Abstract
Free full text

Models and Tools for Studying Enteroendocrine Cells
Abstract
Gut hormones produced by gastrointestinal enteroendocrine cells modulate key physiological processes including glucose homeostasis and food intake, making them potential therapeutic candidates to treat obesity and diabetes. Understanding the function of enteroendocrine cells and the molecular mechanisms driving hormone production is a key step toward mobilizing endogenous hormone reserves in the gut as a therapeutic strategy. In this review, we will discuss the variety of ex vivo and in vitro model systems driving this research and their contributions to our current understanding of nutrient-sensing mechanisms in enteroendocrine cells.
The gastrointestinal (GI) tract epithelium is produced by stem cells that differentiate into absorptive and secretory cell types, including the rare hormone- producing enteroendocrine cells (EECs). Taken as a whole, EECs make up the largest endocrine system in the body, with >20 different gut hormones having been described. Classically, EECs have been defined by which hormones they express and secrete (Fig. 1), although it is now evident that individual EECs exhibit an unexpected degree of heterogeneity in hormone expression (1). Gut hormones play a number of roles in normal and disease physiology and are a major focus of academic and industrial research. The GI tract is exposed to ingested nutrients, circulating hormones/nutrients, and other GI components such as bile acids and bacterial metabolites. Many of these act as physiological stimuli that promote or inhibit hormone secretion from EECs (Fig. 1). Once secreted, gut hormones can act locally in a paracrine manner and target other cells in the mucosa and local neuronal networks or enter the bloodstream to reach distant organs. In this review, we will highlight the different experimental models and tools used for EEC research.
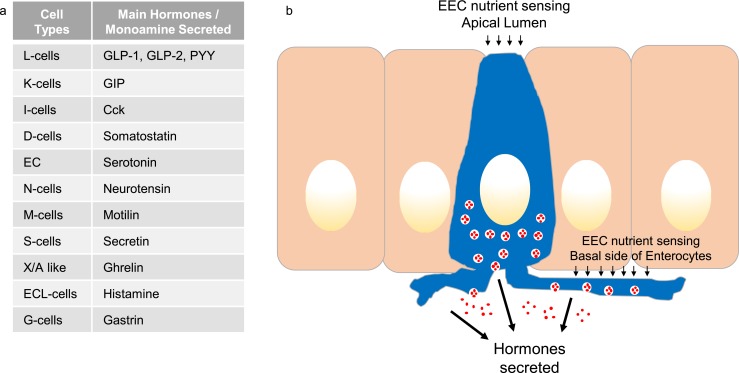
EECs and their hormones. (a) Table listing the different EEC types and the main hormones/monoamines they produce. (b) Schematic showing an open-type EEC (blue) facing into the gut lumen, between neighboring enterocytes in the intestinal epithelium, highlighting sites of stimulus detection and hormone (red) secretion. CCK, cholecystokinin; GIP, glucose-dependent insulinotropic peptide; GLP, glucagon-like peptide.
Model Tissues for Enteroendocrine Research
There are many models currently being used to investigate EECs. In this review, we will concentrate on ex vivo and in vitro models. The majority of research in the field has been performed using animal tissues (mostly rodent and in particular murine), as these are readily available, relatively inexpensive, and make use of the multiple transgenic strains available. Ideally, for research into the role of EECs in health, human tissue would be used, but this is restricted by tissue availability, practicalities, and additional technical difficulties. Some studies have used pigs, as it has been suggested they are a more relevant model for humans; unlike rodents, for example, they express the hormone motilin. However, there are key digestive differences between the two species, including increased GI fermentation in pigs, which is likely to influence EEC hormone secretion. Pigs also have the disadvantage over rodents that due to their size, studies require larger facilities, more labor time, and increased animal housing costs.
In this review, we will discuss ex vivo model systems involving isolated intestinal perfusion and Ussing chambers and in vitro models such as cell lines, short-term primary cultures, and intestinal organoids (Fig. 2). The characteristics of each model with regard to throughput capability, tissue suitability, longevity, etc., have been summarized in Fig. 2a.
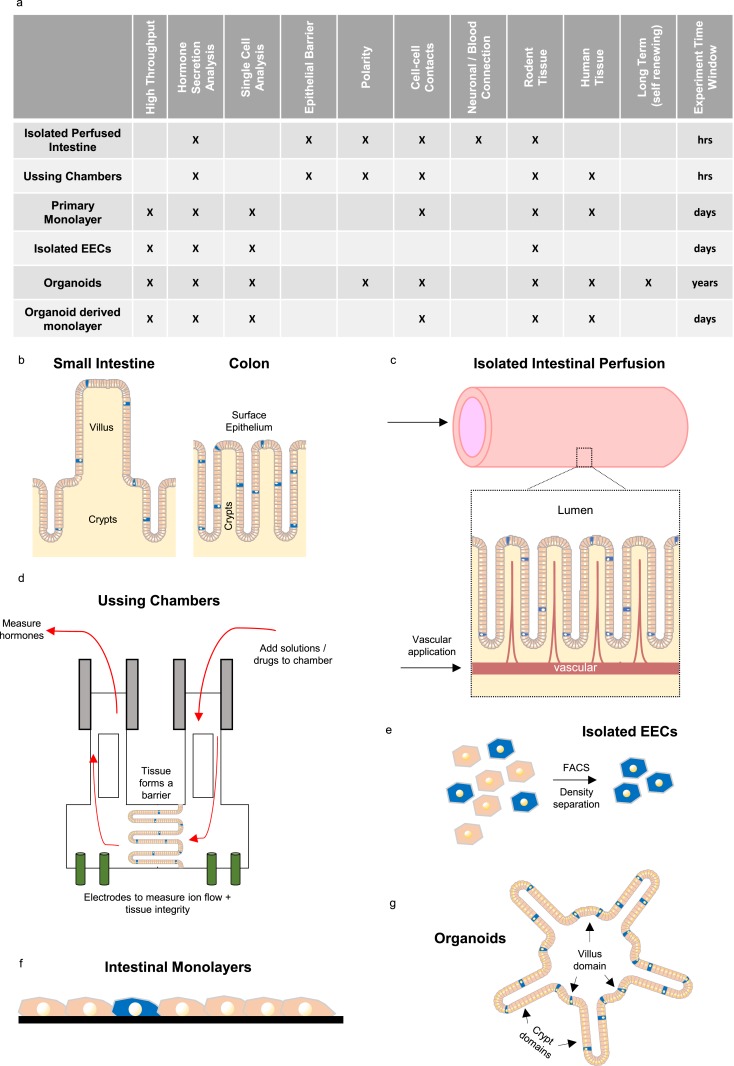
Ex vivo and in vitro models for studying EECs. (a) Table showing the different features and applications of ex vivo (isolated perfused intestine and Ussing chambers) and in vitro (primary cultures, isolated EECs, and organoids) models described in the text. (b) Schematic of small intestine and colonic epithelium with the rare EECs (blue) found in both crypts and mature epithelium (villi and surface epithelium). (c–g) Diagrams showing the different model systems. (c) In isolated perfused intestine, solutions can be perfused via the intestinal lumen and vascular supply. (d) Ussing chamber setup, in which tissue forms a barrier between two compartments (integrity measure by electrodes). Solutions can be added to, and sampled from, either compartment. (e) Single-cell digest of intestinal epithelium, enabling EECs to be isolated by fluorescence-activated cell sorting (FACS) or Percoll density gradient. (f) Primary intestinal monolayers generated by epithelial digestion and seeding onto a matrix scaffold (black) to form a nonpolarized monolayer in which EECs are intermixed with other epithelial cell types. (g) Three-dimensional intestinal organoid structures resembling in vivo architecture (crypt and villi domains) with polarized epithelium containing all cell types including EECs.
Ex Vivo Studies
Isolated intestinal perfusion and Ussing chamber systems are classic ex vivo models that have been widely used to investigate EEC function in different regions of the GI tract (2–10). Intestinal perfusion can be performed on animals as small as mice and generally involves isolating a target gut segment, whereas it is physically retained within the abdominal cavity, enabling vessel cannulation while the heart is still beating. The gut segment under investigation is defined by the area supplied by the cannulated artery, and the lumen can be additionally cannulated and perfused (Fig. 2c). In further modifications, the method has been adapted to enable gut hormone sampling from the intestinal lymph (11). Perfused intestinal models can be used to monitor hormone secretion from different regions of the small intestine or the colon and are a useful tool to study EECs in the context of their surrounding environment, as their local neuronal and vascular circuits largely remain intact, thus supporting near-normal regulation of the EECs. The intestinal epithelial barrier is also maintained, allowing luminal and systemic application of nutrients or drugs to identify their directionality of action. A blood substitute without red blood cells is commonly used for the vascular perfusion, allowing larger sample volumes and more frequent sampling than is possible in in vivo studies on mice, and thus better resolution of hormone secretory dynamics. Hormone secretion has also been measured from isolated intestinal loops mounted in liquid paraffin, which can be readily perfused via the gut lumen but not through the vasculature. This method relies on the detection of hormones after they have diffused through the muscular layers and serosa into the surrounding space, where they collect in small aqueous pools adjacent to the paraffin (12). Although this is a simpler experimental setup than the vascularly perfused intestine, hormone diffusion is too slow to allow resolution of the time course of secretion, and the tissue is more prone to hypoxia due to the lack of continuous vascular perfusion with oxygenated medium. For obvious ethical reasons, isolated human intestinal perfusion studies are not possible, and most research is performed in rodents or sometimes in pigs, as they are considered a more human-relevant model. Isolated intestinal perfusion studies involve highly specialized surgical techniques and are of low throughput compared with the in vitro models, which are quicker, easier, and can be scaled up to enable the parallel examination of multiple test conditions.
Ussing chambers have also been extensively used to study hormone secretion from intact intestinal tissue pieces (2, 6–10). This method involves mounting tissue segments in specialized chambers that were classically used to investigate mucosal physiology and pharmacology (Fig. 2d). As with the perfused intestinal models, the epithelial barrier is maintained, enabling questions to be asked about whether drugs and nutrients target EECs from the luminal or basolateral direction (2, 10). In this system, the integrity of the tissue (epithelial barrier) can be assessed using electrodes that enable live assessment of the transepithelial resistance and potential difference of the mounted specimen. Hormone secretion in Ussing chamber studies can be monitored either by directly assaying hormone concentrations in the basolateral chamber or by measuring the electrical properties of the epithelium, which are altered when secreted hormones such as peptide YY (PYY) and glucagon-like peptide-1 (GLP-1) target their receptors on enterocytes or enteric neurons. Electrophysiological changes in response to cAMP elevation through forskolin/3-isobutyl-1-methylxanthine can also be used to assess the responsiveness/viability of the investigated tissue at the end of the experiment. When hormone concentrations are measured in Ussing chambers by immunoassay, optimization of the chamber volumes and tissue size is required to ensure that hormone concentrations are not below the detection limit of the assay. It is also sometimes necessary to strip away the muscle layers to improve diffusion of hormones and stimuli between the EECs and the basolateral fluid compartment.
A considerable drawback of both these ex vivo systems is the limited experimental window during which the tissue remains healthy. Unlike in vitro models, which can be kept for a few days in primary culture or maintained indefinitely as cell lines, the ex vivo model systems described previously remain viable for only a few hours. Upper small intestinal tissue mounted in an Ussing chamber, for example, has been shown to become compromised after just 2 hours, although more distal regions were slightly more resilient (13). This has contributed to a paucity of Ussing chamber studies examining EECs in the upper GI tract. Ussing chambers have been used to study human as well as mouse EECs, as the method only requires small tissues pieces that can be obtained under ethical approval from routine surgical operations that resect normal tissue as part of a clinical procedure. Nevertheless, studies on human intestine are limited by tissue availability and by the integrity of the epithelium, as human samples generally incur substantial delays between tissue collection and experimentation (14).
In Vitro Studies
Historically, a basic cellular tool for many researchers has been cell lines, immortalized cells that can be routinely passaged and maintained and scaled up for high-throughput studies or experiments requiring large cell numbers. There are multiple cell lines used as models for EEC research, including GLUTag, NCI-H716, STC-1, HuTu-80 and BON cells. Several cell lines have been used as models for GLP-1–producing l-cells, including GLUTag, a murine endocrine tumor-adherent cell line, STC-1, a rat adherent cell line and NCI-H716, a human-derived suspension cell line (15–18). Multiple disadvantages are associated with the use of cell lines in research: many lines are derived from tumors and therefore have genetic and morphological differences from their in vivo counterparts, they are a simplified system and grown without other cell types present, and they lack many physiological traits of their native counterparts with regards to cell morphology and polarity. At the transcriptomic level, GLUTag and STC-1 cells exhibited many similarities to native l-cells, but also a number of differences, including altered expression of key G-protein–coupled receptors (GPCRs) involved in l-cell sensing (19, 20). A recent analysis of different EEC lines also found substantial differences in hormone processing compared with native cells (18). Cell lines do, however, play an important role in EEC research, particularly for studies that require high cell numbers or high throughput.
In the last decade, techniques and protocols have been developed for the use of primary EECs in research. A number of groups have measured hormone secretion from intestinal tissue biopsies, but responses to stimuli are relatively weak in this setting (14, 21). In 2008, Reimann et al. (22) published the first protocol for the use of short-lived (days) two-dimensional (2D) primary cultures of the murine intestinal epithelium for studying EECs, which has recently been published as a video protocol (23) (Fig. 2f). This method enabled, for the first time, assessment of primary l-cells by electrophysiology and calcium imaging techniques, combined with measures of hormone secretion. Since publication, this technique has been successfully applied to intestinal tissues from other species including human and has supported the identification of many nutrient- and drug-activated pathways involved in the release of gut hormones from varying regions along the GI tract (2, 6, 7, 20, 24–26). Typical preparations from mouse or human tissue samples generate approximately one 24-well plate per 5- to 10-cm length of mouse intestine, so this method allows several drug treatments to be tested in parallel, giving a relatively high experimental throughput compared with ex vivo studies. Although the protocol can potentially be scaled up to 96-well plates or beyond, the method is ultimately limited by the sensitivity of the immunoassays used for hormone detection. The major drawbacks of this system are the short-lived nature of the culture, the isolation of EECs from some of the surrounding cell types, loss of apico-basal polarity, and lack of the epithelial barrier. In cultures derived from the small intestine, EECs only survive for a few days, whereas EECs from the colon were still functional after 10 days (22). No new EECs appear to form under these culture conditions (22, 23), so every preparation requires a fresh tissue sample, incurring associated animal costs and local availability of suitable mouse strains. Because these intestinal primary cultures do not generate an epithelial barrier or exhibit apico-basal polarity, they are not suitable for analysis of whether stimuli act via the apical or basolateral surface.
Once EECs have been completely separated from their neighbors by enzymatic or EDTA dispersal, they seem not to survive for long in culture, although calcium imaging was successfully performed in acutely isolated cholecystokinin (CCK)–producing cells by flow cytometry based on their cell-specific expression of GFP (27). By contrast, Raghupathi et al. (28) separated enterochromaffin cells, an EEC type that primarily secretes serotonin (Fig. 2e), using a Percoll density gradient and generated a 95% pure population of enterochromaffin cells that could be kept in culture for up to 4 days with a 70% survival rate (28–30). The enterochromaffin cells isolated by this process have been assessed using amperometry, quantitative RT-PCR (RT-qPCR), and calcium imaging. The drawbacks of this technique are similar to those described for primary intestinal cultures with the added uncertainty of the potential functional consequences of separating EECs from their neighboring enterocytes.
The use of GI organoids rather than primary cultures eliminates the need for a constant supply of freshly harvested tissue (Fig. 2g). Intestinal organoids are three-dimensional (3D) structures grown from stem cells and consist of organ-specific cell types that self-organize and show spatially restricted lineage commitment (31). These organoids are initiated from either pluripotent or adult stem cells and grown in specialized media that mimic the stem cell niche. Protocols to generate intestinal organoids from embryonic or induced pluripotent stem cells are under development, but still require refinement to optimize the differentiation and maturation of EECs (32, 33). It is relatively straightforward, however, to produce organoids from adult intestinal stem cells, which are coerced to form organoids by creating conditions that mimic the stem cell niche environment deployed during tissue self-renewal or damage repair. Indeed, the intestine is a self-renewing tissue containing abundant adult crypt stem cells and was one of the first tissues to be used to generate long-term organoids from mouse and human (34, 35). The 3D organoids can be maintained in culture by regular splitting and replating and can be cryopreserved in much the same way as a standard cell line. Depending on the intestinal region and species of origin, however, it is often necessary to change the culture conditions to promote the formation of fully differentiated EECs, and, once they have been induced to form a terminally differentiated state containing functional EECs, organoid cultures generally have a limited survival of up to a week, thus resembling the behavior of primary cultures derived from freshly harvested tissue.
Intestinal organoids have recently been used to investigate EEC function and represent a promising model for EEC research (36, 37). When generated from transgenic mouse models expressing fluorescent sensors and reporters driven by different gut hormone promoters, for example, they provide a replenishable source of identifiable EECs for single-cell analysis. Organoid cultures have been shown to contain different types of EECs and to retain their regional identity with regard to the profile of gut hormones produced (38). Other studies have shown that EECs generated within intestinal organoids are responsive to a range of physiological stimuli and are secretion competent (36, 37, 39, 40). Cells within 3D organoids have apico-basal polarity with their apical membranes located toward the organoid core. Apical surfaces are therefore largely inaccessible when stimuli are applied via the media, which is a potential limitation of the model, as most nutritional stimuli first reach their target EECs in vivo via the gut lumen. However, experiments using dextran labeling have suggested that organoids are leaky and that solutions can readily reach the apical membrane (40). We recently developed a 2D plating method for intestinal organoids to facilitate patch clamp electrode access, cellular imaging within a single focal plane, and measurements of GLP-1 secretion (36). Data from these studies showed that l-cells derived from murine organoids exhibited similar responses to l-cells in ileal primary cultures, thus validating the system as a good model for EEC research. This method is particularly exciting because organoids, like cell lines, can be genetically modified to produce lines with knockout, knockin, and overexpression of genes of interest. Similar genetic manipulations of human derived organoids would give this technique unique advantages compared with other ex vivo and in vitro models.
Interestingly, recent progress has been made in converting 3D organoid cultures to an open-faced polarized epithelium that can be used for transport studies (41, 42). One such technique has been shown to recapitulate the normal intestinal hierarchy and morphology, with the formation crypt/villus structures resembling in vivo tissue architecture (41). This method combined with genetically modified organoids will potentially open up new avenues for EEC research that are not currently possible with existing techniques.
Experimental Tools for EEC Research
Hormone secretion studies
The experimental models described previously have been developed to study the mechanisms regulating hormone secretion in response to application of drugs or physiological stimuli. There is widespread interest in this field, because gut hormones have a variety of physiological roles, are currently exploited therapeutically for the treatment of type 2 diabetes and obesity, and are under evaluation as future drug targets for a variety of metabolic and GI conditions. Hormones secreted by EECs have been measured by ELISA, radioimmunoassays, mass spectrometry, and amperometry and by their effects on epithelial short circuit currents.
Immunoassays use antibodies directed against target peptides, linked to quantifiable outputs such as enzymatic reactions, radioisotopes, or electroluminescent signals that are related to the quantity of antibody bound to the target peptide. The best immunoassays have a very high sensitivity, which is essential for the analysis of gut hormone concentrations that typically range from 1 to 100 pg/mL in plasma. Nevertheless, an immunoassay is only as good as its component antibodies, which must have both high affinity and high specificity. These are typically tested by cross-reactivity experiments with other peptides as well as standard curve analysis using serial dilutions of target peptides. ELISAs have been successfully used to measure GLP-1, glucose-dependent insulinotropic peptide (GIP), somatostatin, neurotensin, serotonin, and PYY secretion from a variety of ex vivo and in vitro models (4, 6, 22, 26, 29, 43). Drugs that directly target known secretory signaling pathways, including cAMP (3-isobutyl-1-methylxanthine and forskolin) and protein kinase C (phorbol myristic acid), are typically used as positive controls in hormone secretion experiments.
As discussed previously, individual EECs can produce multiple hormones, and an ideal detection method would therefore enable the parallel measurement of multiple hormones from the same sample. This can be achieved by multiple or multiplexed ELISAs, but the performance of multiple assays on the same sample is generally limited by cost and sample volumes, and many multiplexed assays still perform less well than when individual hormones are assayed separately. The use of liquid chromatography–tandem mass spectrometry (LC-MS/MS) for the detection of peptide hormones is an emerging field that enables the parallel detection of multiple hormones from a single sample (44, 45). This is particularly useful for hormones such as insulin-like 5 (INSL5), for which there are currently no reliable commercial immunoassays available (46). Using LC-MS/MS, we could detect INSL5 secretion from primary human and mouse colonic cultures (44) and have simultaneously measured GLP-1, PYY, and INSL5 release from human and mouse colonic cultures in response to a variety of drugs and physiological stimuli (47). LC-MS/MS analysis of hormone levels does come with some caveats compared with traditional ELISAs, including generally lower sensitivity and the need for modified peptides to be spiked into each sample both to account for loss during preparation and to accurately quantify the relevant hormone levels.
Serotonin secretion from isolated enterochromaffin cells has been measured by ELISA or carbon fiber amperometry (14, 28, 29). Amperometry enables the detection of individual vesicular release events that trigger discrete currents in the carbon fiber electrode due to the oxidation of serotonin (28, 48).
Transgenic mice
The generation of transgenic reporter mice has enabled a dramatic increase in our understanding of EEC function and has helped to identify and validate a wide range of signaling pathways controlling hormone secretion from the intestine. Most genetically modified mouse models used in EEC research harness cell-specific promoters, such as the promoters for the hormones themselves (e.g., GLP-1, GIP, CCK, somatostatin, and ghrelin), chromogranin A (ChgA), EEC-specific transcription factors (e.g., NeuroD1), or tryptophan hydroxylase (Tph1, required for serotonin biosynthesis) (Fig. 3) (22, 26, 43, 49–57). These EEC-specific promoters are used to drive cell-specific expression of fluorescent reporters/sensors or Cre-recombinase. Interestingly, the ChgA-hrGFP reporter line seems specifically to label enterochromaffin cells even though ChgA is considered to be a marker of most EECs, although this mirrors our previous observation that ChgA staining was low in murine l-cells compared with other EECs (37, 56).
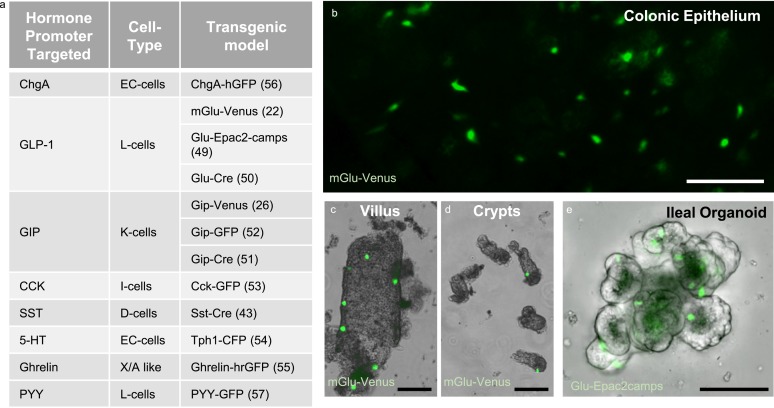
Transgenic EEC models. (a) Table listing the current transgenic models used to identify different EEC types. (b) Glu-Venus (green) mouse tissue showing colonic epithelium (image by L. Billing), (c) an isolated ileal villus, and (d) isolated ileal crypts. (e) Ileal organoid derived from Glu-Epac2-camps mice, with l-cells highlighted by Epac2-camps expression (green). Scale bars, 100 μm. 5-HT, 5-hydroxytryptamine; SST, somatostatin.
When the fluorescent reporter is directly driven by a hormonal promoter, the detection of fluorescence in a cell should reflect current or recent activity of the hormonal promoter, as is seen with mouse strains such as GLU-Venus, GIP-GFP, CCK-GFP, and ChgA-hrGFP (22, 52, 56, 58). Hormone promoters driving Cre-recombinase, by contrast, are actually lineage tracers in the sense that even a brief activation of the hormone promoter and consequent transient expression of Cre-recombinase can activate Cre-inducible elements in the DNA, causing permanent genetic changes that persist for the lifetime of the cell and are passed on to daughter cells. If Cre expression is used to label an EEC population, it does not therefore necessarily reflect ongoing hormone expression. This can be useful when the endogenous cell-specific promoter is too weak itself to drive detectable fluorescence, because it is used instead to activate a fluorescent reporter that is driven by a much stronger promoter. Although, as with all indicators, one might be concerned that chronic overexpression of fluorescent reporters might be toxic or alter the dynamics of the monitored secondary messengers, we have not detected any differences in the responsiveness of our reporter lines to different secretory stimuli and observed similar Ca2+ dynamics when monitoring with GCaMP3 or Fura-2.
Transgenic mouse technologies have allowed researchers to identify the rare EEC cells among the rest of the intestinal epithelial cells, enabling application of a wide variety of analytical methods, including population and single-cell transcriptomics, electrophysiology, and single-cell imaging of intracellular signaling pathways.
Flow cytometry
EECs expressing fluorescent reporters, derived from transgenic mouse models, can be readily separated from their nonfluorescent neighbors by fluorescence-activated cell sorting (FACS) following dispersal of the mucosa by enzymatic digestion or EDTA (22). Flow cytometry gates set on forward scatter and side scatter enable the distinction between cells and debris, and an additional pulse width gate is useful to reduce the collection of cell clusters containing a single fluorescent EEC that appear on the FACS as a single large fluorescent cell. EEC populations of 90% to 95% purity can be achieved by FACS, with the major limiting feature being the quality of the single-cell digest. Additional nuclear stains are frequently used to exclude dead cells.
Flow cytometry can also be used to analyze the proteins produced by individual EECs, in a method that involves fixation and antibody staining of cell suspensions following tissue dispersal (19). The technique enables the identification and quantification of EECs producing, for example, different hormonal combinations or other high abundance proteins that are readily detectable using antibodies. We have also developed protocols to enable transcriptomic analysis of fixed and stained EECs following FACS purification, allowing the characterization of nonmurine species, including human, that do not carry transgenic markers. A similar approach has recently been reported for the isolation of murine chromaffin cells not relying on transgenic labeling (59).
Transcriptomics
FACS-purified EECs have been analyzed at the transcriptomic level by RT-qPCR to quantify preselected transcripts and by microarrays and RNA sequencing to identify all transcripts. RNA-sequencing methods are now sufficiently sensitive to permit analysis of single cells as well as cell populations, although single-cell analysis is still limited by read-depth and the costs of analyzing large numbers of cells individually. By these methods, it has been shown that primary EECs express a wide variety of GPCRs, transporters, and channels that have subsequently been shown to play roles in the function and physiology of EECs (22, 37, 60). Application of single-cell RNA-sequencing methods to investigate EECs was first achieved by random sampling of intestinal organoid cells (61) or primary cells (1) and more recently was applied to duodenal l-cells purified by FACS from the GLU-Venus mouse model (58). These data have highlighted the heterogeneity of EECs with regard to expression of multiple hormones and GPCRs. Enterochromaffin cells in organoids labeled with the ChgA-hrGFP reporter have also been investigated by RT-qPCR and RNA sequencing to identify the channels/transporters and receptors that are differentially expressed compared with the nonfluorescent cell population (37).
Electrophysiology
Electrophysiology evaluates the flow of ions or potential difference across biological tissues or cell membranes. In Ussing chambers, the short circuit current can be used as a measure of net ion transport across the epithelium and has been used to assess PYY secretion triggered by nutrients, hormones, and drugs (6, 9). Locally released PYY binds to NPY1R receptors on enterocytes, thereby lowering intracellular cAMP concentrations and blocking apical chloride secretion, which can be measured by changes in the short circuit current (8).
Early patch clamp studies of STC-1 cells revealed the presence of voltage-gated Ca2+ and K+ currents, but did not find any direct evidence that the cells fired action potentials (62). GLUTag cells, and EECs in primary cultures and organoids, by contrast, were found to be electrically active and capable of firing action potentials dependent on voltage-gated Na+ and Ca2+ channels (22, 36, 37, 60, 63–67). The detection of electrical activity in GLUTag and primary l-cells likely reflects the use of perforated patch rather than whole-cell recordings that retain a more physiological intracellular milieu. Indeed, EECs exhibit very high electrical resistance, and only small leak currents are sufficient to abolish action potential firing. Although patch clamping of colonic primary l-cells (identified using GLU-Venus mice) and GLUTag cells is relatively straightforward because colonic l-cells survive in culture for over a week (64, 67), we found it considerably more difficult to patch clamp duodenal EECs in primary culture because they only survived for a few days and produced substantial quantities of mucus that tended to block the electrodes. Organoid-derived ileal l-cells, however, proved a more robust system for patch clamping, revealing that ileal l-cells, like their colonic counterparts, fire action potentials that are modulated by external stimuli (36). Organoid-derived enterochromaffin cells from ChgA reporter mice also exhibited Na+- and Ca2+-dependent action potentials (37).
Live-cell imaging
Fluorescent dyes and genetically encoded sensors have been used to monitor intracellular concentrations of Ca2+, cAMP, and glucose in EECs in response to stimulus application. Cell-permeant Ca2+ indicators such as Fura-2-AM can be used to load an entire dish of cells and have been used to monitor Ca2+ in a variety of EEC cell lines (67–69). For primary EECs, however, extra steps must be incorporated that permit EEC identification among the mixture of other cell types or include prior cell purification. CCK-expressing cells, for example, have been FACS purified from CCK-eGFP mice and loaded with the Ca2+ indicator Quest Rhod4 to show that CCK-producing cells exhibited Ca2+ responses to ligands of the free fatty acid receptor (FFA) 1 (70), and enterochromaffin cells were purified by Percoll gradients prior to Fluo-4 loading to enable Ca2+ imaging of the enterochromaffin cell population (28–30). More recently, however, we have seen the introduction of genetically encoded sensors that incorporate a binding domain for the signal of interest, fused to one or more fluorescent molecules that exhibit altered fluorescence intensity or fluorescence resonance energy transfer upon ligand binding (25, 50, 71). Genetically encoded sensors provide the advantage that they can be specifically expressed in the target cell of interest using transgenic technologies. ROSA26-GCaMP3 reporter mice, for example, express the Cre-dependent Ca2+ sensor GCaMP3 and can be crossed with mouse strains expressing Cre-recombinase under EEC-specific promoters to enable Ca2+ recordings in EEC populations of interest. Most genetic sensors have not yet, however, been incorporated into Cre-dependent mouse strains, but can be introduced into cell lines by standard transfection methods to enable monitoring of intracellular cAMP or glucose concentration (50, 71). Use of these sensors in primary EECs requires an additional method for cell identification, such as a transgenic EEC-specific fluorescent reporter activated at wavelengths that do not interfere with the sensor or prior cell purification by FACS. Expression of the genetic sensor can also be driven directly by an EEC promoter in a transgenic mouse model, as exemplified by GLU-Epac2-camps, which expresses the cAMP sensor Epac2-camps driven by the proglucagon promoter to allow cAMP monitoring in l-cells (49). These methods have enabled dynamic monitoring of GPCR signaling pathways in live EECs following stimulus application, assessment of net glucose fluxes, and Ca2+ changes downstream of Ca2+ influx or release from intracellular stores.
Assessing Physiological EEC Stimuli by Combined Experimental Techniques
The models and experimental tools described previously have been used to identify a wide range of stimulants and suppressors of gut hormone release, site of interaction of nutrients with EECs, and some of the molecular pathways involved in nutrient detection and hormone secretion. Many of the findings from these studies are summarized in Fig. 4. As an example of how the different techniques have been combined to explore a molecular pathway, we will consider the question of how bile acids trigger GLP-1 secretion.
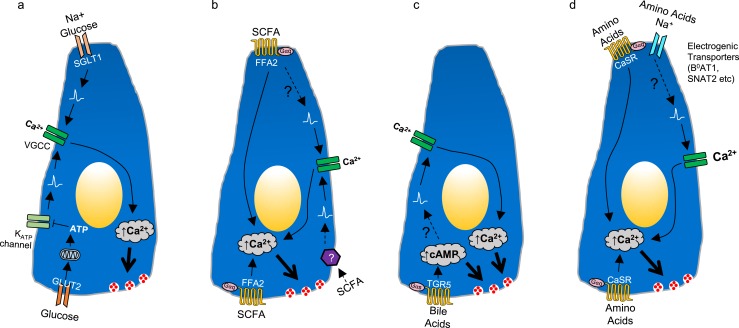
Mechanisms underlying glucose, short-chain fatty acid (SCFA), bile acid, and amino acid sensing in EECs. (a) Glucose sensing in EECs, mediated by two pathways leading to cell depolarization and opening of voltage-gated calcium channels (VGCC), triggering Ca2+ influx and hormone secretion. Glucose cotransport with Na+ through apical sodium glucose-linked transporter 1 can trigger depolarization and secretion, whereas glucose influx via glucose transporter 2 (GLUT2) increases ATP levels and closes KATP channels, potentially modifying the effectiveness of other depolarizing stimuli. (b) SCFA sensing in EECs mediated by FFA2 Gαq signaling, thereby increasing Ca2+ and triggering hormone release. An unidentified pathway might also trigger EEC depolarization and opening of VGCC, as suggested by experiments on perfused intestine. (c) Bile acid sensing is mediated by basolateral G-protein–coupled bile acid receptor (GPBAR) 1 signaling through Gαs, increasing cAMP and triggering hormone secretion. GPBAR1 activation also promotes depolarization and opening of VGCCs. (d) Amino acid sensing in EECs is mediated by multiple pathways. Highlighted in the figure are calcium-sensing receptor Gαq signaling and electrogenic amino acid uptake, which trigger subsequent depolarization and opening of VGCCs.
Bile acids have been demonstrated to increase GLP-1 secretion from GLUTag cells, primary colonic l-cells, ileal organoid-derived l-cells, ileal tissue mounted in Ussing chambers, and perfused intestine (2, 5, 10, 36, 72). Knockdown studies in GLUTag cells and the use of tissues from knockout mice confirmed that the major receptor involved in the bile acid–triggered GLP-1 secretion is the G-protein–coupled bile acid receptor (GPBAR) 1 (also known as TGR5), which is highly expressed in primary l-cells (2, 72, 73). Consistent with the known Gαs coupling of GPBAR1, bile acids and the GPBAR1 agonist GPBAR-A increased intracellular cAMP levels in GLUTag cells transiently expressing the cAMP fluorescence resonance energy transfer sensor Epac2-camps, as well as in primary l-cells in organoids derived from the GLU-Epac2-camps mouse model (2, 36, 72). The use of 2D organoid cultures allowed electrophysiology assessment of the bile acid receptor in ileal l-cells, revealing that GPBAR1 agonism increased action potential firing and enhanced the activity of voltage-gated Ca2+ channels, thereby potentiating Ca2+ signals triggered by FFA1 agonists, as measured in organoid l-cells from GLU-Cre/GCaMP3 mice (36). A key question for the potential translational exploitation of GPBAR1 as a drug target for increasing endogenous GLP-1 secretion is whether small-molecule receptor agonists could target the receptor from the apical membrane, thus enabling the development of nonabsorbable agonists with low side effect profiles. Ussing chamber and perfused intestine experiments, however, demonstrated that bile acids only enhance GLP-1 secretion following their absorption across the epithelium and that nonabsorbable GPBAR1 agonists are only effective when applied from the basolateral direction (2, 5, 10). Nevertheless, activation of GPBAR1, particularly in combination with FFA1, provides strong stimulus to l-cells that deserves further exploration as a potential therapeutic strategy.
Conclusion
Compared with the situation 20 years ago, when studies on gut hormone secretion were largely restricted to the use of whole animals, perfused intestinal preparations, Ussing chamber recordings, and cell lines, EEC research has exploded recently both in terms of the techniques and protocols available to researchers and our understanding of the molecular pathways used by EECs for stimulus secretion and detection. The use of high-throughput systems such as cell lines and primary cultures has enabled the identification of a wide range of nutrient-dependent and nonnutrient-dependent pathways involved in the stimulation and inhibition of hormone release. Increased use of intestinal organoids is hoped to facilitate more translational human-based research and reduce the need for ex vivo animal-based studies. The new technologies that use organoid cultures to generate an in vivo type intestinal architecture with intact barrier function provide promising avenues for the study of human and murine EEC function.
Acknowledgments
Financial Support:This work was funded by Medical Research Council Grants MRC_MC_UU_12012/3 (to F.R.) and MRC_MC_UU_12012/5 (to F.M.G.) and Wellcome Trust Grants 106262/Z/14/Z (to F.M.G.) and 106263/Z/14/Z (to F.R.).
Disclosure Summary: The laboratories of F.R. and F.M.G. have received research funding from AstraZeneca/MedImmune within the last 2 years. Mice models from laboratories the F.R. and F.M.G. have been made commercially available via Cambridge Enterprise. F.M.G. is a consultant for Kallyope. The remaining author has nothing to disclose.
Abbreviations:
2D | two-dimensional |
3D | three-dimensional |
CCK | cholecystokinin |
ChgA | chromogranin A |
EEC | enteroendocrine cell |
FACS | fluorescence-activated cell sorting |
FFA | free fatty acid receptor |
GI | gastrointestinal |
GIP | glucose-dependent insulinotropic peptide |
GLP-1 | glucagon-like peptide-1 |
GPBAR | G-protein–coupled bile acid receptor |
GPCR | G-protein–coupled receptor |
INSL5 | insulin-like 5 |
LC-MS/MS | liquid chromatography–tandem mass spectrometry |
PYY | peptide YY |
RT-qPCR | quantitative RT-PCR |
References
Articles from Endocrinology are provided here courtesy of The Endocrine Society
Citations & impact
Impact metrics
Article citations
Single Cell Analysis of Human Colonoids Exposed to Uranium-Bearing Dust.
Environ Health Perspect, 132(5):57006, 21 May 2024
Cited by: 0 articles | PMID: 38771937 | PMCID: PMC11108582
A human stomach cell type transcriptome atlas.
BMC Biol, 22(1):36, 14 Feb 2024
Cited by: 0 articles | PMID: 38355543 | PMCID: PMC10865703
Cellular mechanisms of incretin hormone secretion.
J Mol Endocrinol, 72(4):e230112, 22 Feb 2024
Cited by: 4 articles | PMID: 38240302 | PMCID: PMC10959011
Review Free full text in Europe PMC
The intestine as an endocrine organ and the role of gut hormones in metabolic regulation.
Nat Rev Gastroenterol Hepatol, 20(12):784-796, 25 Aug 2023
Cited by: 18 articles | PMID: 37626258
Review
Dorothy Hodgkin lecture 2023: The enteroendocrine system-Sensors in your guts.
Diabet Med, 40(12):e15212, 15 Sep 2023
Cited by: 1 article | PMID: 37638546 | PMCID: PMC10946932
Review Free full text in Europe PMC
Go to all (17) article citations
Similar Articles
To arrive at the top five similar articles we use a word-weighted algorithm to compare words from the Title and Abstract of each citation.
Enteroendocrine Cells: Chemosensors in the Intestinal Epithelium.
Annu Rev Physiol, 78:277-299, 06 Oct 2015
Cited by: 277 articles | PMID: 26442437
Review
Gut chemosensing mechanisms.
J Clin Invest, 125(3):908-917, 09 Feb 2015
Cited by: 129 articles | PMID: 25664852 | PMCID: PMC4362249
Review Free full text in Europe PMC
Enteroendocrine Systems That Sense Nutrients in the Gut and Control the Body.
J Nutr Sci Vitaminol (Tokyo), 68(supplement):S5-S7, 01 Jan 2022
Cited by: 0 articles | PMID: 36437016
Diet: friend or foe of enteroendocrine cells--how it interacts with enteroendocrine cells.
Adv Nutr, 3(1):8-20, 05 Jan 2012
Cited by: 47 articles | PMID: 22332097 | PMCID: PMC3262619
Review Free full text in Europe PMC
Funding
Funders who supported this work.
Medical Research Council (5)
Enabling technologies
Professor Giles Yeo, University of Cambridge
Grant ID: MC_UU_00014/5
Grant ID: MRC_MC_UU_12012/5
The Enteroendocrine System in Health and Disease
Prof Fiona Gribble, University of Cambridge
Grant ID: MC_UU_12012/3
Enabling technologies
Professor Giles Yeo, University of Cambridge
Grant ID: MC_UU_12012/5
Entero-endocrinology including the effects of bariatric surgery
Professor Frank Reimann, University of Cambridge
Grant ID: MC_UU_00014/3
Medical Research Foundation (2)
Grant ID: MRC_MC_UU_12012/3
Grant ID: MRC_MC_UU_12012/5
Wellcome Trust (4)
Grant ID: 106262/Z/14/Z
Grant ID: 106263/Z/14/Z
Physiology of the Enteroendocrine System.
Professor Frank Reimann, University of Cambridge
Grant ID: 106262
Grant ID: 106262/ Z/14/Z