Abstract
Free full text

Regulatory T cells in embryo implantation and the immune response to pregnancy
Abstract
At implantation, the embryo expresses paternally derived alloantigens and evokes inflammation that can threaten reproductive success. To ensure a robust placenta and sustainable pregnancy, an active state of maternal immune tolerance mediated by CD4+ regulatory T cells (Tregs) is essential. Tregs operate to inhibit effector immunity, contain inflammation, and support maternal vascular adaptations, thereby facilitating trophoblast invasion and placental access to the maternal blood supply. Insufficient Treg numbers or inadequate functional competence are implicated in idiopathic infertility and recurrent miscarriage as well as later-onset pregnancy complications stemming from placental insufficiency, including preeclampsia and fetal growth restriction. In this Review, we summarize the mechanisms acting in the conception environment to drive the Treg response and discuss prospects for targeting the T cell compartment to alleviate immune-based reproductive disorders.
Introduction
Within days of conception, the embryo attaches to the uterine lining, and trophoblast cells invade into the uterine decidua (1). A finely controlled developmental program then unfolds, with successive waves of trophoblast invasion, proliferation, and differentiation to form a mature placenta that sustains fetal growth throughout gestation.
This remarkable feat occurs in apparent defiance of the mother’s immune response. Abundant immune cells reside in the decidua in close contact with infiltrating trophoblasts, and paternally derived alloantigens are expressed in the developing placental and fetal tissues. Far from the immune evasion or systemic immune suppression historically invoked to explain maternal-fetal tolerance (2), maternal immune cells exhibit priming toward fetal alloantigens (3–5) and actively participate in many aspects of establishing, sustaining, and terminating pregnancy (6).
Mammalian pregnancy cannot be readily reconciled with the classical self/non-self discrimination theory or alternative models of immune regulation (7). Central tolerance, wherein self-reactive lymphocytes are deleted in the thymus (8), is not relevant, since fetal alloantigens are not encountered outside of the reproductive context. Instead, a range of specialized mechanisms in both the innate and adaptive immune compartments mediate an active state of functional tolerance that permits fetal and maternal cells to coexist. Key suppressive mechanisms include attenuated placental expression of polymorphic MHC molecules (9, 10); placental release of antiinflammatory and protolerogenic hormones, cytokines, and immune modulatory molecules (11–13); and specialized decidual regulation of immune cell access and egress (refs. 14, 15, and for additional information, see refs. 6, 16–18).
There is a strong imperative to define how pregnancy tolerance is established, as an immune etiology is implicated in common reproductive conditions including recurrent implantation failure and miscarriage (19–21), as well as later-onset gestational disorders that arise as a result of disturbed implantation and placental morphogenesis (22–24). Recurrent implantation failure occurs when overtly healthy embryos fail to implant normally and is the cause of infertility in approximately 10% of women seeking in vitro fertilization (IVF) treatment (25). Recurrent pregnancy loss, defined as loss of three or more karyotypically normal embryos before 20 weeks’ gestation, occurs in approximately 1% to 2% of women (26). Preeclampsia affects 3% to 5% of pregnancies (27) and is a major cause of morbidity and mortality for women and infants, particularly in low- and middle-income countries, and it is often accompanied by fetal growth restriction and preterm birth (28, 29).
Tolerance arises in the preimplantation phase of early pregnancy and appears to require a unique dialog involving maternal-, paternal-, and conceptus-derived signals and specialized anatomical elements of the reproductive tissues (30, 31). Their interaction drives a cascade of immune changes that initiate prior to conception, persist through gestation, and culminate with birth (6, 18). Innate immune cells, particularly macrophages (32), DCs (33), and a unique population of NK cells with a CD56hiCD57lo phenotype (uterine NK cells, or uNK cells) (34), are abundant in the decidua in the luteal phase of the menstrual cycle when implantation commences. These cells influence placental development through immune regulation, provision of growth factors, and facilitation of adaptations in the uterine vasculature to support trophoblast invasion. Innate immune cells exhibit altered phenotypes and contribute to pathophysiological processes in many gestational conditions (refs. 35, 36, and for more information, see refs. 17, 37, 38).
The adaptive immune response is also critical to pregnancy tolerance (refs. 39–41 and for more information, see refs. 18, 42), and an imbalance of T regulatory cells (Tregs) and effector T cells (Teffs) is emerging as a key underpinning factor in common fertility and obstetric disorders (19–21, 23, 24). Tregs are well known for their capacity to limit excessive inflammation and recalibrate tissue homeostasis after insult or injury, as well as to suppress Teff reactions to self or non-self antigens (43, 44). Tregs exert potent antiinflammatory, immune-regulatory, and vaso-regulatory functions (43–45) relevant to establishing pregnancy. Also significant is their distinct phenotypic plasticity, or capacity to transdifferentiate into Th17 cells (46, 47), which provides a potential mechanism for strategic female reproductive investment (48).
In women, T cells comprise 10% to 20% of decidual immune cells in the first trimester (49). Many of these are CD8+ T cells, including regulatory subsets (50, 51). Among the CD4+ T cells, approximately 10% to 30% express the Treg transcription factor FOXP3, which constitutes a substantial enrichment compared with its expression in peripheral blood (52–54). Decidual Th1 cell frequencies are moderately elevated, while Th17 and Th2 cells are generally not enriched, indicating a mild inflammatory environment controlled by Tregs (53, 55). The Tregs are composed of both thymus-derived Tregs (tTregs) and peripheral Tregs (pTregs) and exhibit phenotypic heterogeneity according to the cycle and pregnancy phase (36, 56, 57). Uterine recruitment of Tregs in preparation for conception commences in the proliferative phase of each cycle, with an estrogen-driven increase peaking at ovulation (58). After increasing in early pregnancy, decidual Tregs remain elevated through mid-gestation before declining prior to birth (52, 53, 59), with peripheral blood Tregs following a broadly similar pattern (60, 61).
In reproductive disorders, insufficient numbers of Tregs or impaired function is a common feature (19, 20, 23), with a counteractive increase in Teffs (21, 24). Compelling evidence that Treg deficiency is causal in pregnancy loss comes from animal models (39, 62–64). An underlying T cell etiology in women is supported by correlations with prior sexual and reproductive history (65) and by couple-specific, HLA-linked dispositions to reproductive conditions (66–68), consistent with a protective effect of adaptive immune “memory” for partner histocompatibility antigens.
In this Review, we describe the current understanding of Tregs as master regulators of pregnancy tolerance, focusing on events surrounding conception that critically impact the availability and function of Tregs for implantation and later gestation. We explain the evidence for Tregs in the pathophysiology of infertility and obstetric disorders, discuss the origins of Treg deficiency in some women, and speculate on the prospect of targeting Tregs to address common reproductive and obstetric conditions.
The immune response and embryo implantation
Normal fetal growth depends on establishing a robust placenta to nurture the fetus; remarkably, two-thirds of genetic mutations identified as embryonically lethal in mice affect placental morphogenesis (69). Even minor derangement of the developmental program of the placenta can cause later miscarriage or set a trajectory toward preeclampsia (22, 27, 70). These conditions can be traced back to aberrant interactions between trophoblasts and the uterine decidua at conception and implantation (70–72).
Critical to implantation is an adequate decidual response. Immune cells are instrumental, with reciprocal interactions between DCs, uNK cells, and invading trophoblasts (73, 74) in response to hormonal triggers to transform the uterine lining in the luteal phase (1, 71, 72). Extensive contact between trophoblasts and immune cells in the decidua is common to all placental mammals (75) but is most conspicuous with invasive hemochorial placentation, as occurs in mice and humans (17). A sufficient number of decidual immune cells must acquire appropriate phenotypes to support the decidual response and remodel the local vascular network for embryo attachment and implantation (71, 76).
Leukocyte recruitment builds during the estrogen-dominated periovulatory phase, and a threshold level of inflammatory activation may facilitate the generation of a receptive endometrium (77, 78). However, within days of conception, inflammation must be contained and controlled in order for decidualization and implantation to progress (72). The capacity to resolve decidual inflammation has evolved as a key feature underpinning placentation in viviparous mammals (79), and disturbance to the dynamic balance between pro- and antiinflammatory mediators is a hallmark of impaired implantation (21, 80). Through their potent antiinflammatory actions, Tregs appear to be critical for controlling inflammation in early pregnancy and establishing a receptive decidual environment (refs. 18, 81, and Figure 1).
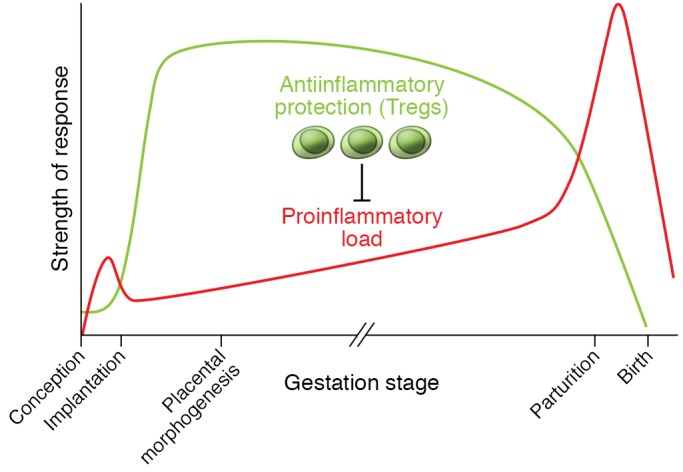
Mouse models show that Tregs act to suppress inflammation, prevent adverse effects of antifetal alloantigen Teff cells, and allow vascular adaptations required for placental morphogenesis (39, 41, 62, 63, 82, 87). Tregs arise as a consequence of events during the inflammation-like response in the periconception phase, and their abundance, suppressive function, and stability are impacted by events at conception and in the preimplantation phase (39, 83–85, 135, 137). Tregs sustain an antiinflammatory environment until a decline, associated with the inflammation events of parturition and birth, is triggered (40, 64, 88, 89). Decidual Tregs in pregnant women show kinetics and regulatory mechanisms comparable to those in mice (36, 52–54, 56, 58, 59). Recurrent implantation failure, recurrent miscarriage, preeclampsia, and in utero growth restriction are all linked with insufficient numbers, reduced suppressive function and/or instability of Tregs (19–21, 23, 24), and excessive inflammation in the uterus and/or gestational tissues (21, 27, 80).
Tregs as essential mediators of pregnancy tolerance
Experiments in mice provide compelling evidence that Tregs are essential for the antiinflammatory transition accompanying implantation and placental development. Initially, this was shown by transferring T cells depleted of CD4+CD25+ Tregs into pregnant T cell–deficient mice (39). In the absence of Tregs, allogeneic fetuses were uniformly rejected, but Tregs were not essential when fetuses shared maternal MHC (39). Likewise, depletion of CD25+ T cells using the PC21 mAb on the day of mating caused a dramatic increase in activated CD8+ and CD4+ T cells in para-aortic lymph nodes (PALNs) draining the uterus, and few fetuses survived in allogeneic pregnancies (82).
Treg dynamics in mice mirror those in human pregnancy, providing a useful model for key regulatory features (83, 84). Treg increases at implantation are consistently greater with allogeneic fetuses, indicating fetal antigen–driven expansion, and are accompanied by specific suppression of anti-paternal alloantigen responses (83, 85). Male-specific minor antigens such as H-Y contribute to driving the Treg response, with male fetuses being more vulnerable to Treg depletion (86).
Studies in which Tregs are depleted at various time points show that the pre- and peri-implantation phase is the most vulnerable. Anti-CD25 Ab administered shortly after mating causes complete implantation failure (62, 87). Depletion of FOXP3+ cells from Foxp3-Dtr mice during early placentation increases later fetal resorption (41, 63), but mid-gestation depletion increases fetal death only moderately (40), unless mice receive a second-hit inflammatory challenge (64, 88, 89). Mice deficient in T cells due to Rag1-null mutation are susceptible to inflammation-induced fetal loss, which is mitigated by CD4+ T cells that differentiate into Tregs after transfer (64). Tregs also protect against fetal loss elicited by activated invariant NK T cells (iNKT cells) (88) or after IL-10 depletion from NOD mice (89). mAb-mediated depletion of CD25+ cells in mid-gestation has a less severe impact, but this may be because Teffs as well as Tregs express CD25 and are removed by PC61 mAb treatment (62, 90).
Mouse models with a high rate of spontaneous fetal loss reinforce the critical importance of Tregs for implantation. CBA/J females mated with DBA/2J males have fewer Tregs and elevated decidual Th1 cell numbers, attributable to DBA/2J expression of the superantigen MIs (87, 91). Adoptive transfer of Tregs from CBA/J females mated with BALB/c males elevates decidual Tregs to restore fetal viability (87), but only if Tregs are transferred before embryo implantation (87). These findings confirm that Tregs have essential roles in the uterus, particularly in the peri-implantation period, consistent with managing the antiinflammatory transition required for embryo receptivity (Figure 1).
Mechanisms by which Tregs mediate implantation success
Mouse studies indicate at least three mechanisms by which Tregs facilitate implantation and placental development (Figure 2). Selective depletion of Tregs, or induction of overwhelming Teff responses, reveals a key function of Tregs in preventing destructive Teff responses to fetal alloantigens (41, 92–94). Paternal antigen–reactive CD8+ Teffs arise in uterus-draining lymph nodes in early pregnancy but normally don’t exhibit cytotoxicity in mice (95) or women (5). However, excessive IFN-γ and IL-2 at priming can promote the generation of cytotoxic CD8+ T cells that later drive fetal loss (96), consistent with evidence of CD8+ T cell–associated trophoblast damage in early-onset preeclampsia (59). Unrestrained Teffs adversely affect placental development in a fetal antigen–independent manner, presumably through inflammatory cytokine release (96), as well as through antigen-dependent trophoblast cytotoxicity (97, 98). Decidual Tregs secrete IL-10 and TGF-β and express CD25, CTLA4, and PD-L1 (53, 88, 99, 100), all of which are hallmark mediators of Treg suppression that probably contribute to Teff constraint in early pregnancy (88, 101).
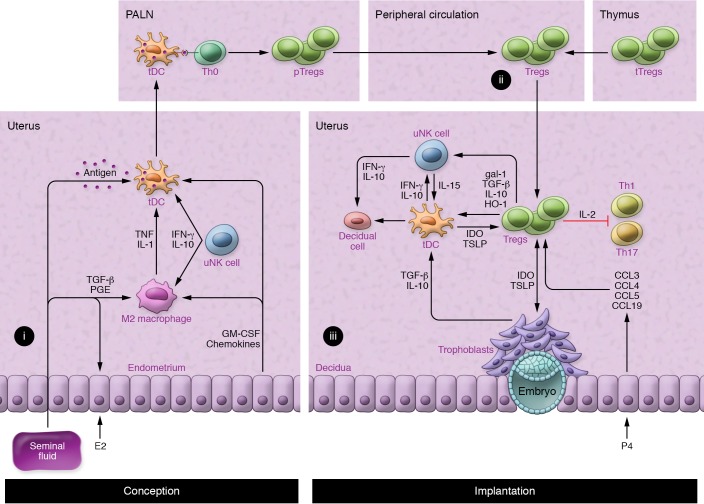
(i) Estrogen (E2) and seminal fluid induce the recruitment of macrophages and DCs, which acquire M2 and tDC phenotypes in response to TGF-β and prostaglandin (PGE) in seminal fluid; granulocyte-macrophage CSF (GM-CSF) and chemokines released by uterine epithelial cells; and IFN-γ and IL-10 originating in uNK cells (38, 105, 128). tDCs take up paternal alloantigens in seminal fluid and traffic to the uterus-draining PALNs (90). (ii) In the PALNs, tDCs present antigen to naive Th0 cells, which become activated, proliferate, and differentiate into pTregs before release into the peripheral blood (39, 84, 90, 137). (iii) An expanded pool of peripheral blood pTregs and tTregs (41, 83, 139) is recruited and retained in the uterus prior to and during embryo implantation in response to epithelial cell–derived CCL3, CCL4, CCL5, and CCL19 (85, 137). Here, Tregs inhibit the activation and function of Th1 and Th17 cells by sequestering IL-2 and other suppressive mechanisms (53, 88, 99, 100) and control inflammation by the release of TGF-β, IL-10, and HO-1 to interact with DCs and uNKs (53, 88, 99, 104, 105). This in turn impairs Th1 survival, promotes further Treg generation (39, 102, 103, 105), and potentially influences decidual transformation and receptivity to embryo implantation (108–110). Treg phenotype and stability are reinforced by IDO and TSLP from tDCs and trophoblasts (111). gal-1, galectin-1; P4, progesterone.
Second, Tregs regulate other leukocytes and nonhemopoietic cell lineages to influence decidual support of implantation (Figure 2 and ref. 42). Notably, Tregs promote antiinflammatory and tolerogenic phenotypes in alternatively activated (M2) macrophages and tolerogenic DCs (tDCs) through TGF-β, IL-10, and CTLA4-mediated mechanisms. Indoleamine 2,3-dioxygenase (IDO) produced by tDCs impairs Th1 cell survival (102, 103). Additionally, Tregs release heme oxygenase-1 (HO-1), which targets uterine DCs and maintains their immature state (104). In turn, these M2 and tDC phenotypes promote further Treg generation (104, 105).
In mice, DCs are key regulators of decidual transformation (73, 76), and through regulation of the uterine DC phenotype (104), Tregs may influence the extent and quality of the decidual response (Figure 2). uNK cells also promote decidualization (106) and regulate decidual artery remodeling (107). Tregs may be important regulators of the uNK phenotype and function at implantation (108), since Tregs control IL-15 release from DCs (109) and suppress uNK cytolytic activity (110). Invading trophoblasts engage with Tregs in a reciprocal interaction that modulates the secretory phenotype of both lineages (111). These coordinated interactions allow Tregs to constrain and limit the inflammatory damage and oxidative stress associated with trophoblast invasion (112).
Third, Tregs are emerging as important regulators of the maternal vascular changes that are essential for normal placental development and adequate placental access to maternal blood. Recent studies have shown that Tregs are critical for modulating cardiovascular function and vascular homeostasis (45). How this relates to the vascular changes required for pregnancy is detailed below.
Tregs and maternal vascular adaptation for placental development
uNK cells regulate decidual blood vessel remodeling to enable vessel dilatation, trophoblast invasion, and secure placental access to maternal blood (ref. 107 and Figure 3). Mouse models show that T cells can interact with uNK cells to influence the maternal hemodynamic response to pregnancy (113, 114), and adverse effects of uNK deficiency on decidual vessel remodeling are exacerbated when T cells are also deficient (115).
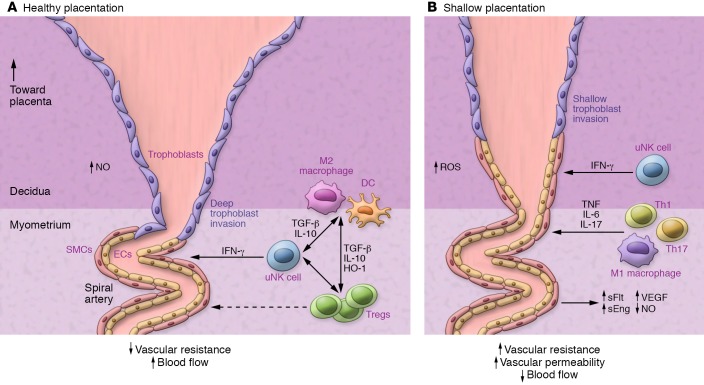
(A) Decidual uNK cells release IFN-γ to regulate decidual vascular remodeling associated with extravillous trophoblast invasion as well as displacement of endothelial cells and smooth muscle cells (SMCs) (107). Tregs release TGF-β, IL-10, and HO-1 to suppress inflammatory activation and modulate decidual uNK, macrophage, and DC phenotypes (53, 88, 99, 104, 105). The result is reduced vascular resistance and increased blood flow to the developing placenta. (B) When Tregs are deficient, decidual vascular remodeling is impaired (41, 63, 116), particularly when uNK cells are also dysregulated (115). The effects of Treg cell deficiency may be mediated by elevated decidual Th1 and Th17 cells and/or M1 macrophages (41, 97, 99). The result is an elevated synthesis of the proinflammatory cytokines TNF, IL-6, and IL-17 that elicit elevated vascular resistance and permeability, causing inflammatory injury accompanied by elevated soluble Flt (sFlt), soluble endoglin (sEng), VEGF, and NO, which in turn impair placental development, resulting in fetal growth restriction (63, 119, 120). Treg deficiency is thus implicated in contributing to “shallow placentation,” the upstream cause of preeclampsia and other gestational disorders in women (27, 70, 176). ET-1, endothelin-1.
Treg-deficient mice show consistent impairment in uterine spiral arterial modification, reduced placental blood flow, and fetal growth restriction (Figure 3 and refs. 41, 63, 116). Acute depletion of FOXP3+ Tregs in early pregnancy causes later uterine artery dysfunction associated with increased production of the vasoconstrictor endothelin-1 (ET-1) (63). A particular function for pTregs is indicated by CNS1-null mice, in which deficiency for the Foxp3 enhancer impairs remodeling of uterine spiral arteries and placental development (41). Poor trophoblast invasion and failure to transform spiral arteries is also seen in mice after depletion of proangiogenic, neutrophil-induced Tregs (116).
Hypertensive mouse models indicate that Tregs limit inflammatory injury and oxidative stress to reduce blood pressure and protect against hypertensive damage (117, 118). Tregs also protect against hypertension in pregnancy (63, 119), with depletion of FOXP3+ Tregs during early pregnancy perturbing the regulation of arterial blood pressure in mid-gestation (63). Rat models of preeclampsia show that Tregs suppress inflammation to reduce blood pressure and progression to preeclampsia-like symptoms. In the reduced uterine perfusion pressure (RUPP) model, reduced uterine artery blood flow results in placental ischemia and oxidative stress and hypertension. This is accompanied by reduced decidual and placental Tregs, elevated CD4+ T cells and Th17 cells, and increased circulating vasoactive and inflammatory factors TNF, IL-6, and IL-17, resulting in fetal growth restriction (refs. 119, 120 and Figure 3). The preeclampsia symptoms are T cell dependent: they cannot be induced in T cell–deficient athymic rats, but can be induced by transfer of Th17 cells (120), and are mitigated by Tregs transferred from pregnant controls (119). Treatments that boost endogenous Tregs, including IL-10 (121) or low-dose CD28 superagonist (119), also reduce hypertension and fetal growth restriction in the RUPP model. In a rat model of preeclampsia, induction of Tregs using a CD28 superagonist treatment alleviates maternal and fetal disease, most effectively in the preimplantation phase (122).
Treg origins and antigen specificity in early pregnancy
The two lineages of Tregs required for implantation have different ontogenies: tTregs emerge from the thymus fully competent to suppress responses to self and alloantigens, while pTregs differentiate from conventional naive CD4+ precursors in peripheral lymph nodes or tissues (123) following presentation of cognate antigen by tDCs in the presence of IL-2 and TGF-β (124). Both pTregs and recent thymic emigrant (RTE) tTreg populations require antigen contact to activate full suppressive function and memory (125).
Expansion of CD4+Helios+FOXP3+ pTregs predominantly accounts for the Treg expansion in blood and decidua in early human pregnancy (36). This is consistent with nonredundant functions for pTregs shown in CNS1-null mice, wherein elevated fetal loss is attributable to pTreg deficiency (41). Helios+ tTregs, as opposed to pTregs, may be preferentially recruited into first trimester decidua (56). Among peripheral blood tTregs, the CD45RA+CD31+ RTE population expands in the first trimester and differentiates into CD45RA–CD31– memory Tregs (57).
By using tetramer-based enrichment, selective stimulation and expansion of endogenous Tregs with fetal alloantigen specificity can be demonstrated initially in the PALNs and then decidua in mice (40), explaining why fetal-maternal MHC disparity is an important determinant of Treg numbers (126). Antigen-experienced Tregs retain protective memory for fetal MHC antigen and rapidly reaccumulate during second pregnancies (40). Although antigen is required for Treg generation, the antigen independence of the effector functions of Tregs confers bystander suppression and infectious tolerance that protect a wider array of fetal and placental antigens than the antigens against which the Tregs were initially primed (127).
Contact with conceptus alloantigens must occur under conditions that favor stable Treg (not Teff) development. pTregs require the support of tDCs to differentiate from naive Th0 cells. A tolerogenic phenotype is imposed on uterine tDCs by TGF-β, granulocyte-macrophage–CSF (GM-CSF), IL-10, galectin-1 (gal-1), and prostaglandin E (refs. 38, 100, 128, and Figure 2). Treg-derived IL-10, TGF-β, and HO-1 induce tDC and M2 macrophages to express IDO and sustain pTreg generation (102–104). Decidual Tregs also express CTLA4 (53, 105), which downregulates the DC costimulatory molecules CD80 and CD86 needed for Teff activation (43). uNK cells contribute to the reinforcement of protolerogenic M2 through IFN-γ–mediated promotion of IDO expression in decidual M2 macrophages (105) and IL-10–mediated stabilization of tDCs (76). A PD-1–PD-L1 interaction is essential for Treg-mediated protection of alloantigenic fetuses, as shown by fetal death after PD-L1 blockade (129) or in PD-L1–null mutant mice, which can be reversed by WT Tregs (130). Trophoblasts reinforce the tDC phenotype and drive local Treg differentiation by inducing DC production of the cytokine thymic stromal lymphopoietin (TSLP) (111).
The significance of antigen exposure for Treg effector function at implantation is evidenced by studies in abortion-prone mice. Tregs are only effective in rescuing fetal loss when transferred from donors carrying MHC-matched fetuses, whereas antigen-inexperienced donor Tregs from nonpregnant mice are ineffective (87). Consistent with a role for fetal alloantigens in facilitating uNK and Treg responses, studies in congenic mice show that fetal-maternal MHC mismatch promotes decidual vascular adaptations and facilitates fetal growth (9). Nevertheless, endogenous antigens such as hyaluronidase or microbial antigens might also influence the activation and expansion of Tregs in early pregnancy (131).
The majority of T cells in the human decidua have a memory phenotype (CD45RA– or CD45RO+) (51, 132). HLA-C is the only polymorphic HLA expressed in human trophoblasts, and fetal-maternal HLA-C mismatch is associated with elevated decidual Tregs (4) and maternal protection from preeclampsia (68). Many decidual Tregs show fetal HLA-C antigen specificity (54, 133), but specificity to reproductive and other antigens has not been evaluated.
Seminal fluid priming of Tregs
In mice, appropriate conditions for T cell antigen priming occur in two waves during the reproductive process. A first exposure to paternal alloantigens that will later be expressed by the conceptus occurs following contact with seminal fluid at coitus, which primes the activation of antigen-specific Tregs (84). Once placental morphogenesis is complete in mid-gestation and maternal blood contacts the syncytiotrophoblast surface, fetal antigens in the form of placental exosomes are released into the maternal blood, providing a second surge of antigen exposure (134).
The two stages of T cell activation can be tracked in mice expressing transgenic T cell receptors (TCRs) that are reactive with surrogate paternal antigens. Mating with male mice engineered to constitutively express OVA in semen elicit a pulse in the proliferation of OVA-reactive CD4+ and CD8+ T cells within the PALNs, followed by a progressive gain in the postimplantation phase once OVA+ placental cells contact maternal blood (90). Release of placental microparticles containing OVA sustains a progressively systemic T cell response until the postpartum phase (90). A similar pattern of T cell responsiveness is seen in T cell–transgenic models tracking female responses to paternally derived H-Y antigen (135).
Seminal fluid contains high levels of TGF-β as well as TLR4 ligands, which provoke an inflammation-like response in the female reproductive tract (136), eliciting DCs and macrophages that take up male antigens, traffic to the PALNs, and present antigen to naive T cells (ref. 90 and Figure 2A). This provokes a wave of T cell activation corresponding with a 2-fold expansion in Tregs detected within days of conception in the PALNs and then the spleen and peripheral blood (refs. 39, 84, 137, and Figure 2B). Circulating Tregs then accumulate in the uterus (39) in response to CCL3, CCL4, CCL5, and CCL19 secreted by epithelial cells (85, 137) and may undergo further rounds of proliferation (39) to induce a state of hypo-responsiveness to paternal alloantigens in time for embryo implantation (refs. 84, 135, and Figure 2C).
Mouse studies imply that seminal fluid priming is a key step for expansion of the pTreg population in early pregnancy (41). Consistent with this, pregnancies sired in the absence of seminal fluid contact have poor outcomes (138). The size and suppressive competence of the pTreg pool are determined by the strength of the antigenic challenge and the cytokine context in which antigen contact occurs, parameters determined by seminal fluid composition as well as female tract factors. The plasma fraction of seminal fluid is instrumental in Treg activation, as surgical excision of the seminal vesicle glands ablates the maternal response (135, 137) and abrogates the paternal alloantigen hyporesponsiveness seen after mating with intact males (84).
A population of tTregs also expands systemically prior to conception and embryo implantation (83, 139). These cells accumulate in the uterus and PALNs during the estrous stage of the reproductive cycle in mice in response to increases in estradiol (E2) at ovulation (139). The antigen specificity of tTregs and whether they can be further stimulated by tDC presentation of seminal fluid or trophoblast antigens is not clear.
In women, vaginal intercourse elicits an immune response to seminal fluid, with elevated cytokine expression, immune cell recruitment, and T cell activation in the cervix (140, 141). Human seminal fluid contains soluble HLA antigens and high levels of TGF-β among an array of immune regulatory cytokines (141). Analysis of peripheral blood T cells provides evidence that prior seminal fluid contact contributes to the priming of paternal antigen–specific Tregs (54) to build on the follicular-phase Treg pool expansion (58). Although it is yet to be proven that seminal fluid induces pTregs in vivo, seminal fluid has been demonstrated to skew DCs to a tDC phenotype and induce Tregs in vitro (141–143). A priming effect of seminal fluid explains the benefit of seminal fluid contact for implantation success in IVF treatment cycles (144) and the protective effect in preeclampsia of sexual cohabitation with the conceiving partner (145, 146), particularly when maternal-fetal HLA sharing is greater (68).
T cell imbalance in unexplained infertility, miscarriage, and preeclampsia
In women with recurrent miscarriage, the luteal-phase endometrium exhibits elevated expression of the inflammatory mediators IL-1B, TNF, and IFN-γ and reduced IL-4, IL-6, IL-10, IL-6 family cytokine LIF, and VEGF (147, 148), and this is accompanied by altered uNK and T cells (72, 149–151) as well as evidence of uterine vascular dysfunction and reduced expression of key angiogenic regulators (152). Together, these observations point to dysfunctional receptivity to implantation stemming from failure to transition to an antiinflammatory and proangiogenic immune environment. This dysfunction is associated with reduced numbers of Tregs in decidua (20, 153–155) and peripheral blood (58, 155, 156) compared with numbers in fertile women. Conversely, IL-17–producing T cells in peripheral blood and decidua are increased (21, 155), implicating a systemic immune imbalance. Reduced Tregs may precede miscarriage onset, with low circulating Treg numbers in early pregnancy predicting a risk of loss in women with a miscarriage history (157). Tregs from women with recurrent miscarriage exhibit reduced suppressive function (58), with fewer CD45RA– cells among the CD45RA–FOXP3hi effector pool (56) and reduced expression of CTLA4 (153) and Ubc13, an enzyme important for Treg stability (158, 159). Conversely decidual Th17 cell numbers are elevated (155), and elevated susceptibility to IL-6 trans-signaling, which can confer abnormal suppressive function and disposition to Th17 conversion, may contribute to this (160).
In primary unexplained infertility, IL-1A, IFN-γ, and CCL11 are elevated in uterine fluid (161), and increased IL-12, IL-15, and IL-18 levels are linked with elevated uNK numbers in the decidua (80). Reduced endometrial expression of FOXP3 indicates fewer Tregs (19) potentially due to a systemic defect, as IVF success correlates with circulating levels of CD4+CD25+FOXP3+ cells (162, 163). Primate studies suggest that systemic and endometrial Treg deficiency is exacerbated by endometriosis, a common condition in infertile women (164). The association between reduced fertility and fewer Tregs in asthma (165), allergy (166), and autoimmune disease (167) supports the view of Treg dysfunction as an underlying cause (168).
In preeclampsia, Tregs in the maternal peripheral blood and decidual tissue are reduced (23, 36, 59), and suppressive function is impaired (169, 170), with an accompanying rise in proinflammatory Th17 cells (24), CD8+ effector cells, and trophoblast apoptosis (59). This is linked with pTreg deficiency (36), most notable in early-onset severe preeclampsia (59), as well as with CD45RA+CD31+ RTE tTregs being less able to acquire a memory phenotype (171). Dysfunctional DCs with reduced HLA-G and ILT4 expression (36) and/or insufficient expression of PD-L1 (172) may amplify Treg deficiency.
The clinical features of preeclampsia point to immune priming and memory, invoking a causal role for the adaptive immune response. Preeclampsia is more common in first pregnancies, especially when sexual contact with the conceiving partner has been limited because of a short duration of sexual cohabitation or barrier contraceptive use — and protection afforded by prior pregnancies is lost with a change of partner (65, 145, 173). Use of donor oocytes in assisted reproduction, where there is no prior contact with the donor’s alloantigens, leads to a striking 4.3-fold increase in preeclampsia compared with natural conception (174). With donor sperm, the risk is also increased, but, remarkably, this is mitigated by multiple exposures to the same donor’s semen (175).
Inadequate Treg priming and “shallow” placentation
Considering the extant evidence, it is reasonable to infer that insufficient Tregs in the periconception phase is a key upstream driver of the altered decidual environment and failure to achieve appropriate inflammatory resolution that precede limited invasion of trophoblasts and maternal vessel remodeling, in turn causing “shallow” placentation and ultimately preeclampsia and/or fetal growth impairment in later gestation (refs. 27, 70, 176, and Figure 3). This view fits the emerging paradigm invoking early pregnancy as the origin not just of miscarriage, but also of disorders of placentation linked with early-onset, severe preeclampsia, and as a contributing factor to fetal growth restriction, late spontaneous abortion, and preterm labor (27, 176, 177). Direct evidence for this comes from transcriptional analysis of chorionic villous samples, showing evidence of immune changes originating at implantation in women who later developed preeclampsia (178).
This raises the question of why some women have insufficient Treg numbers and function. Because newly generated pTregs are more vulnerable to phenotype switching and lineage instability (47), a secure Treg fate will depend on the conception environment. Treg priming may be dysregulated as a result of seminal fluid composition or responsiveness (112, 141). For example, when CD4+ cells from patients with recurrent miscarriage are cultured with DCs and the partner’s seminal fluid antigens, CD4+IL-17 and CD4+IFN-γ+ cells proliferate excessively, and fewer CD4+CD25+FOXP3+ Tregs are generated compared with fertile controls (158). The composition of seminal fluid immune-regulatory agents, particularly protolerogenic TGF-β, varies among and within men over time (179). The antitolerogenic cytokine IFN-γ, which drives the generation of Th1 immunity, fluctuates substantially in seminal fluid and can be elevated in response to infection (180). IFN-γ is elevated in the seminal fluid of male partners of women with recurrent miscarriage (181, 182). IFN-γ can interfere with the synthesis of GM-CSF required to drive T cell activation at conception (128, 183), skew Th0 differentiation toward Th17 cells (46, 184), and cause Tregs to transdifferentiate (185).
Bioavailability of cytokines, hormones, and microRNAs and the reproductive tract microbiome in the conception environment all potentially influence the Treg response (186–188). IL-10 deficiency elicits an unstable Treg response at implantation, with more rapid phenotype conversion and a reduced capacity to withstand an inflammatory challenge in later gestation (101, 189). Neutrophils also contribute, with neutrophil-depleted mice displaying insufficiency of proangiogenic, neutrophil-induced Tregs (116). Progesterone bioavailability impacts the Treg phenotype and secure fate commitment (190, 191), in part by inducing gal-1 to reinforce the tDC phenotype (100). Progesterone is commonly administered as luteal-phase support in IVF cycles (192), but whether this elevates Tregs at implantation remains to be determined.
Within hyperinflammatory environments, pTregs exhibit phenotypic plasticity and lineage instability, with a capacity to shift the phenotype and express cytokines that are characteristic of Teff lineages (47, 185). Tregs that undergo transdifferentiation into effector Th1 or Th17 cells, known as exTregs, drive pathology in inflammatory conditions and autoimmune disorders (193, 194). Epigenetic regulation of FOXP3 expression is a key determinant of whether T cells can maintain a suppressive phenotype (195, 196).
There is emerging evidence for Treg phenotype instability in reproductive disorders. Defects in stability would explain the observations of reduced Treg-suppressive competence (60) and evidence of elevated Th1 and Th17 cells in preeclampsia (24, 99). An intrinsic deficiency in peripheral blood Tregs in recurrent miscarriage is indicated by diminished IL-2 and TGF-β secretion as well as reduced IL-2/STAT5 signaling (197), while decidual Tregs have elevated IFN-γ expression (198). Exploratory studies implicate gene polymorphisms in the promoter region of FOXP3 in preeclampsia (199, 200). Tregs that express insufficient FOXP3 may be phenotypically plastic and express inflammatory cytokines, and exTregs could directly contribute to the pathology.
Conclusions and therapeutic prospects
Taken together, clinical studies and animal models support the inference that decidual Tregs are rate limiting for resolving inflammation at embryo implantation and establishing a decidual environment conducive to implantation receptivity and robust placental formation. It seems likely that in a substantial proportion of cases of infertility, recurrent miscarriage, and preeclampsia, Tregs are central and causal in disease development (21, 80). Immune imbalance or maladaptation causing incompetent phenotypes or insufficient Tregs provides a point of intersection channeling environmental, metabolic, and genetic factors (187) that likely interact with clinical factors such as prior pregnancy and male partner histocompatibility, which are identified as important in the prepregnancy antecedents of adverse pregnancy outcomes (22).
The biological properties of Tregs, particularly their responsiveness to environmental context and capacity to undergo phenotype switching (47, 187), may confer a maternal ability to distinguish and differentially invest in reproductive opportunities. The capacity of Tregs to transdifferentiate into Teffs in the event of infection, excessive inflammation, or disrupted fetal development (129) confers the capacity to terminate pregnancy and ensure maternal survival. The plasticity of Tregs may thereby provide an evolutionary benefit by contributing to maternal “quality control” that ensures optimal reproductive investment and maximizes offspring fitness (48). Vulnerability to reproductive disorders due to Treg instability (56, 57, 170) may be the biological trade-off (48).
The recognition that Tregs contribute to and may be causal in disorders of pregnancy raise the prospect of modulating the immune response to suppress the progression of symptoms and/or prevent their development. Interventions to boost Treg numbers and/or functional competence are in development and show promise for autoimmunity and tissue transplantation (194) and have been explored for cardiovascular disease as well (45). Given the rapid advances in Treg therapeutics, coupled with informative diagnostics based on flow cytometric analysis of peripheral blood, there is a strong prospect of targeting Tregs to tackle immune-based disorders in reproductive and obstetric medicine. Questions to first resolve include the antigen specificity of decidual Tregs, the key female and male partner determinants of optimal Treg generation and phenotype commitment, and the relevance of different Treg subsets including CD8+ Tregs.
Treg therapies relevant to pregnancy disorders could take one of three approaches: (a) advice on sexual activity, contraception, and lifestyle modifications to promote endogenous Treg generation; (b) in vivo interventions to increase Treg numbers and/or function in an antigen-nonspecific, tissue-targeted, or systemic manner; or (c) cell therapy methods that could include ex vivo generation and/or expansion of Treg numbers in vitro. Candidate biological agents to boost Treg numbers and stability using PF-L1 Fc (172) or CD28 superligand (122) already show a promising proof of concept in mouse models of preeclampsia. It will be important to focus on interventions and matching diagnostics that can be applied sufficiently early in pregnancy to have a reasonable chance of diverting the course of disease development. It is critical that any experimental evaluation of these approaches in a human reproductive setting take a highly cautious approach and be based on robust clinical trial design principles, as the risk-to-benefit ratio in reproductive and obstetric conditions is different than that for life-threatening immune diseases.
Acknowledgments
SAR has received funding from the National Health and Medical Research Council of Australia (NHMRC) (APP1099461). ASC has received funding from the NHMRC (APP1092191).
Footnotes
Conflict of interest: SAR is an inventor on international patent PCT/AU99/00499 and receives royalty income from Origio A/S. SAR and LMM receive research support from Ferring Pharmaceuticals (US).
Reference information: J Clin Invest. 2018;128(10):4224–4235.
https://doi.org/10.1172/JCI122182.
References
Articles from The Journal of Clinical Investigation are provided here courtesy of American Society for Clinical Investigation
Full text links
Read article at publisher's site: https://doi.org/10.1172/jci122182
Read article for free, from open access legal sources, via Unpaywall:
http://www.jci.org/articles/view/122182/files/pdf
Citations & impact
Impact metrics
Article citations
The role of viral infection in implantation failure: direct and indirect effects.
Reprod Biol Endocrinol, 22(1):142, 11 Nov 2024
Cited by: 0 articles | PMID: 39529140 | PMCID: PMC11552308
Review Free full text in Europe PMC
Development of a humanized mouse model with functional human materno-fetal interface immunity.
JCI Insight, 9(20):e176527, 22 Oct 2024
Cited by: 0 articles | PMID: 39435662 | PMCID: PMC11529984
B cells: roles in physiology and pathology of pregnancy.
Front Immunol, 15:1456171, 07 Oct 2024
Cited by: 0 articles | PMID: 39434884 | PMCID: PMC11491347
Review Free full text in Europe PMC
Pregnancy-related factors induce immune tolerance through regulation of sCD83 release.
Front Immunol, 15:1452879, 12 Sep 2024
Cited by: 0 articles | PMID: 39328416 | PMCID: PMC11424458
Metabolomics analysis of the effect of GnRH on the pregnancy rate of ewes with estrus synchronization scheme based on progesterone.
Front Vet Sci, 11:1442931, 11 Jul 2024
Cited by: 0 articles | PMID: 39055862 | PMCID: PMC11270128
Go to all (158) article citations
Similar Articles
To arrive at the top five similar articles we use a word-weighted algorithm to compare words from the Title and Abstract of each citation.
Reduction in Regulatory T Cells in Early Pregnancy Causes Uterine Artery Dysfunction in Mice.
Hypertension, 72(1):177-187, 21 May 2018
Cited by: 55 articles | PMID: 29785960
Seminal fluid and the generation of regulatory T cells for embryo implantation.
Am J Reprod Immunol, 69(4):315-330, 01 Apr 2013
Cited by: 93 articles | PMID: 23480148
Review
Immunological determinants of implantation success.
Int J Dev Biol, 58(2-4):205-217, 01 Jan 2014
Cited by: 64 articles | PMID: 25023687
Review
Seminal fluid and fertility in women.
Fertil Steril, 106(3):511-519, 30 Jul 2016
Cited by: 83 articles | PMID: 27485480
Review