Abstract
Free full text

Pathophysiology of Lacunar Stroke: History’s Mysteries and Modern Interpretations
Abstract
Since the term “lacune” was adopted in the 1800s to describe infarctions from cerebral small vessels, their underlying pathophysiological basis remained obscure until the 1960s when Charles Miller Fisher performed several autopsy studies of stroke patients. He observed that the vessels displayed segmental arteriolar disorganization that was associated with vessel enlargement, hemorrhage, and fibrinoid deposition. He coined the term “lipohyalinosis” to describe the microvascular mechanism that engenders small subcortical infarcts in the absence of a compelling embolic source. Since Fisher’s early descriptions of lipohyalinosis and lacunar stroke, there have been many advancements in the understanding of this pathological phenomenon. Herein, we review lipohyalinosis as it relates to modern concepts of cerebral small vessel disease. We discuss clinical classifications of lacunar stroke as well as radiologic definitions based on modern neuroimaging techniques. We provide a broad and comprehensive overview of lacunar stroke pathophysiology both at the vessel and parenchymal levels. We also comment on the role of biomarkers, the possibility of systemic disease processes, and advancements in the genetics of cerebral small vessel disease. Lastly, we assess preclinical models that can aid in studying lacunar stroke disease pathogenesis. Enhanced understanding of this highly prevalent disease will allow for the generation of specific molecular targets capable of mitigating disease sequalae.
Introduction and Historical Perspectives
Since the 1800s, lacunes were recognized as small infarcts involving the distal small penetrating branches of larger cerebral arteries. Their cavitated end-products originally seen on autopsy were referred to as “lacunes”, a French word derived from the Latin, lacuna, meaning cavity. Their cavitated end-products originally seen on autopsy were referred to as “lacunes”, a French word derived from the Latin, lacuna, meaning cavity. The first to characterize these lacunes was Amédée Dechambre, an intern at the Salpétriêre Hospital in Paris.(1) In 1838, he described the pathological findings of a male patient with hemiplegia and stated the following: “a number of small lacunes, of variable size and form, more or less filled with a milky fluid… resulting from liquefaction and partial reabsorption in the centre of the softening. According to the degree of hardening and reabsorption of the liquefied pulp these lacunes appear empty or stay more or less filled… It is, no doubt, with small foci of partial softening, that these round cavities without membranes should be correlated”.(2,3) Five years later, another French neurologist Maxime Durand-Fardel independently arrived at similar findings.(4) In his treatise, he noted that: “the striatum… showed a certain number of small lacunes with no associated alteration of colour or consistency from whose surface there extended a fine meshwork containing very small vessels”.(5) In later works, he used the term état criblé to describe enlarged spaces in the white matter, which were in a cribriform pattern.(3,6)
It was not until 1901, when Pierre Marie correctly made a distinction between état lacunaire and état criblé.(6,7) Using the collective term état lacunaire to describe numerous basal ganglia infarctions, he noted that these lesions were responsible for sudden motor deficits and the characteristic gait, marche à petits pas de Déjérine. He astutely observed that the underlying lacunes were created by a “local arteriosclerotic process”(8) as well as “destructive vaginalitis”, in which perivascular spaces demolished adjacent areas of brain tissues.(6) These disease paradigms persisted until the mid-20th century when Charles Miller Fisher performed numerous pathological studies of lacunes.(9) He used the term, lipohyalinosis, to describe a unique mechanism that gave rise to such cerebral small vessel disease (cSVD)-related infarcts.(10,11)
Today, lacunar strokes (LS) comprise up to 20-30% of all acute ischemic strokes.(12,13) Although these infarcts are smaller in size compared to those caused by large vessel occlusions, they were found to be the most common type of acute strokes (37%) to progress after onset in the Harvard Stroke Registry.(14–16) The term “stuttering” has been used to describe this phenomenon. Stuttering LS are particularly challenging for the clinician as there are limited interventions he or she can implement as patients deteriorate. Furthermore, LS are burdensome given their recurrence rate of up to 20% over 3 years and their resultant functional impairment and disability.(17) Because of their association with cardiovascular disease, one-fourth of patients who experience these strokes will die after five years.(18) Moreover, there is an increased incidence of LS in younger patients.(19) Estimates suggest that annually 11 million people suffer from silent strokes in the US alone, the majority of which are due to cSVD,(20) showing that the pathology is highly prevalent even without overt symptoms. These asymptomatic infarcts contribute to a large proportion of neurological morbidities including vascular cognitive impairment,(21) which has been increasing in prevalence.(18) The care of stroke patients is complex(22) and while there have been several revolutionary advances in the treatment of large vessel occlusions,(23–28) little progress has been made in the targeted treatment of LS.(29) However, continued advancements in understanding LS pathogenesis may make targeted cSVD therapeutics a realistic goal.
Clinical and Radiographic Classification Criteria
Clinical LS in the classic sense are caused by infarcts between 5 mm to 15 mm in greatest diameter and involve the deep gray and subcortical white matter (Figure 1). Infarcts above that size in diameter (15 to 20 mm) may be due to involvement of several perforating vessels in the putamino-capsulo-caudate region, and have been referred to by some as “giant” lacunes,(30) “lagunes” (a portmanteau for large lacune), and “macunes” (for major lacunes).(31,32) Not surprisingly, these large LS are more likely to have severe symptoms and disability and may reflect more heterogenous underlying etiologies including cardioembolism(32) or atherosclerotic disease in a major penetrator artery at its origin.(33)
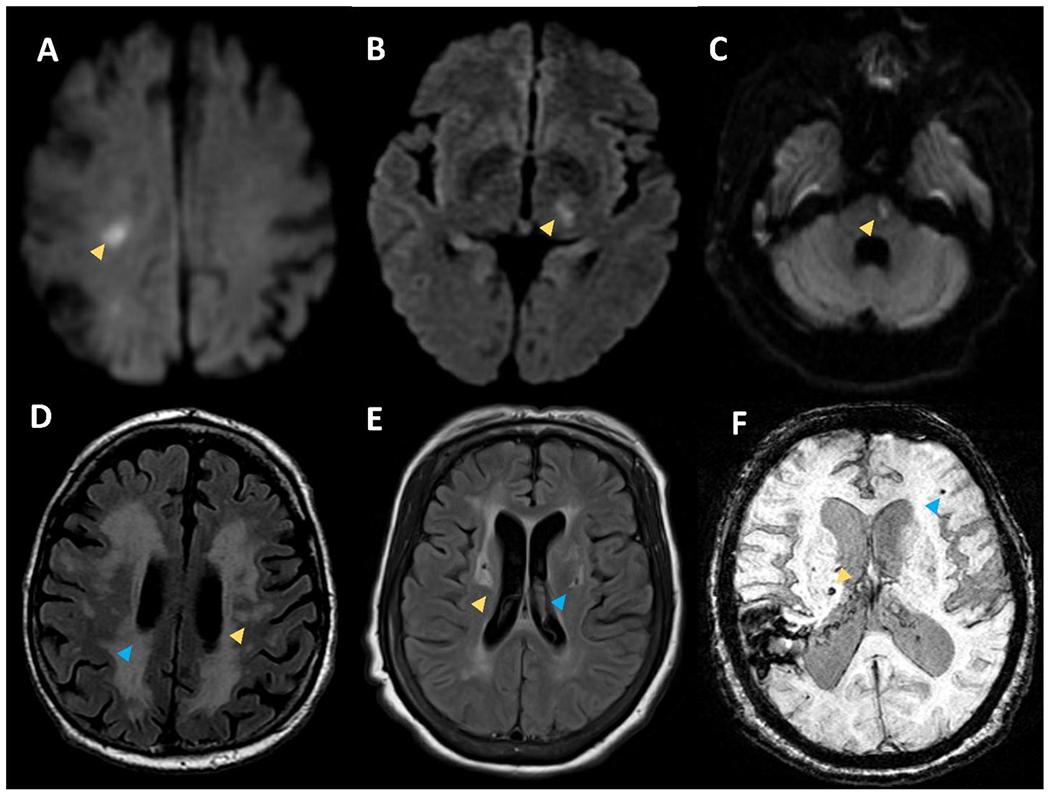
Small infarcts on DWI sequences within the right subcortical white matter (A), left thalamus (B), and left pons (C) consistent with acute lacunar strokes. Panel D shows T2 FLAIR sequence with white matter hyperintensities in the subcortical white matte (yellow arrow) and periventricular white matter (blue arrow). Panel E shows T2 FLAIR sequence with white matter hyperintensities on in the basal ganglia (yellow arrow) and deep chronic lacune with a rim of hyperintensity (blue arrow). Panel F shows susceptibility weighted imaging with superficial microbleed (blue arrow) and deep microbleed (yellow arrow).
There are many classification schemes for LS (Table 1), but perhaps the most widely accepted classification of stroke subtypes was described in the Trial of Org 10172 in Acute Stroke Treatment (TOAST) conducted in 1993.(34) In this stroke classification schema, the third subtype of stroke was typified by cSVD. Per TOAST classification, cSVD-related infarcts are generally accompanied by traditional clinical LS syndromes; Fisher described 21 different syndromes.(11) A hallmark clinical feature of these syndromes is the absence of cortical signs or symptoms such as aphasia, apraxia, agnosia, visual field cut, memory impairment, stupor, coma, loss of consciousness, or seizures. Perhaps the five most notable syndromes are pure motor strokes, pure sensory stroke, ataxia-hemiparesis, dysarthria-clumsy hand, and sensorimotor strokes. In addition to a LS syndrome, neuroimaging with computed tomography (CT) or magnetic resonance imaging (MRI) should be normal or reveal a relevant brain stem or subcortical lesion with a diameter of less than 15 mm. Potential cardiac sources for embolism should be exonerated, and vessel imaging should not demonstrate a stenosis of greater than 50% in an ipsilateral proximal artery. While these criteria have been adopted by many practitioners, some have argued that their strict interpretation under-represents the total cases of LS.(35)
Table 1.
Classification schemes for lacunar stroke and cerebral small vessel disease.
LS Classification | Assessment | Definition | Reference |
---|---|---|---|
TOAST | Clinical | CT/MRI unremarkable or with subcortical lesion <15mm that explains acute symptoms, No cardioembolism, No ipsilateral large vessel stenosis >50% | (34) |
CCS | Clinical | CT/MRI with lesion in territory of penetrating vessel and <20mm that explains acute symptoms, No focal pathology in parent artery | (36) |
ASCOD | Clinical | CT/MRI with small/deep lesion <15mm that explains acute symptoms, Stuttering symptoms, CT/MRI evidence of chronic LS/WMH/CMB/PVS | (37) |
STRIVE | Radiographic | Recent small subcortical infarcts are DWI hyperintensities <20mm, Chronic lacunes are T2 hypointensities with hyperintense rim <15mm | (38) |
Poirier and Derouesné | Pathologic | Type Ia old infarcts <20mm with pan-necrotic cavitation and scattered macrophages, Type Ib incomplete infarcts with selective loss of vulnerable elements | (92) |
cSVD Disease Process | Vessel Size | Definition | Reference |
Arteriosclerosis/atherosclerosis | 200-800 μm | Microatheromatous plaques with foam cells | (11) |
Arteriolosclerosis | 40-150 μm | Concentric hyaline thickening of vessel wall | (59) |
Lipohyalinosis | 40-300 μm | Non-inflammatory lipid and protein aggregation | (60) |
The Causative Classification of Stroke (CCS) was the first computerized, evidence-based algorithm that provided both causative and phenotypic stroke subtypes in a rule-based manner. Through answering a series of questions, the algorithm displays the most likely diagnosis. It was shown to have a high inter-rater reliability and be useful for international multicenter studies.(36) Within the algorithm, a LS is defined as being located in the territory of a penetrating artery and should be less than 20 mm in its greatest diameter. There should be no focal pathology in the parent artery at the site of the origin of the penetrating artery.
Another recent classification, the ASCO (A: atherosclerosis; S: small-vessel disease; C: cardiac pathology; O: other causes) criteria, and the subsequent ASCOD(37) (including dissection) also incorporated cSVD as a stroke subtype. This classification schema incorporated disease causality into the stroke classification using five categories: 1) potentially causal, 2) causal link uncertain, 3) causal link likely but the disease is not present, 4) small-vessel disease is not detected, and 5) incomplete workup. Small vessel strokes are deemed potentially causal if the patient has a small, deep infarct less than 15 mm in diameter on neuroimaging in the territory corresponding to symptoms. Additional criteria that need to be met include the presence of one of the following: 1) several old or silent lacunes or small subcortical strokes in other territories, 2) leukoaraiosis (white matter hyperintensities, WMH), cerebral microbleeds (CMB), or dilated perivascular spaces, or 3) recurrent transient ischemic attacks that are attributable to the same territory as the LS. The causality is classified as uncertain in cases without accompanying neuroimaging evidence of stroke but with a clinical LS syndrome.
Because of recent advances in the understanding of cSVD, the STandards for ReportIng Vascular changes on nEuroimaging (STRIVE) consortium developed additional criteria that more accurately reflects neuroimaging findings of cSVD(Figure 2).(38) These criteria separate the imaging findings of LS into recent small subcortical infarcts and lacunes of presumed vascular origin. The term recent small subcortical infarcts (which can be up to 20 mm in maximum diameter) describes infarcts that are seen on diffusion-weighted imaging (DWI) sequences in the acute setting. The chronic products of such infarctions include lacunes of presumed vascular origin and white matter hyperintensities.(29,39) Chronic lacunes of presumed vascular origin are typically subcortical fluid-filled spaces (approximately 3 to 15 mm in diameter) and are best seen on T2-weighted MRI sequences. Furthermore, WMH have a diverse and heterogenous etiology of their own, in addition to resulting from cSVD.(40,41) Although not formally adopted in STRIVE definitions, smaller lesions have been termed cerebral microinfarcts (CMI), which are 0.2 mm to 3 mm in size(42). These lesions are also likely related to cSVD (discussed below).
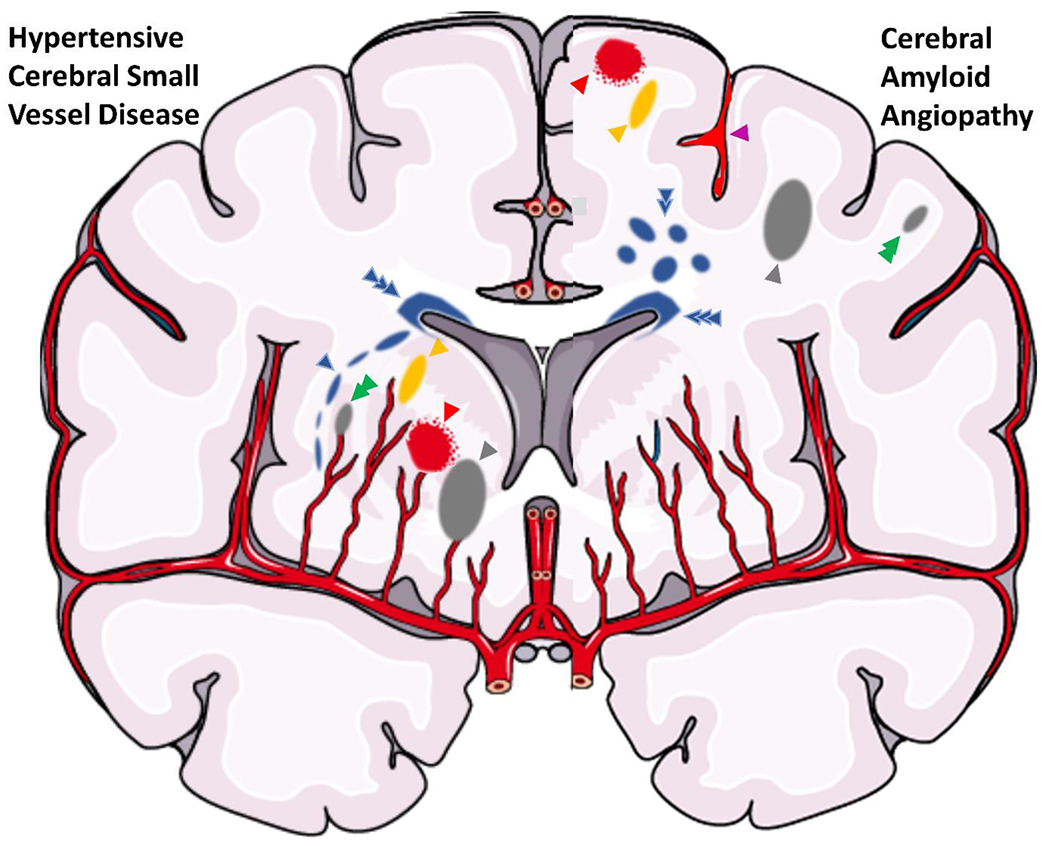
Illustration of a coronal brain section with parenchymal findings of cerebral small vessel disease. The right hemisphere includes findings suggestive of hypertension-related small vessel disease, including a peri-basal ganglia white matter hyperintensity (blue arrow), dilated perivascular space (yellow arrow), microinfarct (green arrow), deep lacune (gray arrow), and microbleed (red arrow). The left hemisphere includes findings suggestive of cerebral amyloid angiopathy, including a microbleed (red arrow), sulcal siderosis (purple arrow), dilated perivascular space (yellow arrow), subcortical white matter hyperintensity spots (double blue arrow), lobar lacune (gray arrow), and microinfarct (green arrow). There is also perivascular white matter hyperintensity (triple blue arrow), which is associated with both small vessel diseases.
For this review, we focus on LS either determined in the acute setting (recent small subcortical infarct) or the chronic cavitated end-product, acknowledging that recent subcortical infarcts can also lead to WMH. For the vessel pathology, we focus on sporadic cSVD (sometimes referred to as hypertension-related) as opposed to cerebral amyloid angiopathy (CAA)-cSVD.(43) We also acknowledge that while LS may appear “lacunar” clinically and radiographically, some result from etiologies other than diseased small vessels.(44–46) These non-cSVD etiologies may account for around 10% of imaging and clinically-defined LS.(47) These include branch atheromatous disease involving large vessel disease that obstructs a perforator,(45) artery-to-artery embolism,(48) which can arise from the carotid(49) or aortic arch,(50) and cardioembolism.(47) While the aforementioned studies show LS may be associated with non-cSVD etiologies, it is important to recognize that cSVD shares risk factors with these other etiologies, and it can be difficult to rule out concomitant processes..(51) Indeed, another study of a cohort of ICH patients showed that there was no association between intracranial atherosclerosis (vessel calcification and stenoses) and markers of cSVD (WMH and CMB), supporting atherosclerosis and cSVD are distinct unrelated processes.(52) Most LS result from intrinsic disease of the small perforating arteries. Furthermore, to conceptualize LS and devise novel therapeutic targets, it is important to distinguish the vessel pathology from the parenchymal pathology, although they are intimately related (Figure 3).
Vessel Histopathology
In Fisher’s early studies of serial sections through over 68 lacunes in 18 human cadaveric brains, he noted that most were found distal to occlusive lesions of small perforating arteries.(9,10,53–57) These occlusive lesions are created by distinct vessel pathologies that are based on the size of the vessel and include: arteriosclerosis/atherosclerosis, arteriolosclerosis, and lipohyalinosis.(58,59) Arteriosclerosis/atherosclerosis affects vessels of 200 to 800 μm and involves similar mechanisms as atherosclerosis of larger vessels (although calcifications are less prominent). In Fisher’s descriptions, atherosclerotic plaques with foam cells were described as microatheromas in the proximal perforating arteries, junctional atheromas in the origin of a perforator, or mural atheromas.(11) Arteriolosclerosis affects vessels 40 to 150 μm and causes concentric hyaline thickening of the vessel wall, as seen in other organs such as the kidney.(59) The third mechanism of LS is a process that Fisher termed lipohyalinosis. This process affects vessels with a size ranging from 40 to 300 μm and is characterized by the aggregation of lipid and proteins.(60) This histopathological term is thought to be the major driver behind cSVD, and underlies most LS.
Because of his pathological observations, Fisher defined lipohyalinosis as a “hypertensive cerebral vasculopathy in which the lumen of an artery, usually less than 200 μm in diameter, is occluded, the wall of the artery is … reduced to connective tissue shreds, hemosiderin-filled macrophages lie scattered in the vicinity, and the wall stains bright red with oil-red-O”.(11) The central feature of this pathology is non-inflammatory fibrinoid vessel wall necrosis. Connective tissue largely replaces the affected vessel walls to variable degrees, extending to the central region of most vessels and occluding the lumen. Muscular elements and elastic elements were often unrecognizable. Few fatty macrophages were observed between lamellae of the disintegrating vessel wall. The vessels did not contain polymorphonuclear leukocytes or pleomorphic histiocytes. Fibroblast proliferation was noted in the adventitia. Interestingly, this disorganization was a focal process, usually extending 2-3 times the vessel diameter. This “segmental arterial disorganization” was often found at sites of bifurcation and branching.(10)
Segmental arteriolar disorganization is associated with three prominent, but often overlapping findings: vessel enlargement, hemorrhage, and fibrinoid deposition.(10) In most of the lesions, enlargement was thought to be primarily from connective tissue external to the vessel lumen, increasing the diameter two- to three-fold. Fisher argued that there was no clear evidence of lumen dilation; rather the fibrosis and disorganization obscured the boundary between wall and lumen, increasing the diameter due to wall thickening. The form of the enlargements varied, but most were asymmetric. In some cases, single arteries were identified with multiple focal areas of disorganization/enlargement of different ages. Hemorrhage was also present in some lesions via a destruction of vessel walls.(10) Some acute lesions had red blood cells within the damaged wall itself, suggesting a possible local microdissection. Chronic lesions were associated with hemosiderin laden macrophages, suggesting prior hemorrhage. Astroglial proliferation was observed around the hemorrhage. It was also noted that hemorrhage could be found in lesions that did not appear to have enlargement, arguing against a microaneurysm rupture hypothesis. Many lesions also demonstrated evidence of fibrinoid deposition.(10) This deposit could be found in acute or chronic lesions. Furthermore, the fibrinoid deposition within the vessel appeared to cause both lumen reduction and outward wall bulging.
In similar fashion to larger vessel occlusions, connective tissue was often found proximal and distal the lesion, indicating thrombosis and subsequent organization. Retrograde occlusion was noted within pontine penetrators in particular, where normal restoration of flow occurred at the junction of the parent artery. The organized connective tissue varied from fine threads to dense collagenous strands. There were infrequently noted small “channels of recanalization.” In areas of arteries affected by secondary thrombosis, walls were thinned, poorly stained, and atrophic, resembling veins.(10) The progression of this pathology has been described to affect the brain in 3 stages. In the first stage, lipohyalinosis preferentially affects the basal ganglia. In the second stage, lipohyalinosis involves the deep white matter, and also the cerebellum, thalamus, and leptomeningeal arteries. In the last stage, lipohyalinosis affects the brainstem.(11)
Vessel Pathophysiology – Initiation and Progression
Much of Fisher’s work aimed to elucidate the pathophysiology of lipohyalinosis and segmental arterial disorganization, which we refer to as cSVD. At the time of his detailed description, the process of small vessel destruction had been given several other names including fibrinoid necrosis,(61) plasmatic destruction,(62) hyalinosis,(63) angionecrosis,(64) and fibrinoid arteritis.(65) Regardless of name, the mechanism of cSVD is incompletely understood mostly because of the inherent limitations of human autopsy studies. Most autopsy studies of diseased vessels are of old, healed lesions given the low mortality rate of acute LS.(66) With only histologic descriptions, one must be cautious when making inferences about mechanism. Novel imaging techniques are enhancing our understanding of the disease, but do not allow for direct visualization of the human small vessels in vivo. It is now believed that the vessel pathophysiology underlying cSVD likely involves two main pathogenic mechanisms: endothelial dysfunction and blood-brain-barrier (BBB) disruption.(45) In cSVD, there is a shift in the balance of basic functions of both the endothelium and the BBB.
The endothelium is important for the regulation of vessel tone, fibrinolysis/coagulation, inflammation, and angiogenesis. Endothelial dysfunction can be best understood in terms of a shift in each of these functions towards: vasoconstriction with impaired autoregulation, procoagulation, proinflammation, and proliferation.(67) There are different theories regarding how this shift occurs. It was initially hypothesized that hypertension (HTN) was the most important factor, where abnormal mechanical forces on the vessel were secondary to elevated pressure. However, the prevalence of cSVD remains high despite today’s effective and widely used antihypertensive agents. As the disease progresses, there is a failure of autoregulation, and the vessel is unable to dilate to maintain perfusion distally.(68) “Forced dilatation” occurs, as connective tissue accumulates in the vessel wall which leaves downstream vessels subject to even higher pressures and mechanical damage.(69–71) The thickening worsens and eventual narrowing of the lumen occurs with thrombosis and occlusion.(45) Indeed, experimental animal models of HTN can induce fibrinoid necrosis.(72) As the necrosis matures, there is subsequent worsening disorganization and occlusion of the arterial lumen.
Other theories of endothelial dysfunction are proposed, some less widely accepted.(60) Rather than direct effects of HTN on mechanical vessel wall destruction, there may excessive early autoregulatory vasoconstriction that causes focal ischemia. Interestingly, one model of experimental HTN showed that fibrinoid necrosis occurred in only localized segments that demonstrated areas of alternating vasoconstriction and dilation.(71,73) Repeated administration of the vasoconstrictor vasopressin in a rat model demonstrated that it is possible to induce fibrinoid necrosis.(74) More recent studies have focused on other targets. Thrombomodulin, a marker of endothelial dysfunction, may also be involved. A study of cSVD brains compared to aged controls, showed that it was elevated in cSVD brains.(75) Vascular endothelial growth factor has also been considered for a potential role, though it failed to correlate with disease severity in one study.(76) Yet another autopsy study of cSVD brains showed that white matter penetrating arterioles had impaired vasodilation compared to those in the pia.(77)
The BBB is also important in the vessel pathology of LS. The BBB is comprised of several inter-related structures: tight junctions joining endothelial cells, basement membranes, associated perivascular spaces, pericytes, glia limitans, and astrocyte end feet.(68) High arterial pressure has been proposed to degrade the BBB. This results in local edema and plasma protein deposition in the vessel wall, which deteriorates the wall structure and damages smooth muscle cells.(78) However, as with endothelial dysfunction, it is becoming clear that other factors besides HTN are important. BBB degradation likely also underlies the development of more slowly progressing WMH, which are intimately related to LS. Edema develops surrounding leaky vessels which results in gliosis and appears as WMH radiographically.(45) In patients with LS, poor functional outcome was associated with increased basal ganglia BBB permeability as measured by contrast-enhanced MRI 3 years later.(79) Additional studies showed that BBB leakage may mediate cSVD, and HTN was associated with the severity of leakage.(80) In patients with recent LS, global cSVD burden was associated with increased BBB degradation, interestingly at both the acute infarct site and non-ischemic areas.(81)
To understand what shifts the balance of these basic functions of the endothelium and BBB, leading to endothelial dysfunction and BBB degradation, respectively, it is useful to think in terms of initiation and progression of the disease. What causes the disease process in the first place and why does it get worse over time? A complex interplay of several factors, including HTN, diabetes mellitus (DM), aging, oxidative stress, mechanical stress, and genetic predisposition are likely involved. Epidemiological studies and genetics studies offer insight (discussed below). There have been several recent advancements in our understanding of the risk factors for cSVD and LS.(43) HTN has been a long known risk factor(45) for fibrinoid necrosis in the small vessels of the brain and other organs.(82) Interestingly, the vessels of the brain appear to be particularly susceptible as they undergo fibrinoid necrosis secondary to milder elevations in blood pressure.(65,83,84) Different organs have been shown to have varying degrees of susceptibility to HTN induced damage in an animal model of inducible HTN.(85) However, it has been proposed that HTN may be an epiphenomenon in the development of cSVD. Using a transgenic rodent model expressing prorenin solely in the liver, it was shown that “hypertensive” end-organ damage, including fibrinoid necrosis, could be seen in normotensive rats.(86) This finding raised the question that HTN may not be required to induce vessel pathology, but it may be secondary to local generation of an excess of vasoconstrictors, such as angiotensin II. This peptide is not only a potent vasoconstrictor but also plays a role in vascular remodeling.(87)
DM is another well-known risk factor for LS.(45) Using data from the Atherosclerosis Risk in Communities study, it was shown that smaller lacunes <7mm were associated with DM and HbA1C levels, while larger ones were associated with low density lipoprotein.(88) This supports DM plays more of a role in cSVD compared to atherosclerosis and other diseases of the smaller cerebral vessels. Another study using Framingham Heart data showed that incident LS was associated with HTN and DM, but incident intracerebral hemorrhage was associated only with HTN.(89) Perhaps DM is more important for the small vessel pathology that causes LS. A genetic predisposition to longevity may also be protective for cSVD and LS. Patients from the Leiden Longevity Study (offspring of nonagenarians) had lower susceptibility to WMH and lacunes, but not CMB.(90) Similarly, stroke in the young appears to be associated with the development of a higher burden of cSVD in mid-life, where these patients had accelerated cerebral aging by 10-20 years.(91)
Parenchymal Histopathology
In addition to the vessel pathology, there is also a unique parenchymal pathology. It is important to make this distinction to understand the pathophysiology of LS and identify novel therapeutic targets, but the vessel pathology and parenchymal pathology are intimately related. In regard to the brain parenchyma, Fisher examined lacunes of varying ages and observed that more chronic lacunes had trabeculated cavities that were surrounded by gliosis and few macrophages, while recent lacunes contained numerous macrophages and had minimal gliosis. Due to the wide-ranging appearance of these lesions, Jacques Poirier and Christian Derouesné proposed a classification schema for these lesions in 1984.(92) This classification system included three types of lacunes: Type I are ischemic lesions, Type II are microbleeds where the lacunar cavity is filled with hemosiderin-laden macrophages, and Type III are dilated perivascular spaces. Recent advancements in neuroimaging (described above), have since allowed the radiographic correlates to be easily distinguished. On T2-weighted MRI, lacunes have a surrounding hyperintense rim compared to dilated perivascular spaces, which lack this finding and are typically smaller than lacunes (<3mm).(38,93,94)
The pathologic description of the subtypes of Type I ischemic lesions, Ia and Ib, provide additional insight into cSVD pathogenesis. Type I lesions are characterized by an irregular cavity ranging from 1 to 20 mm in length, and are most commonly found in the putamen, caudate, thalamus, pons, internal capsule, and hemispheric white matter.(9,95) Within this group, Type Ia lesions are old infarcts with pan-necrotic cavitation and scattered macrophages. Type Ib lesions represents incomplete infarcts with selective loss of only some vulnerable elements.(96) Interestingly, in a study by Lammie et al. examining 172 autopsy brains, Type Ib incomplete infarcts were seen in the majority of cadaveric brains that contained the classic Type Ia lesions.(96) These lesions exist on a spectrum, and can be graded from 1-3(96), based on their size, parenchymal integrity, surviving neurons, surviving glia, presence of reactive glia, and presence of inflammatory cells. Grade 1 lesions are about 0.5x0.5 mm, have parenchymal rarefaction/pallor, a mild reduction in neuron number with occasional “ghost” neurons, preserved oligodendroglia, absent reactive astrocytes, and absent inflammatory cells (lymphocytes, macrophages, siderophages). Grade 3 lesions, on the other hand, are about 10x1 mm, and exhibit spongiosis, rarefaction with small central cavitation, no surviving neurons with only occasional surviving oligodendroglia, gliovascular septa in cavities with surrounding gliosis, and inflammatory cells scattered in and around the cavity. Grade 2 lesions are described as an intermediary of the two.
The etiology of Type Ib parenchymal lesions has been debated; understanding the cause of these lesions has implications for therapeutic targets. It has been contended by some that Type Ib are secondary to local edema instead of ischemia.(97) Ma et al. first described “edema-associated gliosis” (97) that appears similar in morphology to these lesions.(98) Furthermore, similar findings have been recapitulated in animal models.(99–101) As mentioned above, this pathology was originally described as resulting from destructive vaginalitis in which the blood vessel remained patent, but there was perivascular space dilatation which destroyed surrounding parenchyma (similar to Type III lesions). Still, most speculate that Type Ib lesions result from ischemia, similar to Type Ia lesions.(102) Most likely, these incomplete Type Ib lesions differ from Type 1a lesions secondary to a less severe degree of ischemia, a shorter duration of ischemia, and less likely a different etiology of ischemia.
Parenchymal Pathophysiology – Ischemia, Infarction, and Repair
Three principles underlie the dynamic processes of injury and recovery in LS: ischemia, infarction, and repair. Ischemia occurs when parenchyma is deprived of blood affecting its function but not causing apoptosis and necrosis. Infarct refers to parenchyma that has undergone apoptosis and necrosis. These likely exist on a continuum where blood flow changes can tip the balance. While different from large vessel occlusions,(103) there is also mounting evidence supporting a role of decreased blood flow in lacunes, small subcortical infarcts, and WMH. A recent study showed WMH had decreased cerebral blood flow (CBF) and decreased vascular reactivity compared to contralateral normal white matter.(104) Another study demonstrated that at 4-year follow-up, baseline WMH volume was associated with decreased CBF over time, but baseline CBF was not associated with progression of lacunes or WMH.(105) In contrast, another study of minor stokes showed that at 18 month follow-up, regions of low baseline CBF were associated with the development of new WMH.(106) Many hypothesize that lower CBF precedes the development of LS and WMH, but the data are conflicting.(107) Interestingly, the peri-infarct region was recently pathologically examined in lacunes,(108) and axons up to 150% of the infarct diameter away showed changes reflecting a loss of normal cell-cell adhesion and signaling between axons and oligodendrocytes. This may reflect injury to myelin out of proportion to axons, as opposed to decreased CBF. These findings have been replicated in another study demonstrating enlargement/progression of lacunes by serial imaging.(109) This slow stroke progression may prove a viable target for therapeutic intervention in many human patients.
Repair refers to several processes involving proliferating surviving elements and neuroplasticity, including neurogenesis, gliogenesis, axonal sprouting, remyelination, and altered neuronal excitability.(110) Importantly, the repair phase occurs after the initial ischemia and infarct phases. There is a biphasic nature to this progression, emphasizing the importance of when, where, and how damaged brain makes the transition from injury into repair. (111) This is exemplified at the structural level by matrix metalloproteinases, which have a deleterious role acutely but are essential for neurovascular remodeling during subsequent repair,(112) and at the functional level by changes in excitability, in which excitotoxicity leads to further injury acutely but increasing excitability promotes recovery in the repair phase.(113,114) This concept may also be pertinent for LS and injury isolated to white matter, although the structural and functional elements are different and only beginning to be understood. There are likely spontaneous processes that stabilize the BBB, modulate glial scarring, regulate remyelination, slow axonal degeneration, and promote the health of the oligovascular unit.(108,115,116) These endogenous mechanisms involve oligodendrocyte precursor cells (OPCs),(117) which proliferate, migrate, and differentiate into myelinating oligodendrocytes.(118,119) There may also be endogenous processes that can hinder repair. Glial scar formation involves several cell surface and extracellular matrix proteins that can inhibit axonal growth and impair the ability of oligodendrocytes to myelinate new axons. These detrimental proteins include neurite outgrowth inhibitor (Nogo), ephrin ligands, and chondroitin proteoglycans.(120) A recent mouse study showed Nogo receptor 1 blockade could overcome remyelination failure and stimulate functional recovery after LS.(121). Further demonstrating the complexity and biphasic nature of repair, reactive astrogliosis can be beneficial both acutely and under certain circumstances during repair.(122,123)
As expected, these parenchymal processes are difficult to study in vivo, especially in human patients. However, serial imaging studies of small subcortical strokes, lacunes, WMH, and other imaging biomarkers of cSVD may help us understand the progression of disease. These imaging sequelae are inter-related and show changes consistent with both progression and regression of severity.(124) Indeed, some small subcortical infarcts can become undetectable(41) and some WMH can regress on repeat imaging.(125) This may occur through alteration in blood flow, focal small vessel changes, and parenchymal repair processes. Interactions between small subcortical infarcts, lacunes, and WMH have been studied, and a WMH “penumbra” hypothesis has been proposed as incident lacunes have a predilection for the edge of WMH.(126) However, another study confirmed their main axes aligned with the orientation of perforating arteries and not white fiber tracts,(127) supporting the widely accepted vascular etiology. Perhaps flow-limiting areas of vessel disease affecting lower-order branched vessels contribute to downstream hypoperfusion and WMH. When subsequent branches develop worsening pathology and/or when perfusion decreases further in these already vulnerable areas, small subcortical infarcts occur and WMHs progress.(29)
In addition to relationships to each other, lacunes, small subcortical infarcts, and WMHs are also related to CMI. While smaller than small subcortical infarcts and lacunes, CMI may be a linked to LS in their underlying etiology. CMI have been classically observed in neuropathological studies(128) and have been more recently observed by 3T and 7T MRI scanners.(42,129–132) In a cadaveric study, in vivo 1.5 T MRI was compared to post mortem ex vivo 7T MRI and histology. The authors noted that the vast majority of CMI are under the detection limits of clinical in vivo MRI.(133) In another study, silent DWI positive CMI were detected in 3.8% of cognitively impaired, 5% of normal, and 12.9% of past ICH patients.(134) Histologic sampling showed that even a single DWI lesion suggested an annual incidence of hundreds of new CMI.(134) CMI are becoming more clinically relevant as they become better understood. Asymptomatic DWI positive CMI have been shown to produce chronic local microstructural injury;(135) they are a common finding in memory populations and are associated with vascular dementia.(130,131,136)
CMI likely have a heterogenous etiology but many result from cSVD of high order branched vessels. Indeed, the location of CMI has shown some predictive value. While deep CMI are likely secondary to cSVD,(42,137) cortical CMI are more heterogeneous in etiology as they are related to CAA,(42,137) cSVD and LS,(132) and intracranial stenosis and large cortical infarcts.(132) A study of patients with recent ICH showed that the lobar location was associated with CMI in the frontal lobe, while the deep location was associated with CMI in the parietal lobe.(138) In another study of patients with ischemic stroke, CMI were found in 28%, 71% of which were in the vascular territory of the primary infarct. WMH was associated with CMI outside the territory and large vessel atherosclerosis was associated with CMI within it.(139) Understanding the role of CMI may provide further insight into LS pathogenesis.
These imaging findings in cSVD have also been found to be associated with other imaging biomarkers, including ICH. One study showed that lobar lacunes, defined as lacunes by the STRIVE definition that are located in cerebral lobes, were associated with CAA-related ICH, while deep lacunes were associated with HTN-related ICH. Furthermore, lobar lacunes had an association with WMH.(46) This finding was confirmed in another study, which also demonstrated an association of lobar lacunes with brain amyloid deposition on positron emission tomography imaging.(140) These associations prompt investigation into the role of CAA in lacune formation. While lacunes are more typically found in deep brain structures, they have been reported in the subcortical white matter such as the centrum semiovale, even by Fisher.(9,10)However, we acknowledge that lacunes from infarction are sometimes difficult to distinguish from dilated perivascular spaces, which are also associated with CAA.(141) Another study demonstrated that subcortical WMH were more common in CAA, while peri-basal ganglia WMH were more common in cSVD. Predictors of peri-basal ganglia WMH included deep CMB, total WMH volume, high BG perivascular spaces.(142) Indeed, WMH are related to both cSVD and CAA. In patients with CAA, WMH increase over time, and are associated with incident lobar ICH. This supports that WMH in CAA is related to progressive vessel disease.(143) Yet another study showed that WMH have a predilection for areas of lower perfusion, regardless of underlying diagnosis.(144) Perivascular spaces may also provide insight into the underlying microangiopathy. In a study of patients with ICH, centrum semiovale perivascular spaces were associated with lobar CMB and cortical superficial siderosis, while basal ganglia perivascular spaces were associated with deep ICH and WMH volumes.(145) A similar finding was shown in patients with cognitive impairment, where white matter perivascular spaces were associated with lobar CMB, and basal ganglia perivascular spaces were associated with HTN.(146)
Biomarkers in Lacunar Stroke and Systemic Disease
The development of biomarkers for LS and cSVD is important for predicting disease severity and prognosis and for preventing disease progression (Table 2). Biomarkers can also shed light on mechanisms and serve as outcomes for future studies. A meta-analysis showed that markers of coagulation/fibrinolysis, endothelial dysfunction, and inflammation were altered in LS compared to other stroke subtypes and/or healthy controls. This suggests that these three pathways are clinically relevant mechanisms to LS pathogenesis.(147)
Table 2.
Biomarkers in lacunar stroke and cerebral small vessel disease.
Biomarker | Association | Comparison | Reference |
---|---|---|---|
Coagulation/fibrinolysis | |||
tissue plasminogen activator | ↑ | LS vs patients without stroke | (147) |
tissue plasminogen activator | ↓ | LS vs cortical stroke | (148) |
fibrinogen alpha chain | ↑ | LS vs patients without stroke | (158) |
fibrinogen beta chain | ↑ | LS vs patients without stroke | (158) |
fibrinogen | ↓ | LS vs cortical stroke | (147) |
fibrinogen | ↑ | LS with adverse outcome vs LS without | (158) |
D-dimer | ↑ | LS vs patients without stroke | (147) |
D-dimer | ↓ | LS vs cortical stroke | (147) |
thrombin-antithrombin complex | ↑ | Blacks with LS vs those without | (153) |
Endothelial dysfunction | |||
von Willebrand factor | ↑ | LS vs patients without stroke | (149) |
von Willebrand factor | ↓ | LS vs cortical stroke | (149) |
homocysteine | ↑ | LS vs patients without stroke | (149) |
homocysteine | None | LS vs cortical stroke | (149) |
endothelial progenitor cells | ↓ | LS and WMH vs hypertensive controls | (150) |
angiogenic T-cells | ↓ | LS and WMH vs hypertensive controls | (150) |
Platelet activation | |||
activated glycoprotein IIb/IIIa | None | LS vs patients without stroke | (149) |
P-selectin | None | LS vs patients without stroke | (149) |
platelet microparticles | None | LS vs patients without stroke | (149) |
Inflammation | |||
alkaline phosphatase | ↑ | Silent LS vs large vessel atherosclerosis | (151) |
C-reactive protein | ↑ | LS vs patients without stroke | (152) |
C-reactive protein | None | LS vs cortical stroke | (152) |
interleukin-6 | ↑ | LS vs patients without stroke | (147) |
interleukin-6 | ↓ | LS vs cortical stroke | (147) |
interleukin-6 | ↑ | LS with major vascular events vs without | (155) |
tumor necrosis factor | ↑ | LS vs patients without stroke | (147) |
tumor necrosis factor | ↑ | LS with major vascular events vs without | (155) |
neopterin | ↑ | LS vs hypertensive controls | (154) |
Adhesion | |||
intercellular adhesion molecule-1 | ↑ | LS vs hypertensive controls | (154) |
vascular cadherin adhesion molecule-1 | ↑ | LS vs hypertensive controls | (154) |
vascular cadherin adhesion molecule-1 | ↑ | Blacks with LS vs those without | (153) |
integrin alpha-IIb | ↑ | LS with adverse outcome vs LS without | (158) |
talin-1 | ↑ | LS with adverse outcome vs LS without | (158) |
filamin-A | ↑ | LS with adverse outcome vs LS without | (158) |
Lipids | |||
glucosylceramide | ↑ | LS vs patients without stroke | (156) |
phosphatidylethanolamine | ↑ | LS vs patients without stroke | (156) |
free fatty acids | ↑ | LS vs patients without stroke | (156) |
triacylglycerol | ↑ | LS vs patients without stroke | (156) |
omega 3-polyunsaturated fatty acids | ↓ | LS with cSVD vs without | (157) |
Other | |||
myelin basic protein | ↑ | LS with adverse outcome vs LS without | (158) |
albumin | ↓ | LS with adverse outcome vs LS without | (158) |
LS (lacunar stroke), cSVD (cerebral small vessel disease).
One comprehensive study compared several biomarkers in nondisabling LS versus cortical stroke after one month; the LS group had decreased tissue plasminogen activator, which was the only biomarker found to be significantly different.(148) Another study enrolled recent LS patients and showed that markers of platelet activation (activated glycoprotein IIb/IIIa, P-selectin, and platelet microparticles) were not different from healthy controls, however markers of endothelial dysfunction (von Willebrand factor and homocysteine) were increased.(149) Patients with essential HTN and WMH or lacunes, versus HTN alone, were enrolled in another study that showed that endothelial progenitor cells (which may ameliorate endothelial dysfunction) and angiogenic T-cells (which stimulate endothelial progenitor cells) were both decreased in number.(150)
Serum alkaline phosphatase, posited to be a marker of vascular calcification, was shown to be associated with silent lacunes but not large vessel atherosclerosis in neurologically healthy patients.(151) Alkaline phosphatase is an acute phase reactant so this association could be due to inflammation, which underlies the pathophysiology of LS, at least in part.(45) Another study of healthy patients showed a correlation of higher C-reactive protein levels and number of silent lacunes.(152) One small study of African Americans with LS revealed they had elevated pro-inflammatory biomarkers, soluble vascular cadherin adhesion molecule-1 and thrombin anti-thrombin, and an abnormal response to acute immune challenge six weeks after stroke.(153) Vascular inflammation was also assessed in a study of first-time LS and essential HTN controls. Neopterin and circulating adhesion molecules were higher in patients with MRI-confirmed cSVD.(154) The higher Levels of Inflammatory Markers in the Treatment of Stroke (LIMITS) study (ancillary biomarker study within SPS3) examined patients with recent LS and showed interleukin-6 and tumor necrosis factor receptor concentrations predicted risk of recurrent vascular events.(155)
In addition to these three categories of biomarkers, other novel potential biomarkers are under evaluation. One group developed a plasma panel for lipid species, including glucosylceramide, phosphatidylethanolamine, free fatty acid, and triacylglycerol, that was 93% sensitive and 97% specific for LS compared to healthy controls.(156) Another study found low levels of plasma omega 3-polyunsaturated fatty acids were associated with markers of cSVD, though perhaps less so in LS, when patients were enrolled 7 days after stroke.(157) Using quantitative proteomics of microvesicle enriched plasma, one study showed that the patients with adverse outcomes had decreased albumin and increased brain-specific proteins (myelin basic protein), coagulation cascade proteins (fibrinogen alpha chain, fibrinogen beta chain), and focal adhesion proteins (integrin alpha-IIb, talin-1, and filamin-A).(158)
The literature investigating biomarkers can be difficult to interpret given the heterogeneity of stroke and the many variables involved. Future biomarker studies should be hypothesis-driven, specifically evaluating the unique properties of cSVD-induced LS compared to other stroke subtypes. This involves including controls, such as patients with HTN without cSVD and patients with other sub-types of stroke. Duration after stroke onset, size of stroke, and location of stroke are all also important considerations. Lastly, studies should evaluate features that are unique to the cerebral vasculature that may differentiate cSVD from systemic thrombosis and systemic vessel diseases. Indeed, it has been suggested that cSVD may be a component of a systemic disease, affecting multiple organs by similar pathophysiological mechanisms causing systemic arteriolar dysfunction.(159) An example is the association between WMH, dementia, and retinal vascular abnormalities.(159) Moreover, chronic kidney disease may be linked to WMH, demonstrated in a study that showed patients with kidney disease had increased WMH.(160) Further studies showed that patients with reduced creatinine clearance have increased WMH volume.(161)
Genetics of Lacunar Stroke
Although LS is a multifactorial disease that is associated with numerous risk factors, there are considerable differences in susceptibility to these risk factors when comparing individuals. Moreover, there are examples of patients that develop cSVD in the absence of risk factors, suggesting a role for genetic modulation(162). Family history of vascular disease is an independent risk factor for LS.(163) There is also evidence from twin and family studies that WMH, related to LS, has a genetic basis.(21,164,165) One study examined 74 monozygotic and 71 dizygotic twins, showing a 73% heritability for WMH volume.(166) Moreover, concordance rates for WMH were 61% among monozygotic twins and 38% in dizygotic twins. Another heritability study utilized a family-based study design from the Framingham heart cohort.(167) WMH volume was assessed in over 2000 patients who were free of stroke. The results of this study demonstrated a WMH volume heritability of 78% among woman and 52% among men.
Apart from these heritability studies, there are several single gene disorders that are linked to mutations that precipitate cSVD. These account for about 1 to 5% of all strokes.(168) Among the major diseases are Fabry’s disease, CADASIL, CARASIL,(169) COL4A1-related cSVD,(170) and autosomal dominant retinal vasculopathy with cerebral leukodystrophy.(171) The contribution of these individual genes in developing spontaneous cSVD is unknown; however, a more complete understanding of these disorders may provide insight into its pathogenesis.(172)
Candidate gene studies have been a popular method to study complex polygenic disorders, and employ the use of SNP analysis in genes that are suspected to play a role in the disease.(173) Genes that encode for angiotensin-converting enzyme (ACE) and endothelial nitric oxide synthase (eNOS) are among some candidate genes that have been examined. The deletion (DD) genotype for ACE and the GG genotype of Glu298Asp eNOS polymorphism(174) have been associated with all ischemic stroke(175) and specifically LS.(176) Another candidate gene that has been extensively studied is the apolipoprotein E (ApoE) gene. ApoE ϵ4 allele carriers had a significantly higher subcortical (and not periventricular) WMH burden when controlling for HTN.(177) Since apoE is involved in lipid metabolism(178) and neuronal repair,(179) it raises the question whether it may be involved in the pathophysiology of subcortical LS. Some speculate that the D allele of ACE may share a similar pathway as ApoE ϵ4 and may be implicated in the development of WMH.(180) As with many candidate gene studies, sampling bias and poor phenotyping are limitations.(181) Larger studies failed to replicate this finding, and only showed an association between the ACE I/D polymorphism and WMH.(182)
One novel method that circumvents the arduous task of examining individual genes has been genome wide association studies (GWAS). Applying this methodology to two independent Japanese samples with LS revealed a nonsynonymous SNP in a member of the protein kinase C family, PRKCH.(183) This protein is expressed in endothelial cells, macrophages, and smooth muscle cells of atherosclerotic plaques. Similar findings were recapitulated in subjects with silent lacunes.(184) In GWAS examining WMH, 6 SNPs were associated with larger WMH volumes (although the distribution of these lesions was not examined). These SNPs were located near WBP2, TRIM65, TRIM47, MRPL38, FBF1, and ACOX.(185) In another such GWAS study conducted in the Netherlands, chromosome 5q12 was shown to have increased susceptibility to stroke.(186) Further mapping of this locus revealed that SNPs near the PDE4E gene are intimately tied to stroke risk.(187) The authors showed that the C allele of SNP45 and the T allele of SNP39 increased the risk of LS by 4.8 and 6.3 times, respectively.(188) A recent GWAS was performed on over 1000 early-onset LS patients.(189) On the basis of histopathological features and radiographic findings, the authors defined two subsets of LS: isolated LS and multiple LS with leukoaraiosis. There was a significant heritable component to LS of around 20-25%, with isolated LS at 15–18% and multiple LS/leukoaraiosis at 23–28%. This argues for the presence of LS subtypes.
GWAS have been criticized as they are underpowered to detect rare variants which could contribute higher risk than common variants, which generally confer a small relative risk.(181) To assess for this, the use of non-hypothesis-driven methodology using large sample sizes is required. In this regard, whole exome sequencing using next-generation technology allows for the analysis of the entire coding sequences in the human genome. A small study using exome sequencing was performed in the Chinese Han population.(190) A SNP in the c1orf156 gene located on 1q24 and another in the XYLB gene located on 3p21 were identified as being related to ischemic stroke. These genes have been implicated broadly in cardiovascular disease. Another whole exome sequencing study examined ten patients, five of which had LS.(191) Two genes, CSN3 and HLA-DPB1, were implicated among the LS group. Interesting, the CSN3 gene has been associated with DM and coronary artery atherosclerosis.(192,193) A study utilizing next generation sequencing on LS specifically is currently underway;(194) it may provide further insight into LS pathogenesis. Although the exact genetic determinants of cSVD remain to be clarified, it is likely that it is a polygenic phenomenon involving an interplay between many genetic variants across the genome and environmental factors.(172)
Modeling Small Vessel Disease and Lacunar Stroke
A major barrier to the study of cSVD and LS is that animal and cellular models that accurately replicate the vessel and parenchymal pathology in humans are only recently being developed. For many of the basic questions regarding mechanism, experimental models may enhance our understanding. Models must be chosen carefully, however, as no single model perfectly replicates the human phenotype. Furthermore, several models may be required in complementary fashion to capture both vessel and parenchymal pathology and replicate findings. There are three main categories of animal models: chronically hypertensive animals, chronically hypoperfused animals, and animals that undergo targeted small focal infarcts.(195)
The stroke-pone Spontaneously Hypertensive Rat (SHR-SP) is an example of a chronically hypertensive model.(196) Compared to control rats, SHR-SP show reduced endothelial tight junctions, reduced nitric oxide bioavailability, impaired myelination, increased glial and microglial activity, impaired matrix proteins, impaired vascular reactivity, and reduced albumin.(197) Established in 1974,(198,199) this inbred rat strain spontaneously develops an arteriopathy that may include cerebral edema, ischemia/infarction, and ICH.(78,196,200,201) The pathology was similar to humans, in that strokes occurred at arteries that branch from larger ones.(201) A decade later, a more detailed histologic description was made through serial sectioning that showed BBB degradation and vasogenic edema.(200) At the leakage sites, they identified fibrinoid degeneration of penetrating arterioles. There was a loss of detectable vessel wall components and a thickening of the vessels with expanded perivascular tissue space. They also described occlusive fibrin thrombi and noted the formation of necrotic cysts, though some parenchymal lesions were a milder variation of edema. Fibrin thrombi were found close to the cysts, though it was argued that these thrombi may form secondarily to a primary leakage event rather than causing a primary ischemic event themselves. The same group later showed that damaged endothelial and smooth muscle cells were replaced by fibrin-like material, basement membrane thickening, and bundles of collagen fibrils.(78) They found these vascular lesions occurred in short segments similar to the description of human lesions by Fisher. Interestingly, more modern studies of SHR-SP have shown the endothelial structural changes predate HTN. The changes included reduced endothelial tight junctions, increased smooth muscle actin and GFAP, as well as altered matrix metalloproteinase 9 compared to controls.(202) A recent detailed systematic review deemed SHRSP the most appropriate model for human cSVD available,(203) and it lends itself to easy study of neuroprotective agents.(196) However, another recent study suggests it also models posterior reversible encephalopathy syndrome.(204)
In addition to modeling the vascular pathology, novel models are being developed to study the parenchymal pathology including cell, tissue, and animal-based platforms.(205) While the traditional middle cerebral artery occlusion (MCAO) model does damage oligodendrocytes and white matter in response to ischemia,(206) it does not target white matter specifically.(207,208) The bilateral carotid occlusion model of chronic hypoperfusion has been shown to induce white matter ischemia.(209,210) This model causes changes in white matter that resemble human WMH(211–213) and cognitive impairment.(214)
For focal infarcts targeted to any desired brain region, laser coagulation models(215) and stereotactic parenchymal injection of vasoconstrictors can be utilized. Common vasoconstrictors include endothelin-1 (ET-1)(208) and N5-(1-iminoethyl)-L-ornithine (L-NIO)(216), the latter being preferred as it avoids receptor mediated effects. If injected into the subcortical white matter, L-NIO creates a lesion presumably from small vessel occlusion and induces a phenotype similar to human LS.(216) Cognitive impairment can also be assessed in these models, further demonstrating translatability.(217) In this model, several histologic changes have been reported after white matter stroke, including focal edema, demyelination, axonal damage, loss of oligodendrocytes, and local activation of astrocytes and microglia(117). One mouse model showed that the size of these strokes grew over the first month,(117) possibly representing vulnerable hypomyelinated peri-infarct axons. In this peri-infarct region, myelin loss was found to exceed axon damage. Furthermore, microglia/macrophages were found near injured axons at day 1, and their presence continued to increase until day 7.
In addition, transgenic mouse models can be utilized. COL4A1/2 models replicate cSVD with ICH,(218) while Notch3 models exhibit WMH largely without vessel pathology.(219) Lastly, there are in vitro models of the BBB and also of neurons, oligodendrocytes, and other glia culture systems that can undergo a multitude of experiments, including oxygen/glucose deprivation.(220)
Conclusion
LS poses a major burden to society in terms of morbidity and mortality. They comprise a large portion of strokes and can be particularly devastating given their stuttering progressive nature, their risk of recurrence, and their cumulative effects on cognition. Since their early discovery in the late 1800s and their detailed description by Fisher in the 1960s, there has been steady progress in the understanding of their pathophysiology. Although LS is multifactorial, cSVD is the major mechanism that contributes to LS. CAA is another common cerebrovascular pathology emerging as a cause of both hemorrhagic and ischemic brain lesions, including LS. It is important to conceptualize LS as a process involving both small vessel and parenchyma pathologies, though they are intimately related. With developments in imaging, CMI may provide additional clues into LS pathogenesis and the relationship of LS to other imaging markers of cSVD can be further examined. Until recently, cSVD has been poorly understood as it is a complex phenomenon that is influenced by genetics and environmental risk factors. Although there has been improvement in understanding this disease, it has not translated into effective targeted therapies for LS. Further research using a variety of strategies including preclinical models and advanced neuroimaging is needed to further unravel the processes that underlie this common disease of older adults.
Acknowledgements
Sources of Funding
Funding for this study was provided by grants from the National Institutes of Health (Robert W. Regenhardt, NS065743; Eng H. Lo, NS055104, NS099620, NS103786, AG055559, NS093415; Cenk Ayata, NS102969; M. Edip Gurol, NS083711).
ACRONYM DEFINITION
ACE | angiotensin-converting enzyme |
ApoE | apolipoprotein E |
ASCO | Atherosclerosis, Small-vessel disease, Cardiac pathology, Other causes |
ASCOD | Atherosclerosis, Small-vessel disease, Cardiac pathology, Other causes, Dissection |
CAA | cerebral amyloid angiopathy |
CBF | cerebral blood flow |
CCS | Causative Classification of Stroke |
CMB | cerebral microbleed |
CMI | cerebral microinfarct |
cSVD | cerebral small vessel disease |
CT | computed tomography |
DM | diabetes mellitus |
DWI | diffusion-weighted imaging |
eNOS | endothelial nitric oxide synthase |
ET-1 | endothelin-1 |
GWAS | genome wide association studies |
HTN | hypertension |
LIMITS | Levels of Inflammatory Markers in the Treatment of Stroke |
L-NIO | N5-(1-iminoethyl)-L-ornithine |
LS | lacunar stroke |
MCAO | middle cerebral artery occlusion |
MRI | magnetic resonance imaging |
Nogo | neurite outgrowth inhibitor |
OPC | oligodendrocyte precursor cell |
SHR-SP | stroke-pone Spontaneously Hypertensive Rat |
STRIVE | STandards for ReportIng Vascular changes on nEuroimaging |
TOAST | Trial of Org 10172 in Acute Stroke Treatment |
WMH | white matter hyperintensity |
Footnotes
Conflicts of Interest/Disclosures
The authors report no conflicts of interest or disclosures.
References
Full text links
Read article at publisher's site: https://doi.org/10.1016/j.jstrokecerebrovasdis.2019.05.006
Read article for free, from open access legal sources, via Unpaywall:
https://www.ncbi.nlm.nih.gov/pmc/articles/PMC7416422
Citations & impact
Impact metrics
Citations of article over time
Alternative metrics
Smart citations by scite.ai
Explore citation contexts and check if this article has been
supported or disputed.
https://scite.ai/reports/10.1016/j.jstrokecerebrovasdis.2019.05.006
Article citations
Development and Validation of a Nomogram for Predicting Lacunar Infarction in Patients with Hypertension.
Int J Gen Med, 17:3411-3422, 06 Aug 2024
Cited by: 0 articles | PMID: 39130489 | PMCID: PMC11316493
Differential blood-based biomarkers of subcortical and deep brain small vessel disease.
Ther Adv Neurol Disord, 17:17562864241243274, 31 May 2024
Cited by: 0 articles | PMID: 38827243 | PMCID: PMC11143814
Protective effects of fecal microbiota transplantation against ischemic stroke and other neurological disorders: an update.
Front Immunol, 15:1324018, 21 Feb 2024
Cited by: 3 articles | PMID: 38449863
Review
FLAIR signal intensity ratio predicts small subcortical infarct early neurologic deterioration: a cross-sectional study.
Neuroradiology, 66(3):343-347, 26 Jan 2024
Cited by: 0 articles | PMID: 38273104
Stroke Subtype Among Individuals With Chronic Kidney Disease.
Can J Kidney Health Dis, 10:20543581231203046, 14 Oct 2023
Cited by: 0 articles | PMID: 37841343 | PMCID: PMC10576427
Go to all (29) article citations
Similar Articles
To arrive at the top five similar articles we use a word-weighted algorithm to compare words from the Title and Abstract of each citation.
Advances in Understanding the Pathophysiology of Lacunar Stroke: A Review.
JAMA Neurol, 75(10):1273-1281, 01 Oct 2018
Cited by: 108 articles | PMID: 30167649 | PMCID: PMC7426021
Review Free full text in Europe PMC
Advances in Understanding the Pathogenesis of Lacunar Stroke: From Pathology and Pathophysiology to Neuroimaging.
Cerebrovasc Dis, 50(5):588-596, 06 May 2021
Cited by: 13 articles | PMID: 33957622
Review
[Lacunar stroke and cerebral small vessel disease: advocacy for a recognition].
Rev Med Suisse, 10(425):782-787, 01 Apr 2014
Cited by: 0 articles | PMID: 24791423
Blood pressure gradients in cerebral arteries: a clue to pathogenesis of cerebral small vessel disease.
Stroke Vasc Neurol, 2(3):108-117, 08 Jun 2017
Cited by: 69 articles | PMID: 28989801 | PMCID: PMC5628379
Funding
Funders who supported this work.
NIA NIH HHS (1)
Grant ID: R01 AG055559
NINDS NIH HHS (7)
Grant ID: K23 NS083711
Grant ID: R01 NS102969
Grant ID: P01 NS055104
Grant ID: R25 NS065743
Grant ID: R01 NS093415
Grant ID: R01 NS103786
Grant ID: R01 NS099620