Abstract
Free full text

Lamin A/C dysregulation contributes to cardiac pathology in a mouse model of severe spinal muscular atrophy
Abstract
Cardiac pathology is emerging as a prominent systemic feature of spinal muscular atrophy (SMA), but little is known about the underlying molecular pathways. Using quantitative proteomics analysis, we demonstrate widespread molecular defects in heart tissue from the Taiwanese mouse model of severe SMA. We identify increased levels of lamin A/C as a robust molecular phenotype in the heart of SMA mice and show that lamin A/C dysregulation is also apparent in SMA patient fibroblast cells and other tissues from SMA mice. Lamin A/C expression was regulated in vitro by knockdown of the E1 ubiquitination factor ubiquitin-like modifier activating enzyme 1, a key downstream mediator of SMN-dependent disease pathways, converging on β-catenin signaling. Increased levels of lamin A are known to increase the rigidity of nuclei, inevitably disrupting contractile activity in cardiomyocytes. The increased lamin A/C levels in the hearts of SMA mice therefore provide a likely mechanism explaining morphological and functional cardiac defects, leading to blood pooling. Therapeutic strategies directed at lamin A/C may therefore offer a new approach to target cardiac pathology in SMA.
Introduction
Spinal muscular atrophy (SMA) is a debilitating genetic disorder, traditionally classified as a neuromuscular disease due to the characteristic pathology of lower motor neuron degeneration and progressive muscle wasting (1). Accumulating evidence of pathology outside of the neuromuscular system, however, suggests that SMA should now be considered as a systemic condition (2). SMA has an incidence of approximately 1 in 10000 live births (3), and in ~95% of patients, it is caused by homozygous loss of survival of motor neuron 1, telomeric, gene, resulting in insufficient levels of the ubiquitously expressed survival of motor neuron (SMN) protein (4). There is no cure for SMA, but the last few years have seen significant progress in the development of therapies aimed at alleviating symptoms by raising full-length SMN protein levels (5). Nusinersen (Spinraza™), an antisense oligonucleotide drug, is now widely available for children and young adults with SMA, and most recently, Zolgensma™ (previously known as AVXS-101), an adeno-associated virus-based gene replacement therapy, was given approval by the Food and Drug Administration for the treatment of SMA children under 2 years of age. Although undoubtedly an enormous step forward, none of the strategies that have been developed so far show complete efficiency (5–8). Coupled with uncertainties around long-term effectiveness and extremely high price of both strategies, there is keen interest to find alternative therapeutic strategies that could, in combination with SMN-targeted therapy, offer maximum therapeutic benefit to all SMA patients (9).
SMN perturbations influence organ development and function across multiple levels (2), and so it is likely that organ-specific and/or systemic therapy delivery may be necessary to fully rescue the SMA phenotype (5). For example, a systematic review of the literature in 2017 found 58 studies that reported on a total of 264 SMA patients with cardiac abnormalities (10). A common finding among the 77 patients with the most severe type of SMA (type I) was structural pathology, observed mainly in the septum and/or cardiac outflow tract. All of the 63 type II SMA patients identified in the literature search had electrocardiogram abnormalities, while the 124 patients with type III SMA had cardiac rhythm disorders and/or structural abnormalities. In addition to the numerous reports of cardiac defects among SMA patients, the systematic review identified 14 studies that have documented cardiac pathology in mouse models of SMA (10). Common macroscopic findings include decreased heart size and decreased thickness of the left ventricular wall and interventricular septum, while a frequent microscopic observation was cardiac fibrosis, which was detected at a pre-symptomatic stage of the disease in both severe and intermediate mouse models of SMA (10–12). In addition, almost all studies of SMA mouse models reported bradyarrhythmias (10). A more recent study of a severe mouse model of SMA at pre- and early symptomatic time points confirmed many of these previous findings but also noted significant pooling of blood in the heart, together with disorganization of cardiomyocytes and lack of trabecular compaction (13). These findings strongly resemble symptoms of cardiomyopathy (13) and indicate serious consequences for the normal electrical and mechanical functioning of the heart.
A recent gene-expression study of hearts from the “Taiwanese” mouse model of severe SMA identified 205 genes that were downregulated and 269 genes that were upregulated at an early symptomatic time point (i.e. P5) (14). Several of these changes were tracked back to a pre-symptomatic time point, suggesting that cardiac defects might be attributable, at least in part, to cell autonomous mechanisms (14). To the best of our knowledge, this is the first study to date that has conducted a comprehensive analysis of molecular changes in the SMA mouse heart, and while it has generated novel insights about changes to the transcriptome, proteomic insights into the SMA heart are lacking. This is particularly important in the context of emerging evidence showing that the SMN protein plays fundamental roles in protein translation (15, 16). In this study, we have conducted a comprehensive quantitative proteomics analysis of heart tissue from the Taiwanese mouse model of severe SMA and show that there is widespread dysregulation of protein expression in SMA compared to controls. We verified the robust increase of one of these proteins, lamin A/C, in the hearts of SMA mice, and propose a role for lamin A/C in SMA cardiac pathology, strongly supported by case reports of an adult form of SMA caused by mutations in the lamin A/C encoding gene, LMNA (17, 18). As with a wide range of neuromuscular conditions caused by mutations in LMNA, including Emery–Dreifuss muscular dystrophy, limb-girdle muscular dystrophy 1B, and dilated cardiomyopathy, cardiac involvement was a notable feature in each case of LMNA-associated SMA. A role for lamin A/C in SMA is strengthened further by experiments in which we demonstrate dysregulation across other cells and tissues, and a mechanistic link between lamin A/C and ubiquitin-like modifier activating enzyme 1 (UBA1) protein, a key contributor to SMN-dependent disease pathways (19–21).
Results
Quantitative proteomics analysis of heart tissue from severe SMA mice reveals widespread molecular defects
To determine the molecular consequences of SMN depletion in the heart of the Taiwanese mouse model of severe SMA (22), a quantitative comparison of the SMA and age-matched control heart proteome was undertaken using iTRAQ™ mass spectrometry analysis. This approach identified 3105 proteins in total (Supplementary Material, Table S1), of which 2479 were identified with a 5% local false-discovery rate. For reliable quantification, proteins identified from just a single peptide were removed, after which, differentially expressed proteins were identified by removal of proteins with a fold change of <1.25 and, finally, exclusion of proteins with a P-value of >0.05 assigned to their fold changes. This left 383 proteins that met the specified criteria for differential expression, of which 177 proteins were increased and 206 were decreased in expression in SMA mouse heart compared to controls (Supplementary Material, Table S2). Twenty-one of the differentially expressed proteins were also altered in expression at the gene level in a recent microarray study of hearts from SMA mice at an early symptomatic time point (i.e. P5) (14), of which 16 followed the same direction of differential expression (Supplementary Material, Table S3).
Gene ontology (GO) analysis using the Database for Annotation, Visualization and Integrated Discovery (DAVID) platform (23, 24) highlighted enriched biological processes and cellular components relating to the up- and downregulated proteins, respectively (Supplementary Material, Table S4). A relatively high proportion of upregulated proteins were found to be blood related (n=
16), and although this compliments a previous report of blood pooling in the hearts of the Taiwanese SMA mouse model (13), these proteins were excluded from further analysis to retain focus on changes specific to the heart tissue. After also removing keratin-associated proteins (n
=
4), the remaining proteins were then subject to analysis using Search Tool for the Retrieval of Interacting Genes/Proteins (STRING) 10 (25) to identify statistically significant associations between them. Comparison of the resulting networks with the GO analysis output (Supplementary Material, Table S4) identified protein clusters associated with highly enriched molecular and/or biological processes. For the upregulated proteins, enriched processes included organization and/or regulation of the cytoskeleton, cell junction, and extracellular matrix (Fig. 1A), while clusters of downregulated proteins were associated with translation and metabolic processes (Fig. 1B).
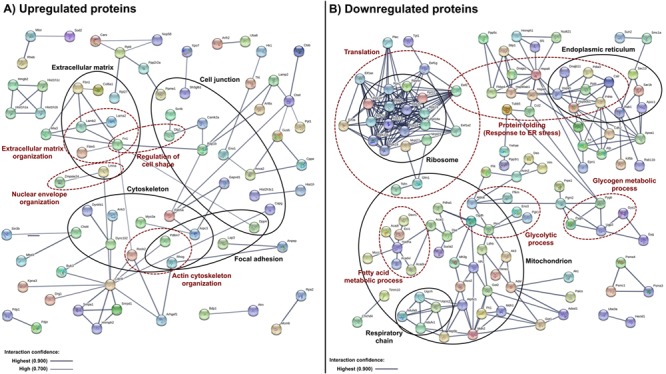
Bioinformatics analysis of the proteins dysregulated in heart tissue from SMA mice. (A) Upregulated proteins (n=
177) and (B) downregulated proteins (n
=
206) were subjected to STRING 10 analysis. Of these, 103 upregulated and 91 downregulated proteins are not shown as statistically significant interactions were not identified in STRING 10. Protein associations were identified with high confidence (0.700) interaction score for (A) upregulated and with the highest confidence (0.900) interaction score for (B) downregulated proteins. The thickness and color of the lines indicate confidence of the interaction (see legend inset in figure). Protein networks were compared to the GO analysis output (Supplementary Material, Table S4). The analysis identified protein clusters that associate with enriched GO terms in the cellular component (black) and biological process (red) domain. Proteins annotated to each term are shown in corresponding circles.
Lamin A/C expression is dysregulated in severe SMA mice and SMA patient fibroblasts
One of the 383 differentially expressed proteins, lamin A/C, was of particular interest to us since mutations in LMNA, the lamin A/C encoding gene, are known to cause an adult form of SMA (17, 18). Additionally, the analysis of published proteomic studies of the neuromuscular system in SMA (26) identified lamin A/C as a conserved molecular change across three separate studies of SMA (27–29). To date, however, these findings do not appear to have been verified, nor has lamin A/C been studied in the context of SMN-dependent pathways in heart. We therefore chose to verify and understand the individual contributions of lamins A and C to the overall lamin A/C expression trend, using quantitative western blotting of heart tissue extracts from postnatal day 8 (P8) SMA mice and healthy littermate controls (see workflow in Fig. 2A). This analysis confirmed a robust increase in lamin A (69%, P=
0.0007) and C (91%, P
=
0.0079) expression in SMA compared to control heart extracts (Fig. 2B and C). Immunohistochemical analysis of SMA and control mouse heart sections revealed few lamin A/C positive cells in the ventricle lumen (Fig. 2D), and although we cannot rule out a minor contribution, this result confirms that the increased lamin A/C levels cannot be solely attributed to circulating blood cells. Indeed, densitometry analysis of lamin A/C expression confirmed a robust upregulation of lamin A/C levels in the ventricle wall of SMA mouse heart sections compared to the unaffected controls (84%, P
=
0.0002) (Fig. 2E).
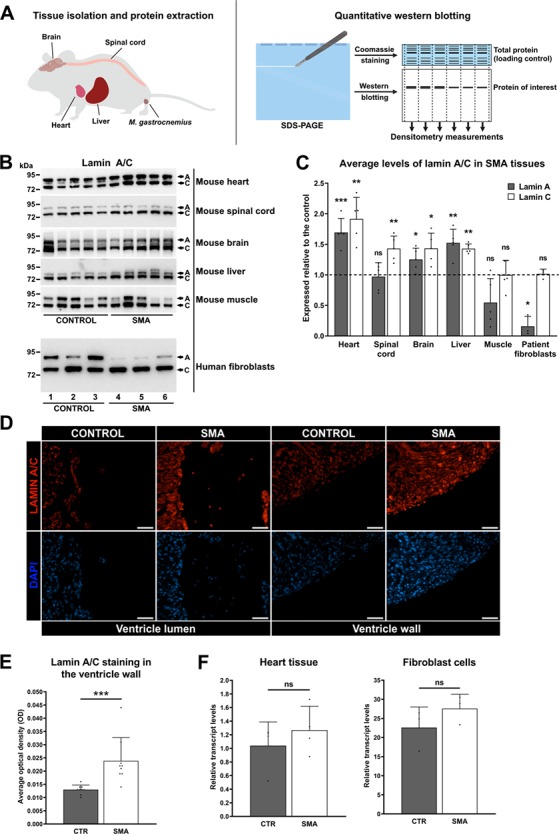
Widespread dysregulation of lamin A/C expression in fibroblasts from SMA patients and in tissues from SMA mice (P8). (A) Schematic diagram showing the quantitative western blot analysis workflow. Total protein extracts from human fibroblast cells and mouse tissues were subjected to SDS–PAGE. A part of the gel was stained with Coomassie blue as an internal, total protein loading control. Proteins from the remaining part of the gel were transferred to nitrocellulose membrane and developed with the appropriate antibody. Quantification of protein levels was performed by normalizing densitometry measurements of antibody reactive bends to densitometry measurements of Coomassie stained gel. Image created with BioRender.com. (B) Representative western blots showing lamin A/C protein levels in the heart, spinal cord, brain, liver and muscle tissue from SMA mice (n=
5) and healthy controls (n
=
5), and in fibroblast cells from SMA patients (n
=
3) and age-matched male healthy controls (n
=
3). Fibroblast cells are listed in this order: 1. GM00498, 2. GM05659, 3. GM00302, 4. GM03813, 5. GM09677 and 6. GM00232. The uncropped blotsand total protein loading controls can be found in the Supplementary Material, File S3. (C) Quantification of lamin A/C levels in tissues from SMA mice and in patient fibroblast cells. In the liver extract, the lower band was presumed to be lamin C, and the upper two bands were presumed to be lamin A isoforms and were consequently measured together to give a single value. The graph is presented as average protein levels in SMA tissues (expressed relative to the control), with error bars showing standard deviation from the mean. The dashed line represents protein levels across healthy control tissues. (D) Representative immunohistochemistry images showing lamin A/C staining in the heart from SMA mouse (P8) and healthy age-matched control. Scale bar
=
50 μm. Increased lamin A/C staining can be observed in the ventricle wall from SMA mouse, with few lamin A/C positive cells found in the ventricle lumen. (E) Densitometry measurements of lamin A/C levels in the ventricle wall are presented as mean OD, with error bars showing standard deviation from the mean. (F) Lamin A transcript levels in the heart from SMA mice (n
=
4) and healthy controls (n
=
4) and in fibroblast cells from SMA patients (n
=
3) and healthy controls (n
=
3). Expression levels of lamin A were normalized to the geometric mean of POLR2J and TBP. The graphs are presented as mean expression, with error bars showing standard deviation from the mean. ns, not significant; *P
<
0.05; **P
<
0.01; ***P
<
0.001.
Protein extracts of SMA and control mouse brain, spinal cord, muscle, and liver tissue, as well as control and SMA patient dermal fibroblast extracts were analyzed by quantitative western blotting to determine whether lamin A/C dysregulation extends beyond the heart. These analyses revealed widespread dysregulation of both lamin A and C, with differing directions of expression change depending on the tissues examined (Fig. 2B and C). A statistically significant reduction of lamin A levels was seen in the SMA patient fibroblast cells compared to those from healthy controls (84%, P=
0.0438), while a statistically significant upregulation of lamin A levels was found in the brain (25%, P
=
0.0434) and liver (52%, P
=
0.0026) tissue from SMA mice. Spinal cord extracts from SMA mice did not show a significant change in lamin A expression compared to the healthy controls, but they did show a significant increase in the levels of lamin C (43%, P
=
0.0078), as did the liver (42%, P
=
0.0075) and brain (43%, P
=
0.0116). Patient fibroblast cells, on the contrary, showed no significant change in lamin C expression compared to the healthy controls, despite having a drastic reduction in lamin A levels. It was not possible to reliably determine whether lamin A or C were dysregulated in the skeletal muscle extracts due to large variability between samples, for reasons we are unable to explain. Lamin A dysregulation in SMA patient fibroblasts and SMA mouse heart (where protein levels were lowest, and highest, compared to controls, respectively) appears to occur post-transcriptionally, since reverse transcription quantitative polymerase chain reaction (RT-qPCR) analysis showed no significant change in lamin A transcript levels in SMA compared to controls (Fig. 2F).
Lamin A/C and UBA1 are mechanistically linked
Having established the widespread dysregulation of lamin A/C levels across SMA patient fibroblasts and mouse tissues (Fig. 2), we next wanted to investigate whether there is a relationship between lamin A/C and UBA1. The justification for this is that a role for UBA1 in SMN-dependent pathways has been well characterized across several models of SMA (19–21), and previous research has shown that, like UBA1 (19), lamin A/C is implicated in the regulation of the β-catenin signaling pathway (30, 31). In addition, a previous study reported decreased levels of UBA1 expression across a range of non-neuronal tissues from the Taiwanese mouse model of SMA compared to controls, with the heart being most severely affected, followed by the liver (20). This is of particular interest in context with the current study, since heart tissue from SMA mice showed the greatest increase of lamin A/C levels across the tissues examined, also followed by the liver (Fig. 2) (SMA patient fibroblasts appear to be an anomaly, as they were the only sample to show reduced lamin A levels compared to controls but had unchanged levels of UBA1; Supplementary Material, File S4). To investigate the relationship between lamin A/C, UBA1 and SMN, a western blot study of their relative protein expression levels was conducted using equal amounts of total protein from heart, muscle, liver, brain and spinal cord tissue extracts from healthy control mice and from healthy human fibroblasts. This revealed a strong inverse pattern of lamin A/C and UBA1 expression (Fig. 3A) whereby the cells/tissues typically considered under the most mechanical strain (i.e. fibroblast cells, heart and muscle tissue) had high relative levels of lamin A/C and low relative levels of UBA1. Tissues under the least mechanical strain (i.e. liver, brain and spinal cord) showed the opposite pattern, with low relative levels of lamin A/C and high relative levels of UBA1 (Fig. 3A). SMN followed the same expression trend as UBA1 across the tissues examined (Fig. 3A).
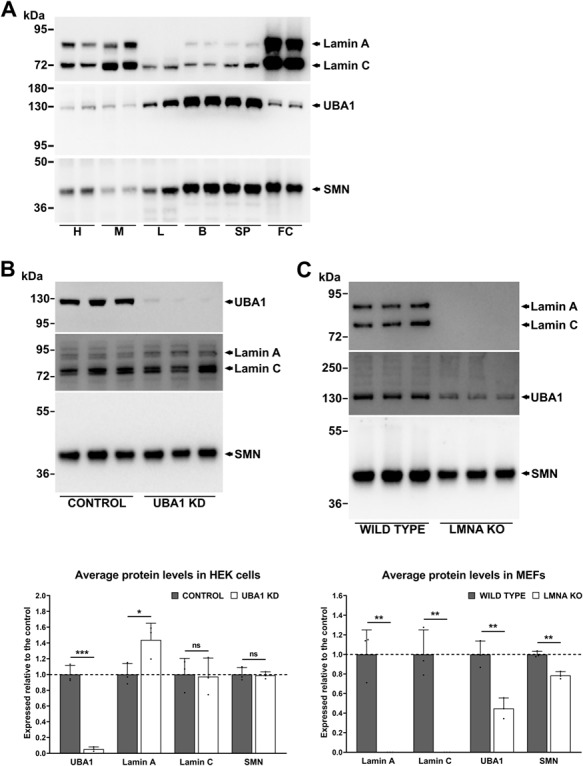
UBA1 and lamin A/C are mechanistically linked. (A) Western blots showing lamin A/C, UBA1 and SMN protein levels in the heart, muscle, liver, brain and spinal cord tissue from healthy control mice (n=
2) and in fibroblast cells from healthy individuals (n
=
2), in (B) UBA1 KD and control HEK cells (n
=
3) and in (C) LMNA KO and wild-type MEFs (n
=
3). The uncropped blots and loading controls can be found in the Supplementary Material, Files S5 and S6. Graphs are presented as average protein levels (expressed relative to the control), with error bars showing standard deviation from the mean. The dashed line represents protein levels in control cells. H, heart; M, muscle; L, liver; B, brain; SP, spinal cord; FC, fibroblast cells; ns, not significant; *P
<
0.05; **P
<
0.01; ***P
<
0.001.
Having established an inverse correlation between UBA1 and lamin A/C expression across a range of tissues, we next wanted to determine whether a mechanistic link exists between them. Knockdown of UBA1 expression in human embryonic kidney (HEK) cells resulted in a ~43% upregulation of lamin A expression (P=
0.0398), independent of changes to lamin C and SMN (Fig. 3B). We also examined mouse embryonic fibroblast cells lacking the LMNA gene (32) and found that UBA1 (55%, P
=
0.0053) and SMN (21%, P
=
0.0016) were significantly reduced compared to the control (Fig. 3C). Immunostaining of control and SMA patient fibroblasts showed colocalization between UBA1 and lamin A at the nuclear periphery in control and SMA cells (Fig. 4A), indicating a possible interaction between lamin A/C and UBA1. To investigate this further, an immunoprecipitation from control and SMA mouse heart extracts was performed using a lamin A/C monoclonal antibody attached to magnetic beads. Although both lamin A/C and UBA1 were easily detected in SMA and control heart extracts by western blot analysis, UBA1 was undetectable in the eluates following pulldown (Fig. 4B). It is important to note though that an interaction between lamin A/C and UBA1 cannot be ruled out on this basis since physiological interactions may occur between a minor proportion of the total protein present and/or it may be vulnerable to disruption by protein extraction methods (33). Interestingly, however, we did find that a small proportion of the total β-catenin coimmunoprecipitated with lamin A/C from both SMA and control heart extracts, providing evidence that lamin A/C interacts with β-catenin in the heart under normal physiological conditions (Fig. 4B). When taken together, these experiments demonstrate a mechanistic link between lamin A/C and UBA1, a proven contributor to SMN-dependent disease pathways, and highlight β-catenin signaling as a potential pathway upon which they both converge.
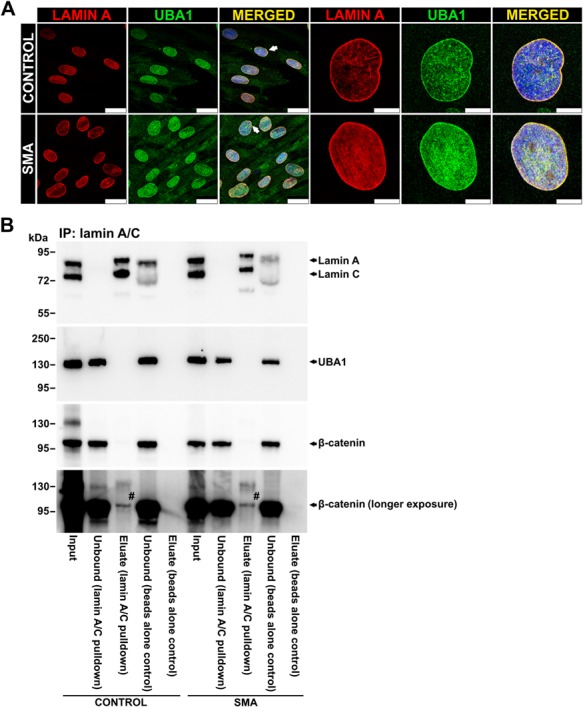
Lamin A/C interacts with β-catenin in mouse heart. (A) Representative immunocytochemistry images showing lamin A and UBA1 staining in control and SMA patient fibroblast cells. Arrows in the lower magnification images indicate cells with the most obvious UBA1 staining at the nuclear periphery. Scale bar=
25 μm. Scale bar
=
10 μm (control) and 7.5 μm (SMA) in higher magnification images. (B) Western blots showing lamin A/C, UBA1 and β-catenin expression in the heart tissue from control and SMA mice after immunoprecipitation with anti-lamin A/C antibody. Beads alone control lanes refer to heart extracts incubated with Dynabeads without the antibody and represent negative control. #β-catenin band was detected in the eluate from lamin A/C pulldown in both control and SMA samples but was not observed in the eluate from the beads alone control.
Discussion
A small percentage of SMA cases are associated with mutations in genes other than SMN, including UBA1 (34), glycyl-tRNA synthetase 1 (GARS1) (35) and LMNA (17, 18). Mutations in the UBA1 gene, which encodes the UBA1 protein, cause a form of X-linked infantile SMA (spinal muscular atrophy, x-linked 2) (34), and a role for UBA1 in SMN-dependent pathways has also been well characterized across several models of SMA (19–21). Mutations in GARS1 are known to cause infantile (36, 37) as well as an adult onset type of SMA (35). Dysregulation of UBA1/GARS1 pathways disrupted sensory neuron fate and altered sensory-motor connectivity in SMA mice, both of which were corrected following restoration of UBA1 levels (21). Unlike UBA1 and GARS1, however, the involvement of LMNA in SMN-dependent disease pathways has so far been unexplored. Here, we demonstrated that there are widespread molecular defects in heart tissue from the Taiwanese mouse model of severe SMA, one of which is the protein product of the LMNA gene, lamin A/C. We verified the robust increase of lamin A/C in the hearts from SMA mice and have identified a mechanistic link between UBA1 and lamin A/C.
The A-type lamins, lamin A and C, are encoded by LMNA gene and, in addition to providing structural support to the nucleus, are involved in several other functions including mechanosignaling, chromatin organization and regulation of gene expression (38). Mutations in LMNA gene cause a spectrum of disorders known as laminopathies, the majority of which prominently feature cardiac pathology. The adult form of SMA caused by LMNA mutations is no exception to this, as each of the patients described experienced cardiac problems with disease progression and required a pacemaker. Examination of family history in each case also revealed a high frequency of cardiac abnormalities and sudden unexplained cardiac deaths (15, 16). This indisputable link between lamin A/C mutations and cardiac pathology in a wide range of conditions, including a rare form of SMA, leaves very little doubt that correct functioning of lamin A/C is a key requirement for the maintenance of cardiac health. The finding here that lamin A/C is robustly increased in heart tissue from a mouse model of SMA therefore strongly suggests that lamin A/C is responsible, at least in part, for previously reported cardiac defects in SMA.
Lamin A/C plays a significant role in the regulation of cell stability and cell dynamics (39), and its expression levels correlate with tissue stiffness, where rigid tissues have the highest levels and softer tissues the least (40). This therefore suggests that changes in lamin A/C expression may be most pathologically relevant in SMA in highly mechanically active tissues such as the heart, where increased expression of lamin A/C is likely to impair its proper functioning. Indeed, increased rigidity of human and rat cardiomyocytes increased the extent to which cells/tissues resist deformation (i.e. passive tension) in vitro (41, 42), and in vivo rat experiments showed that higher passive tension slows the dynamics of myocardium contractions and induces functional heart defects (42). Increased cardiomyocyte rigidity was also identified in a cell model carrying a mutation in the LMNA gene (43). This mutation causes severe dilated cardiomyopathy in humans (43), which strongly mirrors the phenotype previously observed in severe and mild mouse models of SMA, including thinning of ventricle walls and interventricular septum (11, 13), and dilation of ventricles (13, 44, 45). The pathological end-point of dilated cardiomyopathy is systolic heart failure where the heart cannot pump blood properly. This would be evidenced by decreased ejection fraction and blood pooling in ventricles; both of which were identified in SMA mice (13, 44–46) and SMA patients (18, 47, 48). In the context of SMN-dependent SMA, it seems highly likely, therefore, that increased levels of lamin A/C would lead to stiffening of the cardiomyocytes, impeding their ability to contract properly and inducing the cardiac defects previously reported in SMA. In addition, cardiac fibrosis has been described in patients harboring a LMNA mutation (49, 50) and was frequently reported in SMA patients and mouse models of SMA (10), even at a pre-symptomatic stage of the disease in both severe and intermediate mouse models of SMA (11, 12). As cardiac fibrosis is a key regulator of myocardial rigidity (42), it is therefore possible that this might further exacerbate problems described above (Fig. 5).
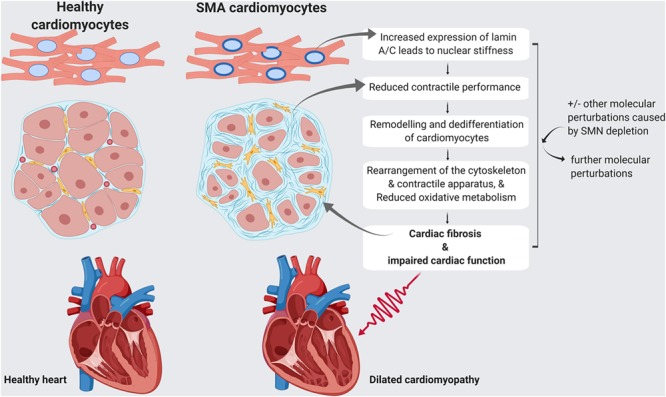
Model of how lamin A/C dysregulation, in combination with other molecular changes, may contribute to cardiac pathology in SMA. A model is proposed in which lamin A/C upregulation, in combination with other molecular changes that occur in SMA, may lead to cardiac fibrosis and impaired cardiac function. Thinner ventricle walls and dilated ventricles in SMA heart are clinical features of dilated cardiomyopathy. Image created with BioRender.com.
A role for lamin A/C in SMA is strengthened further by our data demonstrating a mechanistic link between lamin A/C and UBA1, a key contributor to SMN-dependent disease pathways (19–21). It seems highly likely that this mechanism converges on the β-catenin signaling pathway, since both lamin A/C and UBA1 are implicated in its regulation (19, 30, 31). UBA1 controls the stability of β-catenin through the canonical ubiquitin–proteasome pathway, and deficiency in UBA1 protein levels leads to β-catenin accumulation and neuromuscular pathology in SMA (19). Defective Wnt/β-catenin signaling was found to contribute to the pathology of dilated cardiomyopathy caused by mutation in LMNA gene (30), and in a separate study, lamin A/C was shown to regulate the differentiation fate of mesenchymal stem cells (MSCs) by controlling dynamics of the Wnt/β-catenin signaling pathway (31). Lamin A/C overexpression, for example, increased nuclear levels of β-catenin and activated the Wnt signaling pathway to promote osteoblast differentiation (31). The same study also provided evidence of an interaction between lamin A/C and β-catenin by immunoprecipitation of nuclear proteins from MSCs forcibly overexpressing lamin A/C (31). This physical interaction was proposed as a mechanism by which β-catenin is translocated to the nucleus (31). Here, we further expanded the knowledge of the relationship between lamin A/C and β-catenin, by demonstrating an interaction between them in heart extracts under normal physiological conditions.
The arguments above support the hypothesis that lamin A/C dysregulation is likely to be responsible, at least in part, for previously reported cardiac defects in SMA, but other proteins identified in this screen also offer insight into the molecular defects underlying cardiac abnormalities in SMA. For example, two such proteins, SUN domain-containing protein 2 (SUN2) (51) and cell division cycle 5-like protein (Cdc5l) (52), are known lamin A/C interactors and may provide further insights into lamin A/C-associated pathways in SMA. Like LMNA, mutations in Sad1 and UNC84 domain containing 2, the gene encoding the SUN2 protein, have been associated with Emery–Dreifuss muscular dystrophy, a neuromuscular disorder often associated with cardiac defects (53). Cdc5l, on the other hand, is a known splicing complex component that appears to require lamin A/C in order to regulate its assembly and targeting to the spliceosomal complex (52). Alterations to this interaction may therefore affect splicing and gene transcription, further exacerbating the downstream molecular consequences of reduced SMN expression. With this in mind, it is interesting to note that a large proportion of the downregulated proteins in the SMA mouse heart were associated with translation, and this reenforces previous work showing that the SMN protein plays fundamental roles in protein translation (15, 16). It is also highly relevant that we found a large proportion of the downregulated proteins in the SMA heart to be associated with metabolic processes, while the upregulated proteins were strongly associated with cytoskeletal and extracellular matrix organization (Fig. 1). Imbalances in contractile performance are known to promote remodeling and dedifferentiation of adult cardiomyocytes; the consequences of which include cytoskeletal rearrangement, restructuring of contractile apparatus, and reduced oxidative metabolism (54). Ironically, it seems that these adaptive processes—presumably intended to protect the cardiomyocytes in times of mechanical and/or molecular stress—can lead to adverse consequences for the heart (54). Disruption of actin cytoskeleton, for example, can impair contractile properties of cardiomyocytes and was implicated in initiation of cardiomyocyte apoptosis (55).
Here, we have offered new insight into the molecular defects underlying cardiac abnormalities in SMA, but other important questions remain that are worthy of further attention. It would be of interest in the future, for example, to explore the functional consequences of lamin A/C dysregulation in other cells and tissues throughout the natural history of disease progression in SMA. Moreover, identification of upstream regulators of lamin A/C may help to unravel the tissue-specific regulatory mechanisms that act on lamin A/C in response to SMN depletion. Finally and most importantly, it will be crucial to determine the extent to which SMN-replacement therapies reverse the molecular defects in SMA and whether this is sufficient to fully rescue the cardiac defects widely reported across SMA patients and mouse models. When doing so, it would be important to monitor cardiac health longitudinally to determine whether new phenotypes emerge following long-term SMN-replacement therapy and with increasing age. Therapies primarily designed to target neuronal tissues, such as Nusinersen, are especially relevant in this context since they are unlikely to rescue pathological defects in SMA heart. This knowledge, together with a broader understanding of the interplay between lamin A/C and other regulators of heart function in SMA, would help to isolate the most appropriate targets for therapy design that could, in combination with SMN-targeted therapy, offer maximum therapeutic benefit to all SMA patients.
Materials and Methods
SMA mouse model
The Taiwanese mouse model (original strain purchased from Jackson Laboratories, No. 005058), heterozygous for the survival of motor neuron 2, centromeric (SMN2), transgene on Smn null background (Smn−/−;SMN2tg/+) (22) and age-matched phenotypically normal controls (Smn+/−; SMN2tg/o) were maintained in Specific-pathogen-free (SPF) facilities at the University of Edinburgh. The breeding strategy and genotyping using standard polymerase chain reaction (PCR) protocols were employed as previously described (56). Tissue was harvested at a symptomatic time point, P8. All animal work was carried under the appropriate Project and Personal Licenses from the UK Home Office (PPL:60/4569) and following local ethical review.
Quantitative proteomics analysis
Total protein extracts were prepared from the hearts of P8 SMA mice (n=
5) and healthy littermate mice (n
=
5) for isobaric tags for relative and absolute quantitation (iTRAQ) quantitative mass spectrometry analysis as previously described (57). Following trypsin digestion, peptides were labeled with iTRAQ™ tagging reagents according to the protocol in the iTRAQ kit and were assigned to each sample group as follows: 114-control and 115-SMA.
High pH reverse-phase liquid chromatography fractionation
All iTRAQ-labeled peptides were combined into one tube, concentrated using a vacuum concentrator (Thermo Savant, Thermo Fisher Scientific) and resuspended in 100 μl of buffer A (10 mm ammonium formate [NH4HCO2], 2% acetonitrile [MeCN], pH 10.0). The peptides were then fractionated by high pH reverse-phase liquid chromatography using a C18 column (XBridge C18 5 μm, 4.6×
100 mm, Waters). The column was rinsed with 96% buffer A at 1 ml/min for 6 min until the optical density (OD) on the ultraviolet chromatogram returned to the baseline. The gradient ran from 4% to 28% of buffer B (10 mm NH4HCO2, 90% MeCN, pH 10.0) for 30 min to 28–50% buffer B for 6 min. The column was rinsed in 80% buffer B for 5 min and then was reequilibrated at initial conditions with 4% buffer B for 11 min. Fractions of 0.5 ml were collected every 30 s. The UV chromatogram was analyzed, and the fractions with similar peptide concentration across the elution profile were combined to give 12 fractions. The pooled fractions were concentrated in a vacuum concentrator and resuspended in 30 μl of 0.1% formic acid (FA).
Liquid chromatography–tandem mass spectrometry analysis
One third of each fraction containing the labeled peptides was analyzed by mass spectrometry. Peptides were separated by liquid chromatography using a nanoLC Ultra 2D plus loading pump and nanoLC AS-2 autosampler chromatography system (Eksigent). Peptides were loaded with buffer A (2% MeCN, 0.05% Trifluoroacetic acid (TFA) in ultrapure water) and bound to an Acclaim PepMap100 trap (100 μm×
2 cm) (Thermo Fisher Scientific). The trap was then washed for 10 min with buffer A, after which the trap was turned in-line with the analytical column (Acclaim PepMap RSLC column, 75 μm
×
15 cm). The analytical solvent system consisted of buffer A and buffer B (98% MeCN, 0.1% FA in ultrapure water) at 300 nl/min flow rate. Peptides were eluted using the followed gradient: linear 2–20% of buffer B over 90 min, linear 20–40% of buffer B for 30 min, linear 40–98% of buffer B for 10 min, isocratic 98% of buffer B for 5 min, linear 98–2% of buffer B for 2.5 min and isocratic 2% buffer B for 12.5 min. The eluent was sprayed with a NANOSpray II source (electrospray ionization) into the TripleTOF 5600+ tandem mass spectrometer (AB Sciex), controlled by Analyst® TF software (AB Sciex). The mass spectrometer was operated in data-dependent acquisition top 20 positive ion mode with 120 ms acquisition time for MS (m/z 400–1250) and 80 ms for tandem mass spectrometry (MS/MS) (m/z 95–1800), and 15 s of dynamic exclusion. MS/MS was conducted with a rolling collision energy (CE) inclusive of present iTRAQ CE adjustments.
The 12 raw mass spectrometry data files were analyzed by ProteinPilot software, version 5.0.1.0 (Applied Biosystems) with the Paragon™ database search and Pro Group™ Algorithm using the UniProtKB/Swiss-Prot FASTA database. The general Paragon search analysis parameters were as follows: type “iTRAQ4plex (Peptide Labeled),” cysteine alkylation “MMTS,” digestion “trypsin” as the cleavage enzyme, instrument “TripleTOF” and species “Mouse” for sample parameters; processing parameters were specified as “quantitative,” “bias correction,” “background correction,” “thorough ID” and “biological modifications.” Proteins that showed a protein threshold of >5 were used for the Pro Group Algorithm to calculate the relative quantification of the protein expression, generating an error factor and P-value. A false discovery rate analysis was performed using the Proteomics System Performance Evaluation Pipeline.
Bioinformatics analysis
The DAVID 6.8 (23, 24) was used for the GO analysis. The analysis was performed separately for upregulated and downregulated proteins and included terms with at least two annotated proteins and P-value of ≤0.05. Differentially expressed proteins were also analyzed using the STRING 10 (25) to identify statistically significant interactions between them. Association network analysis was performed with high confidence (0.700) interaction score for upregulated and with highest confidence (0.900) interaction score for downregulated proteins to exclude false-positive results.
Cell culture
Skin fibroblast cells from the Coriell Cell Repository (Supplementary Material, File S1) were grown in high glucose Dulbecco's modified Eagle medium (DMEM; Gibco) with 10% fetal bovine serum (FBS; Gibco) supplemented with 1% non-essential amino acids (MEM–NEAA; Gibco) and 1% penicillin–streptomycin (PEN–STREP; Lonza). Wild-type and LMNA knockout (KO) mouse embryonic fibroblast cells (MEFs) (32) were grown in DMEM (Gibco) with 15% FBS (Gibco) supplemented with 1% MEM–NEAA (Gibco) and 1% PEN–STREP (Lonza).
HEK293 cells were obtained from European Collection of Authenticated Cell Cultures. Cell culture and knockdown of ubiquitin-like modifier-activating enzyme 1 (UBA1) were performed as previously described (21). Briefly, HEK293 cells were grown in DMEM (Life Technologies) with 10% FBS (Sigma), penicillin/streptavidin (Invitrogen) and L-glutamine (Invitrogen). Cells were transfected with RNAiMax (Invitrogen) and 2.5 μm Silencer Select Validated UBA1 siRNA (s601, targeted against exons 24 and 25; Life Technologies) or 2.5 μm negative control siRNA 2 (Life Technologies) according to the manufacturer's instructions. Control and UBA1 knockdown (UBA1 KD) HEK cells were harvested 48 h after transfection.
Western blotting
Protein extraction, sodium dodecyl sulfate–polyacrylamide gel electrophoresis (SDS–PAGE) and western blotting were performed as previously described (58). Briefly, after SDS–PAGE, a part of the gel was excised and stained with Coomassie blue as an internal loading control for total protein. Proteins from the remaining part of the gel were transferred to nitrocellulose membrane by western blotting overnight. Membranes were blocked with 4% powdered milk and incubated with primary antibodies in dilution buffer (1% FBS, 1% horse serum [HS] 0.1% bovine serum albumin [BSA] in phosphate buffered saline [PBS] with 0.05% Triton X-100) for a maximum of 2 h. Primary antibodies were as follows: Mouse anti-SMN (MANSMA12 2E6 (59); 1:100) and rabbit anti-UBA1 (Novus; NBP2-67816; 1:500-1:1000) antibodies were used for cell extracts and mouse tissues. Mouse anti-lamin AC antibody (MANLAC1 4A7 (60); 1:100) was used for cell extracts, while rabbit anti-lamin AC antibody (Abcam; ab169532; 1:2000) was used for tissue extracts. Following incubation with Horseradish peroxidase (HRP)-labeled rabbit anti-mouse Ig (DAKO, P0260) or HRP-labeled goat anti-rabbit Ig (DAKO, P0488) in dilution buffer at 0.25 ng/ml, membranes were incubated with West Pico or West Femto (Thermo Fisher) and visualized using a Gel Image Documentation system (Bio-Rad). Densitometry measurements of antibody reactive bands were obtained using Fiji software (v1.51) (61) and were normalized to densitometry measurements of the Coomassie-stained gel. For densitometry measurements only, contrast and brightness were adjusted uniformly across the gel and blot to decrease the background and enhance signal detection.
RT-qPCR
Total RNA was extracted using RNeasy Plus Mini kit (Qiagen) and quantified with a NanoDrop ND-1000 spectrophotometer (Thermo Fisher). Absorption ratios (OD260/OD280 nm; OD260/OD230 nm) were used to verify the integrity of the RNA. Total RNA was reverse transcribed using SuperScrip VILO cDNA Synthesis Kit (Invitrogen). Quantitative PCR was performed in QuantStudio 3 Real Time PCR system (Applied Biosystems), using SYBR Green detection system (SYBR Select Master Mix; Applied Biosystems). All PCR reactions were performed in triplicates and contained 3.75 ng of cDNA (fibroblast cells) or 2.8 ng of cDNA (heart tissue), and 300 nM Forward and 300 nM Reverse primers in the final volume of 20 μl. Parallel wells with no cDNA (no-template controls) were run for each gene to control for contamination. RNA polymerase II subunit J (POLR2J) and TATA-box binding protein (TBP) were used as reference genes as they have previously demonstrated stable expression across tissues (62). Relative gene expression was quantified using Pfaffl method (63). The parameters of the reactions were 50°C for 2 min, 95°C for 10 min, and 40 cycles of 95°C for 15 s and 60°C for 1 min. Dissociation curves were obtained for each sample, and PCR products were run on 1% agarose gel for a quality control. Primer sequences for lamin A, POLR2J and TBP are available in the Supplementary Material, File S2. Efficiency of the primer pairs was determined by making serial dilutions of the cDNA, plotting the log values of the cDNA against the cycle threshold values, and using a slope to calculate the efficiency according to the equation: E=
10[−1/slope]
×
100 (63).
Immunohistochemistry
SMA and control mouse hearts were fixed in 4% paraformaldehyde for 4 h, cryopreserved in 30% sucrose solution with 0.1% sodium azide and subsequently embedded in a 1:1 solution of 30% sucrose and optimum cutting temperature compound at −40°C. Ventricular heart sections (7 μm) were air dried for 1 h and subjected to antigen retrieval by submersion for 20 min in 10 mm sodium citrate buffer at 90°C, followed by 30 min cooling remaining in solution. Sections were incubated for 2 h in blocking solution (0.4% BSA, 1% Triton X-100 in 0.1 m PBS) at 4°C and then overnight with rabbit anti-lamin A/C antibody (Novus; NBP2-19324) at 4°C. Slides were washed twice (10 min each in PBT [0.1 m PBS with 0.1% Tween-20]), followed by one 10-minute wash in 0.1 m PBS. Sections were incubated with Cy3 goat anti-rabbit IgG (H+
L) (Thermo Fisher; A10520) for 2 h at 4°C, with successive washes as before. Sections were mounted using MOWIOL media (10% Mowiol (Sigma; 81
381), 20% glycerol, 50% 0.2 m Tris buffer pH 8.5, 3% 1,4-diazobicyclooctance made up in distilled water) containing 4′,6-diamidino-2-phenylindole (DAPI).
For the figure, sections were imaged using Nikon eclipse e400 microscope (20× objective). For the purpose of quantification, sections were imaged using a Zeiss LSM710 inverted confocal microscope (63× objective). Densitometry measurements of lamin A/C staining in the heart ventricles were performed using Fiji software (version 1.51) (61). Images were calibrated to OD as previously described (58), and rectangular selection tool of fixed size was used to measure OD of the ventricle wall. Prior to statistical analysis, the OD of the background was subtracted from the OD measurements of the ventricle wall. Nine sections from each mouse were used in the analysis.
Immunocytochemistry
Fibroblast cells were fixed in acetone–methanol solution (50:50) for 10 min, washed with PBS three times for 5 min and incubated with rabbit anti-UBA1 (Novus; NBP2-67816; 1:100) and mouse anti-lamin A (Santa Cruz; sc-71481; 1:50) in blocking buffer (1% FBS, 1% HS, 0.1% BSA in PBS) for 1 or 2 h. After incubation with goat anti-rabbit IgG Alexa Fluor 488 and anti-mouse IgG Alexa Fluor 546 (Life Technologies; 5 μg/ml) in blocking buffer for 1 h, cells were stained with DAPI (Sigma; 0.4 μg/ml) for 10 min and mounted using Hydromount (National diagnostics). Between each step, cells were washed with PBS three times for 5 min. Images were obtained using Leica SP5 confocal microscope with 63× oil immersion objective.
Immunoprecipitation
Immunoprecipitation was performed as described previously (64). Briefly, anti-mouse Pan Ig-coated magnetic beads (50 μl) (Dynal, Oslo) were washed with 4% BSA in PBS and incubated with an anti-lamin A/C monoclonal antibody (26) (neat, 50 μl) for 1 h at room temperature with gentle rolling. The beads were then washed and incubated for 1 h at room temperature with RIPA extracts of heart tissue from SMA and healthy littermate control mice (15 μl) on a roller. The beads were washed thoroughly with Radioimmunoprecipitation assay buffer (RIPA buffer) before eluting captured material by heating at 90°C for 3 min in 1×
SDS sample buffer (30 μl) (2% sodium dodecyl sulfate, 10% glycerol, 5% 2-mercaptoethanol, 62.5 mm Tris–HCl, pH 6.8). Samples were subjected to western blotting as described above. Primary antibodies were as follows: rabbit anti-lamin AC (Abcam; ab169532; 1:2000), rabbit anti-UBA1 (Novus; NBP2-67816; 1:1000) and mouse anti-β-catenin (BD Transduction Laboratories; 610
154; 1:1000).
Statistical analysis
All statistical analyses were performed in GraphPad Prism version 8.0.1 for Windows, GraphPad Software, San Diego, California, USA, www.graphpad.com. The Shapiro–Wilk test was first used to assess the distribution of the data, followed by unpaired two-tailed t-test for parametric data, and a Mann-Whitney U test for a non-parametric data. All data are presented as mean±
standard deviation. Statistical significance was considered to be P
≤
0.05 for all analyses.
Supplementary Material
Supplementary_Tables_Soltic_et_al_2019_ddz195
Supplementary_File_Soltic_et_al_2019_ddz195
Acknowledgements
The authors would like to thank Professor Colin L. Stewart, Institute of Medical Biology, Singapore, for kindly providing wild-type and LMNA KO mouse embryonic fibroblasts, and Professor Glenn E. Morris for helpful discussions about lamin A and for providing access to laboratory equipment.
Conflict of Interest statement. T.H.G. is a member of SMA-related advisory boards for Roche, Muscular Dystrophy UK and SMA Europe.
Funding
Newlife Charity (SG/15-16/11 to H.F.); Keele University ACORN (to H.F. and D.S.); British Heart Foundation (PG/16/68/31991 to I.H.)]; UK SMA Research Consortium (SMA Trust to T.H.G.); Euan MacDonald Centre for Motor Neurone Disease Research (to H.K.S. and T.H.G.); Wellcome Trust (094476/Z/10/Z to S.L.S.).
References
Articles from Human Molecular Genetics are provided here courtesy of Oxford University Press
Full text links
Read article at publisher's site: https://doi.org/10.1093/hmg/ddz195
Read article for free, from open access legal sources, via Unpaywall:
https://www.ncbi.nlm.nih.gov/pmc/articles/PMC6927462
Citations & impact
Impact metrics
Citations of article over time
Alternative metrics
Smart citations by scite.ai
Explore citation contexts and check if this article has been
supported or disputed.
https://scite.ai/reports/10.1093/hmg/ddz195
Article citations
The SMN-ribosome interplay: a new opportunity for Spinal Muscular Atrophy therapies.
Biochem Soc Trans, 52(1):465-479, 01 Feb 2024
Cited by: 2 articles | PMID: 38391004 | PMCID: PMC10903476
Review Free full text in Europe PMC
Innovating spinal muscular atrophy models in the therapeutic era.
Dis Model Mech, 16(9):dmm050352, 01 Sep 2023
Cited by: 2 articles | PMID: 37787662 | PMCID: PMC10565113
Review Free full text in Europe PMC
AAV9-mediated SMN gene therapy rescues cardiac desmin but not lamin A/C and elastin dysregulation in Smn2B/- spinal muscular atrophy mice.
Hum Mol Genet, 32(20):2950-2965, 01 Oct 2023
Cited by: 1 article | PMID: 37498175 | PMCID: PMC10549791
WNT-β Catenin Signaling as a Potential Therapeutic Target for Neurodegenerative Diseases: Current Status and Future Perspective.
Diseases, 11(3):89, 25 Jun 2023
Cited by: 31 articles | PMID: 37489441 | PMCID: PMC10366863
Review Free full text in Europe PMC
The Proteome Signatures of Fibroblasts from Patients with Severe, Intermediate and Mild Spinal Muscular Atrophy Show Limited Overlap.
Cells, 11(17):2624, 23 Aug 2022
Cited by: 4 articles | PMID: 36078032 | PMCID: PMC9454632
Go to all (8) article citations
Data
Data behind the article
This data has been text mined from the article, or deposited into data resources.
BioStudies: supplemental material and supporting data
Similar Articles
To arrive at the top five similar articles we use a word-weighted algorithm to compare words from the Title and Abstract of each citation.
AAV9-mediated SMN gene therapy rescues cardiac desmin but not lamin A/C and elastin dysregulation in Smn2B/- spinal muscular atrophy mice.
Hum Mol Genet, 32(20):2950-2965, 01 Oct 2023
Cited by: 1 article | PMID: 37498175 | PMCID: PMC10549791
Dysregulation of ubiquitin homeostasis and β-catenin signaling promote spinal muscular atrophy.
J Clin Invest, 124(4):1821-1834, 03 Mar 2014
Cited by: 124 articles | PMID: 24590288 | PMCID: PMC3973095
Dysregulation of Tweak and Fn14 in skeletal muscle of spinal muscular atrophy mice.
Skelet Muscle, 12(1):18, 28 Jul 2022
Cited by: 4 articles | PMID: 35902978 | PMCID: PMC9331072
Molecular mechanisms and animal models of spinal muscular atrophy.
Biochim Biophys Acta, 1852(4):685-692, 01 Aug 2014
Cited by: 31 articles | PMID: 25088406
Review
Funding
Funders who supported this work.
British Heart Foundation (1)
The role of nuclear envelope proteins in cardiac conduction and heart disease
Professor Glenn Morris, Keele University
Grant ID: PG/16/68/31991
Euan MacDonald Centre for Motor Neurone Disease Research
Keele University ACORN
Newlife Charity (1)
Grant ID: SG/15-16/11
UK SMA Research Consortium
Wellcome Trust (1)
Grant ID: 094476/Z/10/Z