Abstract
Free full text
Chromogranin A and its fragments in cardiovascular, immunometabolic, and cancer regulation
Abstract
Chromogranin A (CgA)—the index member of the chromogranin/secretogranin secretory protein family—is ubiquitously distributed in endocrine, neuroendocrine, and immune cells. Elevated levels of CgA‐related polypeptides, consisting of full‐length molecules and fragments, are detected in the blood of patients suffering from neuroendocrine tumors, heart failure, renal failure, hypertension, rheumatoid arthritis, and inflammatory bowel disease. Full‐length CgA and various CgA‐derived peptides, including vasostatin‐1, pancreastatin, catestatin, and serpinin, are expressed at different relative levels in normal and pathological conditions and exert diverse, and sometime opposite, biological functions. For example, CgA is overexpressed in genetic hypertension, whereas catestatin is diminished. In rodents, the administration of catestatin decreases hypertension, cardiac contractility, obesity, atherosclerosis, and inflammation, and it improves insulin sensitivity. By contrast, pancreastatin is elevated in diabetic patients, and the administration of this peptide to obese mice decreases insulin sensitivity and increases inflammation. CgA and the N‐terminal fragment of vasostatin‐1 can enhance the endothelial barrier function, exert antiangiogenic effects, and inhibit tumor growth in animal models, whereas CgA fragments lacking the CgA C‐terminal region promote angiogenesis and tumor growth. Overall, the CgA system, consisting of full‐length CgA and its fragments, is emerging as an important and complex player in cardiovascular, immunometabolic, and cancer regulation.
Abstract
In this review, we focus on how full‐length chromogranin A and its peptides, including PST, vasostatin‐1, CST, and serpinin, as important modulators of cardiovascular functions, immunometabolism, and cancer.
Introduction
In September 1965, Banks and Helle reported the release of proteins from the stimulated adrenal medulla.1 In July 1967, Blaschko's group named splanchnic nerve–stimulated release of soluble proteins “chromogranins;”2 and in September 1967, his group coined the term chromogranin A (CgA) for the major component of these proteins.3 Subsequently, it has been reported that patients suffering from neuroendocrine tumors, heart failure (HF), renal failure, hypertension, rheumatoid arthritis, and inflammatory bowel disease display elevated levels of circulating CgA‐related polypeptides consisting of full‐length molecules and fragments (Table 1). The human chromogranin A gene (CHGA; protein abbreviation hCgA) encodes a 439‐amino‐acid mature protein of approximately 48–52 kDa with a coiled‐coil structure.4, 5, 6, 7, 8 Structurally, hCgA has nine dibasic sites and is proteolytically cleaved by prohormone convertases,9, 10, 11 cathepsin L,12 plasmin,13, 14, 15 and kallikrein16 to generate biologically active peptides, including (1) the dysglycemic peptide pancreastatin (PST: hCgA250–301);17, 18 (2) WE14 (hCgA324–337), which acts as the antigen for highly diabetogenic CD4+ T cell clones;19, 20, 21 (3) the vasodilating, antiadrenergic, and antiangiogenic peptide vasostatin‐1 (hCgA1–76);22, 23, 24, 25, 26 (4) the antiadrenergic, antihypertensive, antibacterial, proangiogenic, antiobesogenic, and antiinflammatory peptide catestatin (CST: hCgA352–372);27, 28, 29, 30, 31, 32, 33, 34, 35, 36, 37, 38, 39, 40, 41 and (5) the proadrenergic peptide serpinin (hCgA411–436).42, 43 Several of these CgA‐derived peptides exhibit opposing (i.e., with regard to each other) regulatory effects. For example, cardiac contractility in rodents is controlled by vasostatin‐1 and CST, which are antiadrenergic,36, 44 as well as by serpinin, which is proadrenergic.43 Likewise, angiogenesis is controlled by vasostatin‐1 functioning in an antiangiogenic manner26, 41 and CST functioning in a proangiogenic manner35, 41 (Fig. 1). CgA gene (Chga) knockout (KO) was generated by targeted deletion of exon 1 and approximately 1.5 kbp of its proximal promoter, resulting in complete elimination of Chga expression. Chga‐KO mice provide an important model to study the roles of individual CgA‐derived peptides through analysis of phenotypes after supplementation.33, 40, 45, 46, 47 Here, we focus on how full‐length CgA and its peptides, including PST, vasostatin‐1, CST, and serpinin, are important modulators of cardiovascular functions, immunometabolism, and cancer.
Table 1
Plasma CgA levels in health and diseases
Diseases/abnormalities | Diseases (nM) | Controls (nM) | References |
---|---|---|---|
Cardiovascular diseases | |||
Controls | 2.64 | O'Connor60 | |
Hypertension (primary) | 4.04 | ||
Hypertension (primary) | 4.05 | O'Connor61 | |
Hypertension (secondary) | 3.93 | ||
Controls | 3.29 | Cryer207 | |
Cardiac arrest | 3.23 | ||
Controls | 1.46 | Ceconi et al.52 | |
CHF NYHA I | 2.25 | ||
CHF NYHA II | 3 | ||
CHF NYHA III | 5.7 | ||
CHF NYHA IV | 11.4 | ||
Controls | 0.49 | Omland et al.53 | |
Myocardial infarction | 0.61 | ||
Controls | 1.31 | Pieroni et al.48 | |
DCM | 3.14 | ||
HCM | 3.07 | ||
Controls | 1.0 | Larsen et al.71 | |
CHF NYHA II and III | 2.1 | ||
Controls | 0.98 | Tombetti208 | |
Takayasu arteritis | 2.36 | ||
Renal diseases | |||
Controls | 0.57 | O'Connor209 | |
Renal failure | 7.99 | ||
Controls | 1.16 | Spadaro210 | |
Chronic renal failure | 28.41 | ||
Liver diseases | |||
Controls | 0.57 | O'Connor209 | |
Liver dysfunction | 2.04 | ||
Controls | 1.16 | Spadaro210 | |
Liver cirrhosis | 5.09 | ||
Lung diseases | |||
Controls | 2.51 | Sobol211 | |
COPD | 3.45 | ||
Inflammatory bowel disease | |||
Controls | 1.16 | Spadaro210 | |
IBD | 4.46 | ||
Rheumatoid arthritis | |||
Controls | 1.13 | Di Comite203 | |
Rheumatoid arthritis | 5.79 | ||
Sepsis | |||
Controls | 4.86 | Hsu212 | |
Severe sepsis | 9.89 | ||
Controls | 1.76 | Zhang213 | |
Severe sepsis | 3.34 | ||
Controls | <4.0 | Rosjo214 | |
Sepsis survivors | 9.1 | ||
Sepsis nonsurvivors | 14.0 | ||
Meniere's disease | |||
Controls | 0.67 | Teggi215 | |
Meniere's disease | 1.46 | ||
Erdheim–Chester disease | |||
Controls | 0.72 | Ferrero216 | |
Erdheim–Chester disease | 3.13 | ||
Tumors/cancer | |||
Controls | 2.64 | O'Connor61 | |
Pheochromocytoma | 32.99 | ||
Controls | 1.17 | O'Connor217 | |
Pheochromocytoma | 15.04 | ||
Carcinoid tumor | 723.68 | ||
Pancreatic islet tumor | 101.39 | ||
Thyroid carcinoma | 11.53 | ||
Parathyroid adenoma | 4.46 | ||
Controls | 2.51 | Sobol211 | |
Small cell lung carcinoma | 16.66 | ||
Large cell lung carcinoma | 3.74 | ||
Adenocarcinoma | 3.68 | ||
Controls | 0.57 | Hsiao218 | |
Pheochromocytoma (familial) | 2.41 | ||
Pheochromocytome (sporadic) | 3.76 | ||
Controls | 3.22 | Cryer207 | |
Pheochromocytoma | 6.81 | ||
Controls | 0.29 | Blind219 | |
Medullary thyroid carcinoma | 16.48 | ||
Controls | 1.16 | Spadaro210 | |
Hepatocellular carcinoma | 15.04 | ||
Controls | 1.19 | Lee et al.220 | |
Pancreatic ductal adenocarcinoma | 2.87 |

Schematic diagram showing the major peptide domains of CgA protein. Relative locations of vasostatin‐1, pancreastatin (PST), catestatin (CST), and serpinin domains along with the first and last amino acids for each peptide domain are shown except for CST, where an arginine at position 373 is shown in addition to the last amino acid. Major functions of each of the peptide are shown above the peptide domain.
Cardiovascular functions
Circulating CgA in patients with cardiovascular diseases
Several studies have addressed the role of circulating CgA as a biomarker in patients with cardiovascular diseases using CgA‐ELISA systems that were unable to discriminate between the full‐length CgA and its fragments. Using these assays, increased levels of immunoreactive CgA–related polypeptides have been detected in the blood of patients with myocardial infarction, HF, decompensated and hypertrophic heart, and acute coronary syndromes, with important prognostic implications in several cases.48, 49, 50, 51, 52, 53, 54, 55 Studies in patients with dilated and hypertrophic cardiomyopathy revealed that plasma CgA (detected by a homemade ELISA that was unable to discriminate the full‐length CgA and its fragments) strongly correlates with circulating B‐type natriuretic peptide (BNP), an important diagnostic and prognostic marker of HF.48 A later study performed in patients with acute destabilized HF showed that CgA adds independent prognostic information to N‐terminal proBNP, another known prognostic marker of the disease, thus showing an improved prognostic ability of the combination of the two markers.56 However, the prognostic value of CgA in acute and chronic myocardial failure is still a matter of debate.57 Indeed, results of a trial conducted on a large group of patients with chronic HF (GISSI, Gruppo Italiano per lo Studio della Sopravvivenza nell'Infarto Miocardico) showed CgA association with all‐cause mortality or cardiovascular morbidity in univariate, but not multivariate, regression analysis adjusted for known risk factors.54 Given the highly heterogeneous nature of the CgA antigen owing to differential post‐translational modifications and proteolytic processing, and considering that CgA and its fragments can work as cardioregulatory hormones in certain cases with opposite functions (discussed below), the detection of specific CgA‐related polypeptides with specific immunoassays is necessary to fully assess the prognostic value of the CgA system in cardiovascular diseases. This concept is well exemplified by the results of a recent study showing that serum levels of the hCgA1–113 fragment (known as vasostatin‐2) are reduced in patients with ischemic chronic HF and are negatively, and independently, associated with major adverse cardiac effects.58 The role of CgA and its fragments on cardiovascular functions will be discussed below.
CgA in hypertension
O'Connor's group was the first to report that circulating levels of CgA are associated with hypertension. In 1984, they reported a normal plasma CgA level of 2.64 nM, which was increased by ~12‐fold (32.99 nM) in pheochromocytoma patients,59 as well as ~1.5‐fold in hypertensive patients (4.04 nM).60 His group also reported that plasma CgA levels are increased in both essential (primary) hypertension (4.05 versus 2.64 nM) and secondary hypertension (3.93 versus 2.64 nM).61 Since blocking sympathetic outflow by guanabenz resulted in reduced plasma CgA, it was suggested that plasma CgA is, at least in part, regulated by sympathetic nerve, and essential hypertension could represent an increased sympathoadrenal activity.61 Subsequently, increased CgA content was reported both in the adrenal gland62, 63 and plasma.63
CgA in HF
Characterized by defective ventricular filling or the ejection of blood, HF is caused by structural and functional defects in myocardium. The major pathogenic mechanisms involved in HF include increased hemodynamic overload, ischemia‐associated dysfunction, ventricular remodeling, abnormal myocyte calcium handling, and excessive neurohumoral stimulation.64 Longstanding hypertension is one of the major causes of HF; and most patients with HF show a history of hypertension.65, 66 Augmented sympathetic nerve activity is also recognized as a major contributor to the complex pathophysiology of HF.67, 68 Since sympathoexcitation has been reported to increase plasma CgA, studies were undertaken to evaluate the possible association of CgA with HF.61, 69 A study was conducted with 103 healthy subjects and 160 consecutive elective patients (83.1% men; 16.9% women) with chronic heart failure (CHF), among which patients were classified in accordance with New York Heart Association (NYHA): NHYA I, NHYA II, NHYA III, and NHYA IV, to find out whether plasma level of CgA is increased in CHF subjects. Because of a nonnormal distribution, only median values were presented, which revealed that the increase in CgA in plasma was positively correlated with the severity of the diseases (normal subjects, 1.46 nM; NYHA I, 2.25 nM; NYHA II, 3 nM; NYHA III, 5.7 nM; and NYHA IV, 11.14 nM).52 Of note, although CgA did not correlate with CHF‐activated hormones, such as catecholamines, vasopressin, endothelins, and components of the renin–angiotensin–aldosterone system, it did correlate with the soluble tumor necrosis factor‐alpha (TNF‐α) receptors and, to a lower extent, with TNF‐α itself.70 Another study of 20 healthy volunteers and 25 men with stable CHF (NYHA II and NYHA III) also showed elevated plasma CgA (2.1 versus 1.0 nM controls);71 this study also confirmed a positive correlation between CgA and TNF‐α, as well as between CgA and N‐terminal atrial natriuretic peptide. Since plasma catecholamine levels were comparable, the results suggested that inflammatory responses contribute more to elevated plasma CgA in CHF than to activation of the sympathoadrenergic system.
To find out whether plasma CgA could serve as a prognostic marker for myocardial infarction, Omland's group measured plasma CgA in 119 patients (88 men; 31 women) on day 3 after the onset of myocardial infarction.53 They found significantly higher plasma CgA levels in patients with symptoms and signs of HF (0.61 versus 0.49 nM); the mortality rate was significantly higher in patients with plasma CgA of >0.49 nM. They concluded that plasma CgA levels are associated with long‐term mortality after myocardial infarction.53 In a subsequent study in 217 patients with acute myocardial infarction complicated with HF or ventricular dysfunction, plasma CgA emerged as a strong and independent predictor of death and hospitalization.49 In another study in 137 patients (94% men; 6% women) admitted to a single center with acute decompensated HF, CgA was found to exhibit comparable prognostic accuracy to N‐terminal probrain natriuretic peptide (NT‐proBNP) and provided further prognostic information after adjustment for other risk factors, including estimated glomerular filtration rate and NT‐proBNP.56
One of the largest studies evaluating the prognostic value of CgA in 1233 chronic HF patients (GISSI: 86.4% men and 13.6% women) found that plasma CgA concentrations are increased in proportion to disease severity.54 The estimated probability of death was calculated to be the highest with CgA concentrations >33 U/L and lowest with CgA concentrations <16 U/L. Univariate analyses found a correlation between baseline CgA levels with the two coprimary outcome measures in GISSI‐HF: mortality and mortality of hospitalization for cardiovascular disease during follow‐up. The study found significant correlations between plasma CgA levels and BNP and hs‐C‐reactive protein levels. However, multivariate analyses did not find significant association between elevated CgA levels and mortality or CgA levels and either mortality or cardiovascular morbidity during follow up. Thus, by these data, it was concluded that plasma CgA levels in patients with chronic, stable HF do not provide incremental prognostic information over that obtained from physical examination, routine biochemical analysis, and contemporary HF biomarkers.54 By contrast, the larger study of acute coronary syndromes in a single coronary care unit of a Scandinavian teaching hospital (1268 patients; 70% men and 30% women) found CgA measured on day 1 to be an independent predictor of long‐term mortality and HF hospitalizations, as well as providing incremental prognostic information above evaluation of conventional cardiovascular risk factors.50 Of note, recurrent myocardial infarction was found not to be associated with plasma CgA levels after adjustments for conventional cardiovascular risk markers.50
Full‐length CgA and vasostatins
A growing body of evidence suggests that full‐length CgA (hCgA1–439) and its N‐terminal fragments hCgA1–76 and hCgA1–113 are important players in the regulation of the cardiovascular system.72, 73 These fragments were named vasostatin‐1 and vasostatin‐2, respectively, because of their ability to suppress vasoconstriction in isolated human conduit vessels, originally observed.22, 74, 75, 76 After their discovery, a wide range of other biological effects were assigned to these polypeptides. For example, vasostatin‐1 has been implicated in the homeostatic regulation of plasma calcium, vascular tension, endothelial barrier integrity, innate immunity, inflammation, pain relief, intestinal motility, intestinal barrier, and cardiac functions.55, 74, 76, 77, 78 Full‐length CgA and vasostatin‐1 can modulate the adhesion of cardiomyocytes, smooth muscle cells, and fibroblasts, and to proteins involved in the extracellular matrix and endothelial cell–cell adhesion.74 Interestingly, in certain cases, full‐length CgA and fragments may exert opposite effects; for example, while vasostatin‐1 can enhance the adhesion of fibroblasts to extracellular matrix proteins, full‐length CgA inhibits cell adhesion.25
Studies performed with isolated and perfused rat hearts have shown that recombinant hCgA1–439 and vasostatin‐1 can depress myocardial contractility and relaxation, counteract the β‐adrenergic‐induced positive inotropism, and modulate coronary tone mainly via a nitric oxide–dependent mechanism.72, 73 Thus, these polypeptides can work as cardioregulatory hormones.
Vasostatin‐2, which includes the vasostatin‐1 (hCgA1–76) and the vasoconstrictive‐inhibitory factor sequence (hCgA79–113, known to act as a vasoregulatory molecule when liberated by cleavage79), increases coronary pressure in Langendorff‐perfused rat hearts without affecting inotropism, but can counteract the cardiostimulatory effects of isoproterenol.44 Using a rat model of myocardial infarction, other investigators have shown that vasostatin‐2 can improve cardiac function and reduce remodeling, fibrosis, and inflammation, suggesting that the reduced levels of this fragment observed in patients with ischemic CHF may have a pathogenic role.58
CgA and vasostatin‐1 can exert cardioprotective effects against ischemia/reperfusion (I/R) injury.72, 73 In this setting, vasostatin‐1 can act as a preconditioning inducer.80 Remarkably, hemodynamic and excitatory stimulation of rat hearts induce intracardiac CgA proteolytic processing to generate fragments containing the homologous vasostatin‐1 sequence.81 A recent study has shown that full‐length human CgA and vasostatin‐1 can also prevent cardiotoxic events induced by doxorubicin, a widely used anticancer drug whose clinical application is hampered by dose‐limiting cardiotoxicity.82 In particular, experimental evidence obtained in a rat model showed that low‐dose hCgA1–439 can protect the heart from inflammation, apoptosis, fibrosis, and ischemic injury caused by doxorubicin.82 Notably, this study also showed that pharmacologically active doses of hCgA1–439 do not impair the antitumor activity of doxorubicin in various murine models of solid tumors. Doxorubicin could also reduce both intracardiac expression and plasma levels of CgA.82 It appears, therefore, that doxorubicin can reduce the levels of this endogenous cardioprotective agent and that systemic administration of exogenous hCgA1–439 can restore cardioprotection. Interestingly, exogenous vasostatin‐1 could also exert cardioprotective effects on doxorubicin‐induced cardiotoxicity in the same rat model.82 However, in this case, a higher dose, compared with that necessary for hCgA1–439, was required to achieve cardioprotection, suggesting that the full‐length molecule is more potent. Monitoring CgA and vasostatin‐1 levels in cancer patients before and after doxorubicin therapy, the administration of low‐dose full‐length CgA to patients with low CgA levels might represent a novel approach to prevent doxorubicin‐induced cardiotoxicity, thereby meriting further investigation.
Catestatin
Although initially identified as a physiological brake on catecholamine secretion,27, 28, 29, 30, 31, 32 CST is now established as a pleotropic hormone that reduces blood pressure,33, 83, 84, 85, 86, 87 positively regulates baroreflex sensitivity (BRS)46 and heart rate variability (HRV),88 and provides cardioprotection.36, 39, 89, 90, 91
Vasodilatory and hypotensive effects of CST
The hypotensive effects of CST (bovine CgA344–364) were first identified in pithed rats.83 Intravenous injection of bCST resulted in the reduction of pressor responses to the activation of sympathetic outflow by electrical stimulation. bCST also blunted neuropeptide Y agonist–induced hypertension. The vasodepressor effects of bCST appeared to be mediated by massive release of histamine (21‐fold increase) from mast cells, which is consistent with the vasodilatory effects of histamine.92 Using primary pleural and peritoneal cell populations containing 5% of mast cells, it was reported that bCST acts directly on mast cells to release histamine. The potency and efficacy of bCST were better evaluated when compared with the wasp venom peptide mastoparan.93 In human mast cell line LAD2, hCST induced migration of peripheral blood–derived mast cells to migrate, and caused degranulation and release of leukotriene C4 and prostaglandins D2 and E2;94 hCST also caused increased intracellular Ca2+ mobilization and induced the production of proinflammatory cytokines/chemokines, such as granulocyte‐macrophage colony–stimulating factor, chemokine C‐C motif ligand 2 (CCL2, also known as MCP1), chemokine C‐C motif ligand 3 (CCL3, also known as MIP1A), and chemokine C‐C motif ligand 4 (CCL4, also known as MIP1B).94 The vasodilatory effect of bCST was subsequently tested on human dorsal hand vein (to avoid systemic counterregulation) using hCST after pharmacologic vasoconstriction with phenylephrine, which showed dose‐dependent vasodilation, especially in female subjects;86 histamine‐induced vasodilation is believed to be mediated through increased production of nitric oxide. In rodents, the hypotensive effects of hCST were reported both in monogenic (hypertensive and hyperadrenergic Chga‐KO mice) and polygenic (blood pressure high mice) models of hypertension. In the monogenic model, hCST exerted its hypotensive effects by inhibiting the secretion of catecholamines33 (Fig. 2).
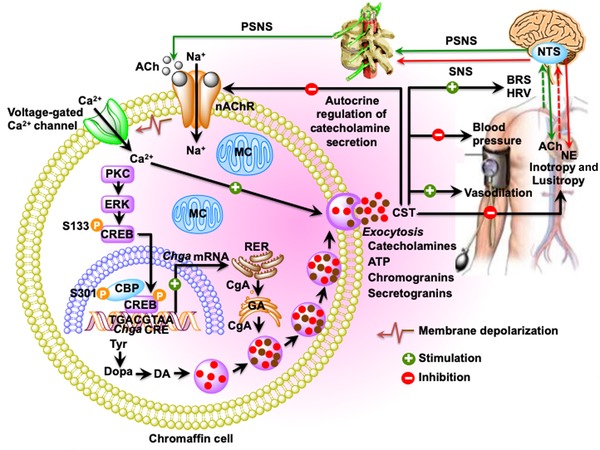
Schematic diagram showing the inhibition of catecholamine secretion and regulation of cardiovascular functions by CST. Acetylcholine released from preganglionic sympathetic endings at the splanchnic–adrenal synapse binds to nicotinic acetylcholine receptors and induces influx of Na+ inside chromaffin cells resulting in the depolarization of the cell membrane and opening of voltage‐gated Ca2+ channels. Increased cytosolic Ca2+ concentration stimulates the transcription of Chga and induces exocytotic release of the secretory cocktail containing catecholamines, CST, ATP, chromogranins, and neuropeptides. Exocytotically released CST inhibits further release of catecholamines by an autocrine/paracrine mechanism; decreases blood pressure and improves BRS and HRV by inhibiting catecholamine secretion; causes vasodilation by stimulating massive release of histamine; and decreases inotropy and lusitropy by activating the β2‐AR‐Gi/o‐protein–PI‐3K–AKT–NO–cGMP signaling pathway. Ach, acetylcholine; BRS, baroreflex sensitivity; CA, catecholamine; CBP, CREB binding protein; CgA, chromogranin A protein; CREB, cAMP‐responsive element–binding protein; DA, dopamine; ERK, extracellular signal–regulated kinase; GA, Golgi apparatus; HRV, heart rate variability; MC, mitochondria; NE, norepinephrine; NTS, nucleus tractus solitarius; PKC, protein kinase C; PSNS, peripheral sympathetic nervous system; RER, rough endoplasmic reticulum; SNS, sympathetic nervous system; Tyr, tyrosine.
Effects of CST on BRS and HRV
Consistent with diminished BRS in hypertensive subjects, hypertensive Chga‐KO mice also display diminished BRS.46 Intravenous administration of hCST increased BRS in hypertensive Chga‐KO mice, where both the reflex bradycardia caused by phenylephrine‐induced hypertension and the reflex tachycardia caused by sodium nitroprusside–induced hypotension were abolished or attenuated.46 These findings indicate that hCST resets the entire autonomic nervous reflex arc to restore normal cardiovascular function.46 Like BRS, hypertensive subjects display decreased HRV; and hypertensive Chga‐KO mice also exhibit decreased HRV.88 Intraperitoneal administration of hCST decreased total power (TP: ms2), which comprises low‐frequency power (LF: 0.4–1.5 Hz), high‐frequency power (HF: 1.5–4.0 Hz), and frequencies outside of low LF and HF power components, in the frequency domain of HRV spectra. hCST also improved each of the time domain parameters, such as mean difference in R–R between consecutive beats in ms (N–N), standard deviation of N–N in ms (SDNN), coefficient of variation of N–N (CVNN), % of consecutive beats more than 7 ms different in R–R (NN7%), reflecting cardiac parasympathetic tone, and root mean square of standard deviation of N–N in ms (RMSSD), which were decreased in Chga‐KO mice.88 Thus, hCST improves both BRS and HRV in Chga‐KO mice, which represents a monogenic model of rodent hypertension (Fig. 2).
Central effects of CST
CgA is ubiquitously expressed in mouse,30, 95 rat,96, 97, 98 and sheep99 central nervous systems. More precisely, CgA is expressed in the cell bodies of most anatomically defined inhibitory, excitatory, and aminergic cell groups of the brain and spinal cord. Barosensitive, bulbospinal neurons in the rostral ventrolateral medulla (RVLM) provide the major tonic excitatory drive to the sympathetic neurons that maintain arterial pressure.100 Microinjection of a pharmacological dose of CST (rat CgA367–387; 50 nL, 1 mM dissolved in PBS) into the RVLM of vagotomized normotensive rat resulted in sympathoactivation of up to 22% with an increase in arterial blood pressure of up to 35 mmHg. rCST also increased sympathetic BRS with concomitant attenuation of somatosympathetic reflex, implicating rCST as a major player in the regulation of adaptive reflexes. The caudal ventrolateral medulla (CVLM), residing caudal to the RVLM, is the major source of the tonic GABAergic inhibition of presympathetic RVLM neurons. CVLM neurons inhibit immediate changes in blood pressure via the baroreflex and tonically blunt the activity of the presympathetic RVLM neurons by baroreceptor‐independent mechanisms. The later effects play an important role in determining the long‐term level of sympathetic vasomotor tone and blood pressure.100 Microinjection of rCST into the CVLM neurons of vagotomized normotensive rats caused excitation of CVLM neurons resulting in decreased mean arterial blood pressure (by ~23%) and attenuation of sympathetic baroreflex (by ~64%).101 By contrast, in spontaneously hypertensive (SHR) rats, infusion of hCST into the pyramidal neurons of the central amygdala exerted profound decrease in arterial blood pressure (by 65 mmHg).102 Like CVLM, central amygdaloid neurons are also GABAergic, send projections to RVLM, and inhibit excitatory outputs from RVLM with consequent decreases in arterial blood pressure. It thus appears that centrally CST acts as an excitatory peptide as opposed to its inhibitory action in the periphery.
The role of CST in cardioprotection
The first indication that CST acts as a cardioprotective peptide came from a Langendorff‐perfused rat heart preparation study, where hCST inhibited both inotropy and lusitropy under basal and stimulated (β‐adrenergic and endothelin) conditions.36 These cardioprotective effects were shown to be mediated by the activation of the β2‐AR‐Gi/o protein–NO–cGMP pathways. In rat papillary muscles and isolated cardiomyocytes, hCST exerted antiadrenergic effects through the endothelial PI3K–AKT–eNOS pathway.103 It was later shown that hCST provides cardioprotection by stimulating phosphodiesterase type‐2 and NO‐dependent S‐nitrosylation.39 SHR rats mimic human CHF and display decreased Frank–Starling response.104 In both normotensive Wistar–Kyoto (WKY) and SHR rats, hCST caused a significant increase of the Frank–Starling response as evidenced by hCST‐induced increase in left ventricular pressure (LVP) and the maximal rate of LV contraction (LV dP/dt max) with greater effects in SHR rats. hCST also resulted in decreased myocardial relaxation of both WKY and SHR rats as evidenced by CST‐induced decrease in the maximal rate of LV relaxation (LV dP/dt min). A positive correlation was reported between hCST‐induced improvement in myocardial mechanical response and protein S‐nitrosylation. Thus, aged HF patients suffering from ventricular dilation or diminished autonomic heart modulation coupled with increased afterload and impaired Frank–Starling response could receive benefits from hCST.
hCST decreased I/R‐induced infarct size by ~41%, which is comparable to the cardioprotection (~44% decrease in infarct size) achieved through a postconditioning mechanism.105 Although data for attenuated I/R injury for hCST may seem promising, the data are based on experimental animal models that may not reflect human disease and previous clinical studies in the field have all had negative outcomes. Exogenous hCST (75 nM) at the onset of reperfusion resulted in attenuated postischemic rise of diastolic LVP (an index of contracture) and improved postischemic recovery of developed LVP. In addition, in isolated cardiomyocytes, hCST caused profound increase (by 65%) in cell viability after simulated I/R.105 Subsequently, it was shown that cardioprotection by hCST in early reperfusion stage was achieved by the activation of mitochondrial ATP‐sensitive K+ (mitoKATP) channels, ROS signaling, and the prevention of mitochondrial permeability transition pore opening via upstream PI‐3K–AKT and PKC signaling106 (Fig. 3). In isolated rat cardiomyocytes undergoing simulated I/R, hCST increased cell survival 65% and decreased cell contracture of I/R cardiomyocytes. The maintenance of mitochondrial membrane potential in I/R cardiomyocytes and increased phosphorylation of AKT at S473, GSK3β at S9, PLB at T17, and eNOS at S1179 have been shown to play important roles in cardioprotection induced by hCST107 (Fig. 3).
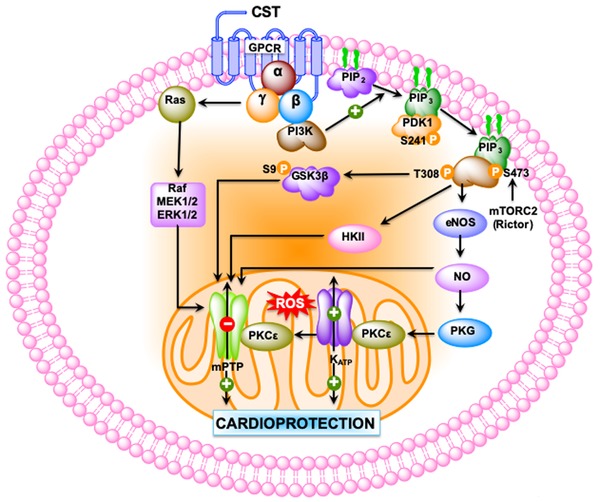
Schematic diagram showing the signaling pathways used by CST to provide cardioprotection. CST‐induced cardioprotection begins by the activation of G protein–coupled or cytokine receptors and the consequent recruitment of signaling pathways: (1) the reperfusion injury salvage kinase (RISK) pathway, including PI‐3K–AKT, ERK1/2, and the downstream target glycogen synthase kinase 3 beta (GSK‐3β); and (2) the PKG pathways. These salvage pathways activate downstream mediators, such as endothelial nitric oxide synthase (eNOS), GSK‐3β, hexokinase II (HKII), protein kinase C‐epsilon (PKCε), the mitochondrial ATP–dependent potassium channel (KATP) with consequent inhibition of mitochondrial permeability transition pore (MPTP). PDK1, pyruvate dehydrogenase kinase 1; ROS, reactive oxygen species.
Effects of human variants of CST on rodent cardioprotection
Five single nucleotide polymorphisms have been discovered in the CST expressing region of CHGA in different human populations, namely, Gly364Ser (rs9658667), Pro370Leu (rs965868), Arg374Gln (rs9658669),32 Tyr363Tyr (rs9658666), and Gly367Val (rs200576557).108, 109 The cardiotropic actions of WT‐CST, Gly364Ser‐CST, and Pro370Leu‐CST were tested in isolated, Langendorff‐perfused rat heart preparation, demonstrating a dose‐dependent (11–200 nM) decrease in LVP (index of contractile activity), rate pressure product (index of cardiac work), and both positive and negative LV dP/dt (index of maximal rate of left ventricular contraction and relaxation, respectively), and increased coronary pressure by WT‐CST. Gly364Ser‐CST was ineffective on basal cardiac performance, and Pro370Leu‐CST induced only negative inotropic activity. Human CST variants also differed in the potencies with which they counteracted the positive inotropic and lusitropic effects of β‐adrenergic stimulation by ISO, with WT‐CST > Gly364Ser‐CST > Pro370Leu‐CST (against ISO‐induced positive inotropism) and Gly364Ser‐CST > WT‐CST > Pro370Leu‐CST (against ISO‐induced positive lusitropism).36
Effects of Gly364Ser‐CST in BRS in humans
In humans, CST 364Ser variant caused profound changes in parasympathetic and sympathetic activity in individuals harboring the variant allele, which include an ~47% and ~44% increase in baroreceptor slope during upward and downward deflections, respectively, an ~2.4‐fold increase in cardiac parasympathetic index, and an ~26% decrease in cardiac sympathetic index in comparison with wild‐type individuals.110 It has been proposed that heightened baroreflex control of circulation may increase the longevity of 364S carriers. However, in response to cold stress, the blood pressure response was significantly less in the Gly/Ser heterozygotes when compared with the WT homozygotes.110
Serpinin
In Langendorff‐perfused rat heart and papillary muscle preparations, both serpinin and pGlu‐serpinin cause dose‐dependent (11–165 nM) increase in cardiac contractility and relaxation, with pGlu‐serpinin being most potent and involving the β1 adrenoceptor–adenylate cyclase–cAMP–PKA pathway.43 This β1‐adrenergic agonist‐like activity of serpinin is in sharp contrast with the β2‐adrenoceptor depressive effects of vasostatin‐1 and CST. It thus appears that serpinin is either mimicking intracardiac sympathetic neurotransmitters and/or circulating catecholamines.43 Like CST, pGlu‐serpinin also provides cardioprotection in both pre‐ and postconditioning mechanisms in WKY and SHR rats. While in the preconditioning mechanism, pGlu‐serpinin caused ~50% decrease in I/R‐induced infract size, it caused an ~33% decrease in infarct size in a postconditioning mechanism of cardioprotection.111 How CST (β1‐adrenergic antagonist) and pGlu‐serpinin (β1‐adrenergic agonist) exerted comparable effects on cardioprotection by a postconditioning mechanism is yet to be addressed.
Immunometabolic functions of catestatin
Effects of CST on metabolism
The role of CST on metabolism came from a study in Chga‐KO mice, which are deficient in CST and PST and display obesity on a normal chow diet.40 Chronic hCST treatment was shown to decrease plasma triglyceride levels in Chga‐KO mice, which is believed to be due to increased lipolysis as evident from increased plasma glycerol and nonesterified fatty acids.40 This effect was mediated through inhibition of α2‐adrenergic receptor (α2‐AR) signaling. In Chga‐KO mice, hCST showed tissue‐specific effects on [14C]‐palmitate incorporation into lipids: decreased incorporation in adipose tissue, but increased incorporation in liver. By contrast, hCST‐induced oxidation of palmitate was comparable between adipose tissue and liver.40 Based on the above findings, it was concluded that hCST inhibits the expansion of adipose tissue and promotes fatty acid uptake in liver for β‐oxidation. Although hCST augmented expression of fatty acid oxidation genes, including carnitine palmitoyltransferase 1α (Cpt1a), peroxisome proliferator–activated receptor‐α (Ppara), acyl‐CoA oxidase 1 (Acox1), and uncoupling protein 2 (Ucp2), it exerted no effect on the expression of lipogenic genes, such as sterol regulatory element‐binding protein 1 (Srebp1) and peroxisome proliferator–activated receptor‐γ (Pparg).40 In view of the above findings, it was concluded that hCST stimulates the incorporation of fatty acids into triglycerides, but not de novo lipogenesis.40 It thus appears that hCST mobilizes fat from adipose to liver for β‐oxidation. In diet‐induced obese and insulin‐resistant mice, hCST caused substantial decreases in hepatic triglyceride, NEFA, and ceramides concomitant with decreases in plasma TG and NEFA levels.112
Effects on CST on immunometabolism
The term immunometabolism was coined in 2011 to describe the multifaceted interactions between the metabolic and immune systems.113 Both the innate (macrophages, eosinophils, neutrophils, and innate lymphoid type 1 and 2 cells)114, 115, 116, 117, 118, 119, 120, 121, 122, 123, 124 and adaptive (CD8+ T cells, CD3+CD4+ T helper type 1 and type 2 cells, CD3+CD4+FOXP3+ T regulatory cells, invariant natural killer T cells, and B lymphocytes)125, 126, 127, 128, 129, 130, 131, 132 immune cells control metabolism. The above studies were conducted in adipose tissues. The liver not only integrates nutrient, hormonal, and environmental signals to regulate glucose and lipid metabolism, but it also plays a key role as part of the immune system. It appears from the literature that cytokines, galectin‐3, and eicosanoids (LTB4) released from immune cells affect hepatocyte insulin signaling resulting in insulin resistance.133, 134, 135 TNF‐α, an inflammatory cytokine released from immune cells in the liver, potentiates steatosis by augmenting lipogenic activity and increasing biosynthesis of ceramides in liver.136 Increased intrahepatocyte ceramide levels in turn cause insulin resistance by inhibiting AKT signaling pathways.137 An intrahepatocyte proinflammatory program has also been shown to promote insulin resistance.138, 139 The first report on the role of CST on immunometabolism came from a recent study in diet‐induced obesity (DIO) and CST gene‐KO mice, where hCST was shown to improve insulin sensitivity by decreasing intrahepatic ceramide levels and inhibiting infiltration of monocyte‐derived macrophages (Ly6C+ proinflammatory macrophages) in liver. Chronic treatment of DIO mice with hCST resulted not only in the reduction of expression of proinflammatory genes (Tnfa, Adgre1 (F4/80), Itgam, Itgax, Ifng, Nos2, and Ccl2), but also increased the expression of anti‐inflammatory genes (Il10, MglI, Il4, Arg1, and Mrc1) in both Kupffer cells (resident macrophages) and recruited monocyte–derived macrophages (LY6C+ macs) (Fig. 4). In addition, hCST caused significant reduction in proinflammatory cytokines, including IL‐1β and TNF‐α in plasma of DIO mice112 (Fig. 4). In human monocyte–derived THP‐1 cells, hCST also increased the expression of the anti‐inflammatory gene Mrc1.140

Schematic diagram showing the putative signaling pathways for the anti‐inflammatory actions of CST. Since CST decreases plasma TNF‐α levels, it is expected that less TNF‐α will be available for binding to its receptor (TNFR1), resulting in the attenuation of the IκB–NF‐κB signaling pathway leading to the translocation of fewer NF‐κB to the nucleus. This reduced NF‐κB signaling will affect the formation of NLRP3 inflammasome resulting in less cleavage procaspase 1 to active caspase 1 leading to less conversion of pro–IL‐1β and pro–IL‐18 to IL‐1β and IL‐18 and secretion of IL‐1β and IL‐18.
Immunometabolic functions of PST
Effects of PST on glucose‐stimulated insulin secretion
PST has been reported to inhibit glucose‐stimulated insulin secretion (GSIS) in vivo in mice, rats, dogs, and pigs, as well as in vitro from isolated rat islets.141 In the perfused rat pancreas model, PST (porcine CgA240–288) was shown to inhibit unstimulated and stimulated insulin secretion.142, 143, 144, 145 Consistent with the inhibitory role of PST in GSIS, GSIS was found to be ~1.7‐fold higher in PST‐deficient Chga‐KO mice at 7 and 15 min after the administration of glucose.45 Although PST is reported to inhibit glucagon secretion induced by low glucose,146 it had no effect on somatostatin secretion.147 Besides the inhibition of GSIS, PST (hCgA273–301) also inhibits insulin‐stimulated glucose transport in primary rat and mouse adipocytes,45, 148, 149 differentiated 3T3‐L1 adipocytes,150, 151 and primary hepatocytes.45, 152
Effects of PST on hepatic glucose metabolism
pPST has been shown to inhibit insulin‐stimulated glycogen synthesis in primary hepatocytes153 and activates glycogenolysis in the rat liver, which implicates a direct anti‐insulin effect on liver metabolism.154, 155 pPST decreases the phosphorylation of insulin receptor substrate 2 at tyrosine residues through the activation of conventional PKC, resulting in the activation of gluconeogenesis. Consistent with the anti‐insulin action, hPST also increases the production of NO with subsequent attenuated phosphorylation of protein kinase B (AKT), forkhead box protein O1 (FOXO1), and reduced matured sterol regulatory element‐binding transcription factor 1c (SREBP1c),45 thereby promoting gluconeogenesis (Fig. 5).

Schematic diagram showing the PST inhibition of gluconeogenesis in hepatocytes. PST initiates a GTP‐binding protein–linked signaling cascade, which results in the activation of diacylglycerol (DAG) and calcium‐dependent conventional PKC (cPKC), thereby attenuating IRS–PI3K–PDK1–AKT signaling pathway. In addition, the stimulation of the cGMP–NOS pathway also dampens this signaling pathway by nitrosylation of IRS. Suppression of this pathway by PST allows FoxO1 and SREBP1c to stimulate the expression of gluconeogenic genes, Pck1 (also known as Pepck) and G6pc (also known as G6Pase), and thus prevents insulin action.
Effects of PST on lipid metabolism
In addition to pronounced effects on glucose metabolism, PST also modulates lipid metabolism. PST (rat CgA263–314) decreases insulin‐stimulated synthesis of lipids in rat adipocytes.156 This finding is consistent with the hPST‐dependent increased expression of hepatic lipogenic genes in Chga‐KO mice, including Srebp1c, peroxisome proliferator‐activated receptor‐gamma (Pparg), and glycerol‐3‐phosphate acetyltransferase (Gpat).45 rPST has also been shown to stimulate the release of glycerol and free fatty acids from rat adipocytes, which is completely inhibited by insulin.156 In humans, hPST augments free fatty acid efflux into the circulation, resulting in an overall spillover of ~4.5‐fold, which is consistent with the reported lipolytic action of rPST,156 confirming the anti‐insulin effects of PST.
Effects of PST on inflammation and insulin resistance
The hallmarks of insulin resistance in DIO mice include obesity, hyperinsulinemia, and increased inflammation.133, 157, 158, 159, 160, 161 Therefore, the suppression of inflammation in DIO mice has been shown to improve insulin sensitivity.162, 163, 164 hPST treatment of Chga‐KO‐DIO mice resulted in increased expression of the proinflammatory genes, including interleukin‐1‐beta (Il1b), TNF‐α (Tnfa), interleukin‐6 (Il6), chemokine C‐C motif ligand 2 (Ccl2), and nitric oxide synthase 2a (Nos2), thereby underscoring the proinflammatory nature of PST. Furthermore, the treatment of Chga‐KO–DIO mice with hPST caused significant reduction in the expression of Arg1 and Il10. Consistent with gene expression data, Chga‐KO–DIO mice show decreased plasma levels of IL‐12p70, IFN‐γ, and chemokine C‐C motif ligand 3‐like 1 (CCL3L1), IL‐6, and chemokine C‐X‐C motif ligand 1 (CXCL1) compared with WT‐DIO mice.
Regulation of angiogenesis and tumor growth
Circulating CgA in patients with neuroendocrine and nonneuroendocrine tumors
Experimental evidence suggests that physiological levels of circulating CgA and its fragments contribute to regulate the vascular physiology in normal and neoplastic tissues.165 CgA is exocytotically released in circulation by the neuroendocrine system to reach, in healthy subjects, about 0.2–1 nM levels.165 This fact implies that tumors with mature and functional blood vessels, either neuroendocrine or nonneuroendocrine, are exposed to physiological amounts of blood‐borne CgA mainly derived from the diffuse neuroendocrine system. Furthermore, although many patients show normal levels of plasma CgA, some patients may have higher levels for a variety of reasons. For example, patients treated with proton pump inhibitors—a class of drugs known to induce gastric enterochromaffin‐like cell hyperplasia and CgA production—may have increased levels of circulating CgA.166, 167, 168, 169 Increased levels of immunoreactive CgA have been also detected in the blood of patients with renal failure, HF, hypertension, atrophic gastritis, inflammatory bowel disease, rheumatoid arthritis, sepsis, and other inflammatory diseases.55 Thus, in patients with nonneuroendocrine neoplasms, the tumor vasculature is exposed to variable levels of CgA derived from the diffuse neuroendocrine system.
The situation is even more complex in the case of neuroendocrine tumors or tumors with neuroendocrine differentiation, as in both these cases the tumor vasculature can be exposed not only to CgA molecules released by neuroendocrine system into the blood, but also to CgA released in tumor microenvironments (and then into the blood) by neoplastic cells. Indeed, cancer cells in neuroendocrine tumors (including pheochromocytomas, carcinoid tumors of the intestine, lung and stomach, parathyroid and medullary thyroid carcinomas, neural tumors, anterior pituitary tumors, small cell lung cancer, pancreaticoduodenal tumors, and many others) can express and secrete CgA in relatively large amounts.167 Also, tumors presenting the focal expression of CgA consequent to neuroendocrine differentiation (such as breast and prostate cancer, non‐small cell lung cancer, and gastric and colonic adenocarcinomas) may also release CgA.7, 167 Thus, the tumor vasculature, in these cases, might be affected by CgA present in the blood and by tumor‐derived CgA in very complex ways.
In this regard, it is important to highlight the fact that detection of full‐length CgA and fragments using specific ELISA systems based on selective antibodies has shown that CgA, either systemically or locally released, consists of variable mixtures of intact protein and fragments, including full‐length CgA (hCgA1–439), the vasostatin‐1 fragment hCgA1–76, fragments lacking part or the entire C‐terminal region, such as hCgA1–373, and other shorter peptides.41, 170 For example, hCgA1–373, which is either not or minimally present in the blood of healthy subjects, is increased in peripheral blood and bone marrow plasma of patients with multiple myeloma, while full‐length CgA is decreased in these patients.170 A central issue is whether changes in the circulating levels of full‐length CgA and its fragments (absolute or relative), which may occur in cancer patients, are epiphenomena of the presence of the tumors or are contribute to tumor pathophysiology. In the following sections, we will discuss the experimental evidence suggesting that CgA and its fragments contribute to regulate tumor growth and responses to therapy.
Role of full‐length CgA and vasostatin‐1 in tumor angiogenesis and growth
It is well known that the unbalanced production of anti‐ and proangiogenic factors in tumors can lead to vascular abnormalities that contribute to tumor cell proliferation, invasion, trafficking, and metastasis formation.171, 172, 173 CgA may be one of the factors that contributes to regulate tumor vascular biology.76, 165 For example, recent studies have shown that full‐length CgA (hCgA1–439) can function as an antiangiogenic factor, with a functional antiangiogenic site in the C‐terminal region 410–439 and a latent (or less active) site in the N‐terminal region 1–76 (vasostatin‐1), which requires proteolytic cleavage for full activation.41 Full‐length CgA and vasostatin‐1 can inhibit endothelial cell migration, motility, invasion, and capillary‐like structure formation induced by vascular endothelial growth factor (VEGF) or fibroblast growth factor‐2 (FGF2), two important proangiogenic factors.41, 174 Vasostatin‐1 can also inhibit the nuclear translocation of hypoxia inducible factor‐1α, a master regulator of angiogenesis, in endothelial cells.175 These proteins can enhance the endothelial barrier function and reduce vascular leakage and trafficking of tumor cells through the endothelium.176, 177
Studies performed in mice have shown that endogenous murine CgA or exogenous recombinant hCgA1–439, but not the fragments lacking the C‐terminal region, can inhibit tumor growth in models of nonneuroendocrine solid‐tumors, including fibrosarcoma, mammary adenocarcinoma, Lewis lung carcinoma, and primary and metastatic melanoma.178 The inhibition of tumor growth is associated with reduction of microvessel density and blood flow in neoplastic tissues. Also, low‐dose vasostatin‐1 can delay tumor growth and reduce microvessel density in murine models of solid tumors.41 In these models, neutralization of endogenous murine CgA with antibodies against its C‐terminal region enhances tumor growth rate, whereas systemic administration of low amounts of hCgA1–439 reduces tumor growth.178 It appears, therefore, that circulating full‐length CgA and vasostatin‐1 can work as physiological inhibitors of tumor growth.
Mechanistically, full‐length CgA induces the production of protease nexin‐1 in endothelial cells, a serine protease inhibitor (serpin) that can inhibit endothelial cell migration, capillary tube formation, and angiogenesis, independently of its serpin activity179, 180 Given that the neutralization of protease nexin‐1 with specific antibodies can suppress the antiangiogenic and antitumor effects of recombinant hCgA1–439, it is possible that the induction of protease nexin‐1 by CgA, as observed in animal models, is an important mechanism underlying its antiangiogenic and antitumor activities (Fig. 6). Furthermore, considering that protease nexin‐1 is also a potent inhibitor of plasmin and thrombin,181 two proteolytic enzymes often present in tumors, and that CgA can be cleaved by these enzymes,41, 170 this inhibitor might also serve to prevent the local cleavage of CgA, thereby preserving its antiangiogenic activity (Fig. 6). In view of the inhibitory activity of circulating full‐length CgA on angiogenesis and tumor growth, and considering that cleavage of its C‐terminal region markedly reduces its activity, it is reasonable to hypothesize that pathophysiological changes of circulating levels of full‐length CgA and/or its fragmentation may contribute to the regulation of disease progression in patients with non‐neuroendocrine tumors.
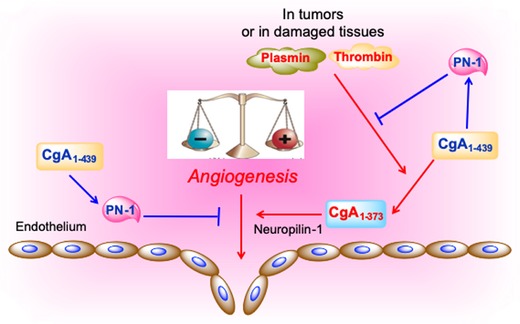
Hypothetical model of the CgA‐dependent angiogenic switch. According to this model, in normal conditions, full‐length CgA1–439 exerts antiangiogenic effects by inducing protease nexin‐1 (PN‐1), an antiangiogenic protein. Since PN‐1 is also a potent inhibitor of plasmin and thrombin, this protein can also prevent CgA cleavage by these enzymes and preserve its antiangiogenic activity. In damaged tissues or tumors, the balance of protease/antiprotease activity is altered leading to CgA cleavage and formation of fragments, for example, CgA1–373, capable of interacting with neuropilin‐1 on endothelial cells (via its PGPQLR sequence) and stimulating angiogenesis (see text).
CgA typically affects tumor growth with a U‐shaped dose–response curve.178 This implies that a marked increase of its concentration may paradoxically result in a reduced effect. For this reason, the effects of CgA on neuroendocrine tumors could be different from those observed in murine models of nonneuroendocrine tumors (and difficult to predict), considering the high concentration that CgA can reach in the tumor microenvironment because of the secretory activity of neuroendocrine cancer cells. Furthermore, one cannot exclude the possibility that the tumor‐derived CgA might be fragmented in a different manner, compared with CgA released in the blood by the neuroendocrine system. Thus, although CgA is a widely used serological and histopathological marker for neuroendocrine tumor diagnosis and follow‐up, development of appropriate models is necessary to address the functional role of CgA and its fragments in patients with such tumors.
Role of CgA1–373 in tumor angiogenesis and growth
Recent studies have shown that a human CgA fragment encompassing residues 1–373 (hCgA1–373; thus lacking the C‐terminal region) promotes angiogenesis by inducing the release of FGF2 from endothelial cells.35, 41, 182 Notably, hCgA1–373, hCgA1–76, and hCgA1–439 can counterbalance the activity of each other in angiogenesis assays,183 suggesting that these proteins may provide a balance of pro‐ and anti‐angiogenic activities tightly regulated by proteolysis. The transition from anti‐ to pro‐angiogenic forms, which can be induced by limited digestion of CgA with plasmin or thrombin,41, 183 occurs in the bone marrow of multiple myeloma patients and correlates with increased tumor microvessel density.170
Studies aimed at elucidating the proangiogenic mechanisms of hCgA1–373 and its role in tumor progression have shown that cleavage of the R373R374 dibasic site of CgA leads to the exposure of the PGPQLR373 epitope (100% conserved in mouse and human CgA), and that this is crucial for tumor progression in various murine models of nonneuroendocrine tumors.184 Indeed, blockade of the PGPQLR site of murine CgA with specific antibodies, unable to recognize full‐length CgA, inhibits tumor growth in various experimental models.184 Blockade of the PGPQLR site with antibodies induces changes in the tumor vascular bed and blood flow in murine fibrosarcomas, suggesting that circulating CgA might work as an “off/on” switch for tumor angiogenesis, when cleaved at the PGPQLR site. Thus, fragmentation of circulating CgA in tumors by local proteases may contribute to “switch on” angiogenesis, and changes in the balance of protease/antiprotease molecules in tumor tissues might represent major mechanisms for regulating the CgA activity (Fig. 6).
Mechanistic studies have shown that hCgA1–373, but not hCgA1–439, can bind neuropilin‐1 with good affinity (K d = 3.49 ± 0.73 nM), suggesting that this protein is an important receptor for hCgA1–373. In particular, the PGPQLR site of hCgA1–373, but not that of hCgA1–439, can bind the VEGF binding‐site of neuropilin‐1.184 It appears, therefore, that the PGPQLR binding site is cryptic in the full‐length molecule or that this region undergoes profound conformational changes upon cleavage of CgA at the R373R374 dibasic site. No binding to neuropilin‐1 occurs with hCgA1–372, a fragment lacking R373, suggesting that the C‐terminal arginine of PGPQLR site (R373) is crucial for binding184 (Fig. 6). Considering that the interaction between fragmented CgA and neuropilin‐1 is important for tumor growth, the PGPQLR site may be a potential therapeutic target.
Neuropilin‐1 is an important coreceptor of various protein factors that regulate vascular permeability and angiogenesis, such as semaphorins and VEGF. The C‐terminal sequence of VEGF (CDKPRR), which contains an R/K‐X‐X‐R/K C‐end rule motif (CendR), can recognize a pocket in the b1 domain of neuropilin‐1, a site that can also accommodate other peptides containing the CendR motif;185, 186 in addition, the PGPQLR site of hCgA1–373 can interact with this pocket of neuropilin‐1.184 Of note, while both hCgA1–373 and VEGF can bind neuropilin‐1 with similar affinity, only VEGF can bind neuropilin‐2.184
It is possible that hCgA1–373 engages other coreceptors for signaling. The hCgA352–372 sequence (catestatin), for example, is known to bind the acetylcholine nicotinic receptor (nAChRs).85, 187, 188 Considering that this class of receptors is expressed on endothelial cells and known to regulate angiogenesis,189, 190, 191, 192 nAChRs might be potential targets. This view is supported by the observation that mecamylamine and α‐bungarotoxin, two nAChRs antagonists, can inhibit the proangiogenic activity of hCgA1–373 in a human umbilical vein endothelial cell (HUVEC) spheroid assay.184 Thus, these receptors, in addition to neuropilin‐1, might play a role in the proangiogenic activity of hCgA1–373.
The R373 residue of hCgA1–373 can be rapidly removed by a plasma carboxypeptidase,184 resulting in the loss of neuropilin‐1 binding and proangiogenic activity and gain of antiangiogenic activity. Interestingly, subnanomolar concentrations of hCgA1–372 (antiangiogenic) can inhibit the activity of subnanomolar levels of hCgA1–373 (proangiogenic), but not that of VEGF and FGF2.184 Thus, the transition of hCgA1–373 to hCgA1–372 may be a specific counter‐regulatory mechanism that serves to limit the activity of hCgA1–373 at the site of production in tumors. Another potential counter‐regulatory mechanism induced by an excess of hCgA1–373 could be related to its biphasic behavior, as suggested by its bell‐shaped dose–response curve observed in angiogenesis assays.41, 178, 184 The mechanisms underlying this behavior are unknown.
In summary, the cleavage of the R373R374 site of CgA in tumors and the subsequent removal of R373 in plasma may provide an important on/off switch for spatiotemporal regulation of angiogenesis in tumors and normal tissues. The interaction of the PGPQLR site with neuropilin‐1 might be, therefore, an important target for angiogenesis inhibition and tumor growth suppression.
Role of full‐length CgA and vasostatin‐1 in tumor cell trafficking
Cancer progression involves the seeding of malignant cells in circulation and the colonization of distant organs. Circulating neoplastic cells can also reinfiltrate the tumor of origin in a process referred to as tumor self‐seeding.177 A study performed in murine models of solid tumors has shown that a modest increase of circulating CgA, as obtained in mice after the administration of omeprazole (a proton pump inhibitor) or low‐dose recombinant hCgA1–439, can reduce tumor self‐seeding and dissemination.177 In particular, the study showed that hCgA1–439 can inhibit shedding of cancer cells in circulation, tumor reinfiltration by circulating cells, and lung colonization in murine models of mouse mammary adenocarcinomas and melanomas. Mechanistic studies showed that hCgA1–439 can reduce endothelial cell gap formation and vascular leakage induced by tumor‐derived factors in tumors, as well as the transendothelial migration of cancer cells.176, 177 Thus, circulating full‐length CgA, besides reducing angiogenesis in tumors, can enhance endothelial barrier function in both tumors and normal tissues thereby inhibiting the trafficking of tumor cells via tumor–to–blood and blood–to–tumor/normal tissue routes (i.e., metastasis and tumor self‐seeding processes).176, 177 Similar effects have been reported for vasostatin‐1.177
A role for circulating CgA in cancer cell trafficking has also been investigated in chronic lymphocytic leukemia (CLL), a hematological cancer characterized by the progressive accumulation of CD5+ leukemic B cells in peripheral blood, lymphoid tissues, and bone marrow, and by the continuous trafficking of these cells among these tissue compartments.193 Physiologically relevant doses of recombinant hCgA1–439 have been shown to reduce the bone marrow/blood ratio of leukemic cells in the Eµ‐TCL1 murine transgenic CCL model, and decrease bone marrow, kidney, and lung infiltration in Rag2 −/− γc (Il2rg)−/− mice engrafted with human MEC1 CLL cells.170 This treatment also reduced the loss of body weight associated with disease progression and improved animal motility. hCgA1–439 was found to enhance the integrity of the endothelial barrier and to reduce the transendothelial migration of MEC1 cells with a bimodal dose–response curve. Vasostatin‐1, but not hCgA1–373, could inhibit CLL progression in the xenograft model, suggesting that the N‐terminal domain contains a site activated by proteolytic cleavage, and that the C‐terminal region is crucial for CgA activity.183 It is therefore possible that CgA and its fragments contribute to regulate leukemic cell trafficking and tissue infiltration in patients with CLL.
Full‐length CgA and response to anticancer therapy
The efficacy of chemotherapy in cancer patients is often limited by inadequate and uneven penetration of drugs in tumor tissues.194, 195 This phenomenon depends on the abnormal vasculature and altered tissue composition typically present in solid tumors, which may cause irregular blood flow, heterogeneous permeability, increased interstitial fluid pressure, vascular compression and, consequently, uneven drug penetration.195, 196, 197 We have shown that NGR‐TNF, a derivative of TNF‐α that targets tumor blood vessels and enhances vascular permeability, improves drug diffusion into neoplastic tissues in various animal models and patients.197, 198, 199, 200 A recent study in patients with primary lymphomas of the central nervous system has shown that this drug can break the blood–brain barrier in tumor and peritumoral areas and enhance the response to chemoimmunotherapy.201
Studies performed in murine lymphoma and melanoma models have shown that pathophysiologically relevant doses of hCgA1–439 are sufficient to reduce the NGR‐TNF–induced penetration of doxorubicin in tumors by enhancing the endothelial barrier function.177 In vitro studies, performed with human endothelial cell monolayers, showed that hCgA1–439 can inhibit the disassembly of VE‐cadherin dependent adherence junctions and permeability to drugs induced by NGR‐TNF. Similar effects in animal models were observed with vasostatin‐1.177 In vitro, this fragment can inhibit various effects exerted by TNF‐α on endothelial cells, such as p38‐MAP kinase phosphorylation, VE‐cadherin redistribution, gap formation, VEGF‐ and thrombin‐induced endothelial cell permeability, and paracellular transport of macromolecules.174, 176, 202, 203 Thus, both full‐length CgA and vasostatin‐1 are efficient inhibitors of TNF‐α–induced alteration of the endothelial barrier function and permeability.
It is therefore possible that increased levels of circulating CgA and its fragments, as may occur in the subpopulation of cancer patients with non‐neuroendocrine tumors, can reduce drug delivery to tumor cells, particularly when they are combined with NGR‐TNF. Of note, daily treatment of mice with omeprazole, a proton pump inhibitor commonly used in the treatment of acid peptic disorders, increased the circulating levels of CgA by twofold and inhibited the penetration of doxorubicin induced by NGR‐TNF in tumors.177 Thus, even a modest enhancement of CgA levels is sufficient to inhibit the synergism of NGR‐TNF with chemotherapy. Considering the wide use of proton pump inhibitors in patients, these findings may have important clinical implications. Therefore, monitoring CgA and its fragments might help to select patients who respond better to this combination therapy. Discontinuation of administration of proton pump inhibitors to patients and/or its replacement with H2 receptor antagonists might increase the response rate in patients with elevated levels of circulating CgA or vasostatin‐1.
Conclusions
The CgA system, comprising full‐length CgA and its fragments, is emerging as an important player in cardiovascular, immunometabolic, and cancer regulation. A major gap of knowledge in this field is related to the receptor mechanisms of the diverse biological functions of the CgA‐related polypeptides, with few exceptions regarding the direct interaction of human CgA with the integrin αv/β6 in the regulation of keratinocyte adhesion,204 CST with nicotinic acetylcholine receptors in the inhibition of catecholamine secretion by chromaffin cells,27, 31, 187, 188, 205, 206 and hCgA1–373 with neuropilin‐1 in angiogenesis and tumor growth stimulation.
In this regard, it is important to highlight the fact that most of the information on the biological functions of CgA and its fragments has been obtained with experiments performed with human‐derived molecules in mouse or rat in vivo models, with only few exceptions. Although the regions corresponding to the main bioactive fragments of CgA show high degree of sequence similarity among different species, the sequences are not identical, introducing the possibility that different receptor mechanisms are brought into play. For example, while human CgA contains an RGD site that can interact with high selectivity and affinity with the integrin αv/β6, this site is changed with QGD in mouse and rat CgA, with loss of integrin binding.204 Furthermore, most experiments with full‐length CgA have been performed with hCgA1–439 produced by recombinant DNA technology in bacterial cells, and thus lacking potentially important posttranslational modifications. These points are potential important limitations of studies performed thus far and may explain some of the controversial results obtained in some cases. Further experiments with syngeneic polypeptides and animal models are necessary to fully elucidate the activity of the CgA system in normal and pathological conditions.
Nevertheless, data have highlighted the complexity of the CgA system in cardiovascular, immunometabolic, and cancer regulation and suggest that qualitative and quantitative characterization of CgA and all its fragments, in normal subjects and diseased patients, are necessary to fully assess the biological role of this system and its potential diagnostic/prognostic value.
Competing interests
The authors declare no competing interests.
Acknowledgments
This work was supported by a Grant from the Department of Veterans Affairs (I01BX000323 to S.K.M.) and by the Associazione Italiana per la Ricerca sul Cancro (AIRC‐5 × 1000 and AIRC‐IG‐19220 to A.C.).
References
Full text links
Read article at publisher's site: https://doi.org/10.1111/nyas.14249
Read article for free, from open access legal sources, via Unpaywall:
https://nyaspubs.onlinelibrary.wiley.com/doi/pdfdirect/10.1111/nyas.14249
Citations & impact
Impact metrics
Citations of article over time
Alternative metrics
Smart citations by scite.ai
Explore citation contexts and check if this article has been
supported or disputed.
https://scite.ai/reports/10.1111/nyas.14249
Article citations
Chromogranin B Protects Human Umbilical Endothelial Cells against Oxidative Stress.
Int J Mol Sci, 25(19):10296, 25 Sep 2024
Cited by: 0 articles | PMID: 39408626 | PMCID: PMC11476595
A Modular Trial of Androgen Signaling Inhibitor Combinations Testing a Risk-Adapted Strategy in Patients with Metastatic Castration-Resistant Prostate Cancer.
Clin Cancer Res, 30(13):2751-2763, 01 Jul 2024
Cited by: 1 article | PMID: 38683200
The Use of Coffee Aroma for Stress Reduction in Postgraduate Dental Students.
Int Dent J, 74(5):1102-1109, 26 Apr 2024
Cited by: 0 articles | PMID: 38677970 | PMCID: PMC11561488
CPLX2 is a novel tumor suppressor and improves the prognosis in glioma.
J Neurooncol, 167(1):63-74, 01 Mar 2024
Cited by: 0 articles | PMID: 38427133
The changes on salivary flow rates, buffering capacity and chromogranin A levels in adults after bariatric surgery.
Clin Oral Investig, 28(3):159, 21 Feb 2024
Cited by: 0 articles | PMID: 38378939
Go to all (42) article citations
Data
Data behind the article
This data has been text mined from the article, or deposited into data resources.
BioStudies: supplemental material and supporting data
SNPs (4)
- (1 citation) dbSNP - rs9658669
- (1 citation) dbSNP - rs965868
- (1 citation) dbSNP - rs9658667
- (1 citation) dbSNP - rs9658666
Similar Articles
To arrive at the top five similar articles we use a word-weighted algorithm to compare words from the Title and Abstract of each citation.
Chromogranin A and derived peptides in health and disease.
J Mol Neurosci, 48(2):347-356, 03 Mar 2012
Cited by: 51 articles | PMID: 22388654 | PMCID: PMC3402615
Review Free full text in Europe PMC
The Emerging Roles of Chromogranins and Derived Polypeptides in Atherosclerosis, Diabetes, and Coronary Heart Disease.
Int J Mol Sci, 22(11):6118, 06 Jun 2021
Cited by: 7 articles | PMID: 34204153 | PMCID: PMC8201018
Review Free full text in Europe PMC
The chromogranin A-derived peptides vasostatin-I and catestatin as regulatory peptides for cardiovascular functions.
Cardiovasc Res, 85(1):9-16, 01 Jan 2010
Cited by: 49 articles | PMID: 19640932
Review
Chromogranin A regulates vesicle storage and mitochondrial dynamics to influence insulin secretion.
Cell Tissue Res, 368(3):487-501, 20 Feb 2017
Cited by: 22 articles | PMID: 28220294 | PMCID: PMC10843982