at 7 days post-β-glucan injection identified glycolysis, cholesterol biosynthesis, and the mevalonate pathway as overrepresented in genes that were significantly upregulated, while genes involved in cholesterol efflux were decreased. Within the bone marrow, IL-1β was increased, as well as increased numbers of CD131+ (the GM-CSF receptor) HSPCs. Treatment with an IL-1 receptor antagonist or blockade of GM-CSF restricted the β-glucan-induced expansion of HSPCs, as did inhibition of glycolysis with 2DG or the cholesterol biosynthesis pathway by statin therapy.
In monocytes, activation of the cholesterol biosynthesis pathway, but not cholesterol synthesis itself, was found to be essential for the induction of trained immunity109. Similar to observations in HSPCs, statins prevented β-glucan and BCG-induced training, blunting both the upregulation of cytokine production upon secondary LPS stimulation, as well as increased glycolysis. The addition of exogenous mevalonate, the metabolite directly downstream of HMG-CoA reductase (the target of the statin class of drugs), was sufficient to rescue the β-glucan innate immune training phenotype in the presence of a statin, and mevalonate alone could induce a trained immunity phenotype through epigenetic reprogramming, enhanced glycolysis and mTOR signaling. The ability of mevalonate to induce innate immune training indicates that this response is not restricted to microbial stimuli, and makes it likely that factors associated with sterile inflammatory insults, such as those that occur in atherosclerosis and other chronic inflammatory conditions, may induce similar effector and metabolic responses that heighten inflammation.
Recent studies have implicated two sterile inflammatory microenvironmental factors of atherosclerosis, namely oxLDL and hypercholesterolemia, as sterile drivers of innate immune training. Human monocytes exposed to oxLDL53 and then re-stimulated with TLR2 (Pam3Cys) or TLR4 (LPS) agonists exhibited an enhanced inflammatory response similar to β-glucan training. Notably, monocytes trained with oxLDL showed increased expression of inflammatory cytokines (IL-6, TNFα, IL-8, MCP-1), which was reversed by 5-methylthioadenosine (a methylation inhibitor), thus implicating epigenetic reprogramming in this response. In an independent study, human monocytes trained with oxLDL for 5 days and then stimulated with LPS also exhibited enhanced IL-6 and TNF-α responses110, which was abrogated by treatment with recombinant IL-1R antagonist. Notably, statin treatment of oxLDL-trained monocytes also blunted the immune training phenotype by mitigating the enhanced glycolytic and proinflammatory cytokine response to LPS re-stimulation109. In vivo, a hypercholesterolemic environment was also found to drive innate immune training110. Monocytes isolated from Ldlr–/– mice fed a western diet (WD) for 4 weeks showed an enhanced cytokine and chemokine inflammatory response when stimulated ex vivo with various TLR agonists. Furthermore, in vivo stimulation of mice fed WD for 4 weeks with LPS induced higher levels of serum inflammatory cytokines compared to chow diet fed mice. Consistent with previous studies, WD increased myelopoiesis, including levels of the myeloid progrenitor GMP population, which showed priming of inflammatory genes and a downregulation of cholesterol biosynthesis pathways upon RNA sequencing. Notably, switching WD-fed mice back to chow diet for 4 weeks normalized the systemic inflammatory milieu, but did not normalize the enhanced monocyte response to ex vivo TLR stimulation. Using RNA and ATAC sequencing (which identifies changes in open and accessible chromatin) of GMPs from mice fed either chow or WD for 4 weeks, or WD for 4 weeks then chow diet for 4 weeks, and then challenged with LPS, the authors concluded that WD effectively reprogrammed GMPs at the transcriptional and epigenetic level. Treatment of mice with an IL-1R antagonist, or deficiency of the NLRP3-inflammasome that regulates IL-1β production, abrogated the enhanced cytokine response of WD-trained monocytes indicating that IL-1β plays a key role in myeloid cell reprogramming during hypercholesterolemia.
Extending these findings to clinical populations, circulating monocytes from patients with atherosclerosis also appear to have an enhanced inflammatory signature that is associated with epigenetic remodeling, which may also be connected to metabolic alterations. For example, an enhanced inflammatory cytokine response to TLR agonists also occurs in patients with either high lipoprotein(a) levels111 or established atherosclerosis112. Monocytes isolated from patients with symptomatic atherosclerosis elicited a stronger cytokine response to ex vivo challenge with LPS and P3C, compared to controls. Subsequent analysis of mRNA from whole blood circulating cells found greater expression of glycolytic (HK2, PFKFB3, PKM1, PDHA1), TCA cycle (ASL, MDH1) and PPP (TALDO1) genes compared to controls112. Collectively, these studies in mice and humans have unveiled the potent effects of innate immune training on circulating monocytes and hematopoietic progenitors that may contribute to atherogenesis. Given the recent exciting findings of the CANTOS trial, in which therapeutic targeting of IL-1β using the monoclonal antibody canakinumab reduced the rate of recurrent cardiovascular events in patients with previous myocardial infarction16, it is notable that inhibiting IL-1β signaling reduced macrophage innate immune training in response to a hypercholesterolemic environment110. These findings suggest that part of the beneficial effects of blocking IL-1β signaling in cardiovascular disease may derive from its ability to reverse myeloid cell epigenetic reprogramming during inflammation, and subsequent heightened immune responses.
Summary and Future Directions
Much work remains to unravel the intertwined and dynamic metabolic and epigenetic landscapes that determine immunologic function in myeloid cells, as they are exposed to different microbial stimuli and unique tissue microenvironments in health and disease. In atherosclerosis, it is clear that macrophages exhibit metabolic rewiring in response to dyslipidemic and environmental factors (hypoxia, dying cells, cytokines) that lead to metabolic heterogeneity among plaque macrophages. These metabolic changes are likely dynamic as the microenvironment evolves during both plaque progression and treatment of disease. Although not discussed in this review, metabolic reprogramming is also ongoing in the other cell types in the plaque, including T cells, dendritic cells, endothelial cells, and smooth muscle cells, and these changes will, in turn, impact macrophage metabolic programs through nutrient competition, extracellular accumulation of metabolic by-products, and cell-cell signaling and interactions.
In the field of basic macrophage biology, there is a need for better delineation of metabolic adaptations and the role of specific metabolites in regulating distinct macrophage effector responses, such as phagocytosis, cytokine production, cholesterol efflux, autophagy and extracellular matrix synthesis and degradation. Although these processes are often studied in isolation in cultured cells, there is also a need to understand how macrophages coordinate their metabolic needs when they must exert multiple effector responses simultaneously. Furthermore, moving beyond single environmental stimulatory agents, there is a need to understand how macrophages behave in vivo, where the microenvironment is more complex and macrophages experience multiple stimuli concurrently. Further study, particularly at the single cell level, will be needed to dissect how macrophages prioritize the different signals that they are exposed to and adopt metabolic signatures that fulfill these different needs.
From an atherosclerosis perspective, it will be important to better understand how metabolic signatures of monocytes and macrophages change during early atherogenesis, and to determine the point at which these programs become maladaptive and disease promoting. This will require studying macrophage metabolism across multiple stages of atherosclerosis, including early foam cell lesions, advanced plaques that are more complex, and importantly, plaques vulnerable to rupture, which are the precursors to myocardial infarction. To date, metabolic studies of macrophages taken from the plaque have been limited due the challenges of their isolation, yet these cells offer metabolic clues to understand the switch from functional to dysfunctional metabolic programs.
There is intense interest in exploiting the plasticity of macrophage metabolism to rebalance inflammatory responses and restore protective immune functions in atherosclerosis. A better understanding of the specific metabolic rewiring of activated macrophages during both progression and regression of atherosclerosis will be of fundamental importance in identifying novel therapeutic approaches. There is already evidence from mouse models of atherosclerosis regression that lowering of apoB-lipoprotein production or raising of circulating HDL levels, shifts the macrophage phenotype in the plaque toward M[IL-4]-like tissue reparative macrophages66–68, and that this requires the ongoing recruitment of Ly6chi monocytes into the plaque113. The identification of drugs that target metabolic intermediates or pathways that could reorient macrophage effector functions to restore homeostasis to the artery wall are needed. In addition, as more evidence emerges that epigenetic memory and metabolic memory are interconnected, drugs that alter epigenetic marks on chromatin are being explored as potential tools to manipulate macrophage inflammatory responses. The increasing availability of small molecules capable of manipulating metabolic routes and epigenetic marks is opening new avenues for discovery and potential therapeutic application in atherosclerosis and beyond.
REFERENCES
Full text links
Read article at publisher's site: https://doi.org/10.1038/s41590-018-0113-3
Read article for free, from open access legal sources, via Unpaywall:
https://www.ncbi.nlm.nih.gov/pmc/articles/PMC6314674
Citations & impact
Impact metrics
Citations of article over time
Alternative metrics
Smart citations by scite.ai
Explore citation contexts and check if this article has been
supported or disputed.
https://scite.ai/reports/10.1038/s41590-018-0113-3
Article citations
Exploring the mechanism of Zhengxintai Formula for the treatment of coronary heart disease based on network pharmacology.
Medicine (Baltimore), 103(41):e40065, 01 Oct 2024
Cited by: 0 articles | PMID: 39465849 | PMCID: PMC11479439
Inflammation in atherosclerosis: pathophysiology and mechanisms.
Cell Death Dis, 15(11):817, 11 Nov 2024
Cited by: 0 articles | PMID: 39528464 | PMCID: PMC11555284
Review Free full text in Europe PMC
Crosstalk between macrophages and immunometabolism and their potential roles in tissue repair and regeneration.
Heliyon, 10(18):e38018, 18 Sep 2024
Cited by: 0 articles | PMID: 39381218 | PMCID: PMC11458987
Review Free full text in Europe PMC
The multifaceted potential of <i>TPT1</i> as biomarker and therapeutic target.
Heliyon, 10(19):e38819, 01 Oct 2024
Cited by: 0 articles | PMID: 39397949 | PMCID: PMC11471257
Review Free full text in Europe PMC
Macrophage energy metabolism in cardiometabolic disease.
Mol Cell Biochem, 29 Aug 2024
Cited by: 0 articles | PMID: 39198360
Go to all (209) article citations
Similar Articles
To arrive at the top five similar articles we use a word-weighted algorithm to compare words from the Title and Abstract of each citation.
Targeting Macrophage Phenotypes and Metabolism as Novel Therapeutic Approaches in Atherosclerosis and Related Cardiovascular Diseases.
Curr Atheroscler Rep, 26(10):573-588, 12 Aug 2024
Cited by: 0 articles | PMID: 39133247 | PMCID: PMC11392985
Review Free full text in Europe PMC
Intracellular and Intercellular Aspects of Macrophage Immunometabolism in Atherosclerosis.
Circ Res, 126(9):1209-1227, 23 Apr 2020
Cited by: 77 articles | PMID: 32324504 | PMCID: PMC7392397
Review Free full text in Europe PMC
Monocyte and macrophage immunometabolism in atherosclerosis.
Semin Immunopathol, 40(2):203-214, 02 Oct 2017
Cited by: 107 articles | PMID: 28971272 | PMCID: PMC5809534
Review Free full text in Europe PMC
Mitochondria orchestrate macrophage effector functions in atherosclerosis.
Mol Aspects Med, 77:100922, 06 Nov 2020
Cited by: 17 articles | PMID: 33162108
Review
Funding
Funders who supported this work.
NHLBI NIH HHS (2)
Grant ID: P01 HL131481
Grant ID: R35 HL135799