Abstract
Background
Bitter-taste receptors (TAS2Rs) are involved in airway relaxation but are also expressed in human blood leukocytes. We studied TAS2R expression and the effects of TAS2R agonists on the lipopolysaccharide (LPS)-induced cytokine release in human lung macrophages (LMs).Methods
Lung macrophages were isolated from patients undergoing surgery for carcinoma. We used RT-qPCR to measure transcripts of 16 TAS2Rs (TAS2Rs 3/4/5/7/8/9/10/14/19/20/31/38/39/43/45 and 46) in unstimulated and LPS-stimulated (10 ng.mL-1) LMs. The macrophages were also incubated with TAS2R agonists for 24 h. Supernatant levels of the cytokines TNF-α, CCL3, CXCL8 and IL-10 were measured using ELISAs.Results
The transcripts of all 16 TAS2Rs were detected in macrophages. The addition of LPS led to an increase in the expression of most TAS2Rs, which was significant for TAS2R7 and 38. Although the promiscuous TAS2R agonists, quinine and denatonium, inhibited the LPS-induced release of TNF-α, CCL3 and CXCL8, diphenidol was inactive. Partially selective agonists (dapsone, colchicine, strychnine, and chloroquine) and selective agonists [erythromycin (TAS2R10), phenanthroline (TAS2R5), ofloxacin (TAS2R9), and carisoprodol (TAS2R14)] also suppressed the LPS-induced cytokine release. In contrast, two other agonists [sodium cromoglycate (TAS2R20) and saccharin (TAS2R31 and 43)] were inactive. TAS2R agonists suppressed IL-10 production - suggesting that this anti-inflammatory cytokine is not involved in the inhibition of cytokine production.Conclusion
Human LMs expressed TAS2Rs. Experiments with TAS2R agonists' suggested the involvement of TAS2Rs 3, 4, 5, 9, 10, 14, 30, 39 and 40 in the inhibition of cytokine production. TAS2Rs may constitute new drug targets in inflammatory obstructive lung disease.Free full text

Bitter Taste Receptors (TAS2Rs) in Human Lung Macrophages: Receptor Expression and Inhibitory Effects of TAS2R Agonists
Associated Data
Abstract
Background
Bitter-taste receptors (TAS2Rs) are involved in airway relaxation but are also expressed in human blood leukocytes. We studied TAS2R expression and the effects of TAS2R agonists on the lipopolysaccharide (LPS)-induced cytokine release in human lung macrophages (LMs).
Methods
Lung macrophages were isolated from patients undergoing surgery for carcinoma. We used RT-qPCR to measure transcripts of 16 TAS2Rs (TAS2Rs 3/4/5/7/8/9/10/14/19/20/31/38/39/43/45 and 46) in unstimulated and LPS-stimulated (10 ng.mL–1) LMs. The macrophages were also incubated with TAS2R agonists for 24 h. Supernatant levels of the cytokines TNF-α, CCL3, CXCL8 and IL-10 were measured using ELISAs.
Results
The transcripts of all 16 TAS2Rs were detected in macrophages. The addition of LPS led to an increase in the expression of most TAS2Rs, which was significant for TAS2R7 and 38. Although the promiscuous TAS2R agonists, quinine and denatonium, inhibited the LPS-induced release of TNF-α, CCL3 and CXCL8, diphenidol was inactive. Partially selective agonists (dapsone, colchicine, strychnine, and chloroquine) and selective agonists [erythromycin (TAS2R10), phenanthroline (TAS2R5), ofloxacin (TAS2R9), and carisoprodol (TAS2R14)] also suppressed the LPS-induced cytokine release. In contrast, two other agonists [sodium cromoglycate (TAS2R20) and saccharin (TAS2R31 and 43)] were inactive. TAS2R agonists suppressed IL-10 production – suggesting that this anti-inflammatory cytokine is not involved in the inhibition of cytokine production.
Conclusion
Human LMs expressed TAS2Rs. Experiments with TAS2R agonists’ suggested the involvement of TAS2Rs 3, 4, 5, 9, 10, 14, 30, 39 and 40 in the inhibition of cytokine production. TAS2Rs may constitute new drug targets in inflammatory obstructive lung disease.
Introduction
Inflammatory lung diseases such as asthma and chronic obstructive pulmonary disease (COPD) are characterized by airway obstruction and airflow limitation. The bitter taste receptors (TAS2Rs) constitute a family of around 25 G-protein coupled receptors (GPCRs) initially thought to be located exclusively on the tongue, where their activation enables our perception of a bitter taste. However, TAS2Rs are now known to be expressed in the human bronchus (Deshpande et al., 2010; Grassin-Delyle et al., 2013), airway epithelial cells (Shah et al., 2009; Jaggupilli et al., 2017), mast cells (Ekoff et al., 2014) and blood leukocytes (Orsmark-Pietras et al., 2013; Malki et al., 2015).
In human airway smooth muscle, TAS2R stimulation induces relaxation (Deshpande et al., 2010; Grassin-Delyle et al., 2013); inhaled bitter tastants also decreased airway obstruction in a mouse model of asthma (Deshpande et al., 2010). The motile cilia of human airway epithelial cells express TAS2Rs, and bitter compounds increase the ciliary beat frequency as part of an airway defense mechanism (Shah et al., 2009). In sinonasal epithelial cells, activation of TAS2R38 stimulates an increase in nitric oxide production, which in turn increases mucociliary clearance and directly kills bacteria (Lee et al., 2012; Cohen, 2017). The polymorphisms that underlie TAS2R38’s functionality appear to be involved in susceptibility to upper respiratory bacterial infections (Lee et al., 2012; Cohen, 2017). In IgE-receptor–activated primary human mast cells, agonists known to bind to the expressed TAS2Rs were found to inhibit the release of histamine and prostaglandin D2 (Ekoff et al., 2014). Furthermore, blood leukocytes from children with severe asthma display elevated TAS2R expression levels and two TAS2R agonists inhibited the release of several pro-inflammatory cytokines and eicosanoids in whole blood from adults (Orsmark-Pietras et al., 2013). In general, TAS2R agonists may have both anti-inflammatory properties and bronchodilatory activities.
Lung macrophages are involved in the pathophysiology of pulmonary diseases like asthma or COPD, where the cells orchestrate inflammatory reactions via the release of cytokines and chemokines (Balhara and Gounni, 2012; Yang et al., 2012; Barnes, 2016). The expression of TAS2Rs in lung macrophages (LMs) has not previously been assessed. There are few old reports on the effect of certain bitter tastants (such as chloroquine, colchicine, erythromycin or ofloxacin) on cytokine release from mouse peritoneal macrophages (Ogino et al., 2009), monocyte/macrophage cell lines (Jeong and Jue, 1997; Ianaro et al., 2000; Jang et al., 2006; Schierbeck et al., 2010), human blood monocytes and monocyte-derived macrophages/dendritic cells (Yasutomi et al., 2005; Jang et al., 2006; Schierbeck et al., 2010; Vrancic et al., 2012). However, human blood monocytes or monocyte-derived macrophages are surrogate cell models that do not adequately recapitulate the biology of primary LMs (Jin et al., 2004; Jeyaseelan et al., 2005; Li et al., 2007; Groot-Kormelink et al., 2012; Tomechko et al., 2015).
The objectives of the present study were to (i) characterize TAS2R expression in human LMs, (ii) describe the inhibitory effects of various TAS2R agonists on lipopolysaccharide (LPS)-induced cytokine production, (iii) infer the subtypes of TAS2R involved, and (iv) determine whether IL-10 acts as a potential mediator of TAS2R inhibitory activities on LPS-induced cytokine production.
Materials and Methods
Drugs and Chemicals
The TAS2R agonists chloroquine diphosphate, quinine hydrochloride dihydrate, saccharin sodium hydrate, denatonium benzoate, 1,10-phenanthroline hydrochloride monohydrate, ofloxacin, strychnine hemisulphate, erythromycin, dapsone, carisoprodol, and sodium cromoglycate were obtained from Sigma-Aldrich (Saint-Quentin Fallavier, France) and diphenidol hydrochloride was provided by TCI Europe (Zwijndrecht, Belgium). All products were solubilized and diluted in sterile water, with the exception of erythromycin, dapsone, and carisoprodol, which were solubilized in DMSO and then diluted in water. Antibiotics, DMSO, L-glutamine, trypan blue dye, heat-inactivated fetal calf serum and LPS (from E. coli serotype 0111:B4) were purchased from Sigma (St. Louis, MO, United States). RPMI medium was from Eurobio Biotechnology (Les Ulis, France).
Preparations of Human Lung Macrophages and Explants
Experiments on human tissue were approved by the regional independent ethics committee board (Comité de Protection des Personnes Île de France VIII, Boulogne-Billancourt, France). In line with the French legislation on clinical research and as approved by the independent ethics committee, patients gave their verbal informed consent for the use of resected lung tissue for in vitro experiments. Lung tissue samples were obtained from 28 patients [18 males and 10 females; smoker/ex-smoker/non-smoker: 11/16/1; mean ± standard error (SD) age: 65.4 ± 8.1 years; FEV1 = 80.2 ± 20.9%; pack-years: 41 ± 21; FEV1/FVC ratio: 0.77 ± 0.1; 7 COPD (FEV1/FVC < 0.7; airflow limitation severity: GOLD 1 for 4 patients and GOLD 2 for 3)] undergoing surgical resection for lung carcinoma and who had not received prior chemotherapy. The LMs were isolated from macroscopically normal lung parenchyma (obtained from sites distant from the tumor), dissected free of pleura, visible airways and blood vessels, and then chopped into 3–5 mm3 fragments, as previously described (Jeyaseelan et al., 2005; Buenestado et al., 2010, 2012; Abrial et al., 2015; Victoni et al., 2017).
Briefly, the fluid collected from several washings of the minced peripheral lung tissues was centrifuged (2000 rpm for, 10 min). The cell pellet was resuspended in RPMI supplemented with 10% heat-inactivated fetal calf serum, 2 mM L-glutamine, and antibiotics. Resuspended viable cells (106 per mL) were then aliquoted into either a 12-well plate (for transcriptional assays) or a 24-well plate (for cytokine assays). Following incubation for at least 2 h at 37°C (in a 5% CO2 humidified atmosphere), non-adherent cells were removed by gentle washing. The remaining cells were maintained at 37°C and 5% CO2 overnight. It has been shown that the adherence step does not significantly influence overall transcriptional changes in alveolar macrophages, relative to flow-cytometry-based cell-sorting (Shaykhiev et al., 2009). As described in previous reports from our group (Jeyaseelan et al., 2005; Buenestado et al., 2010, 2012; Abrial et al., 2015; Victoni et al., 2017), the adherent cells (about 2 × 105 cells per well, for a 24-well plate) were >95% pure macrophages, as determined by May-Grünwald-Giemsa staining and CD68 immunocytochemistry (data not shown). Cell viability exceeded 90%, as assessed in a trypan blue dye exclusion assay. Culture plates with adherent macrophages were washed with warm medium. One mL of fresh medium supplemented with 1% fetal calf serum was added per well, and the culture plates were incubated overnight at 37°C in a 5% CO2 humidified atmosphere.
Treatment of Lung Macrophages
On the day after isolation, macrophages or explants were washed twice, and 1 mL of RPMI was added per well. The LMs were exposed for 24 h to LPS (10 ngmL–1). The latter LPS concentration was selected as being submaximal on the basis of previous data from time-response and concentration-response curves (Buenestado et al., 2012). TAS2R agonists were added to the culture medium 1 h before exposure to LPS. After a 24 h incubation in RPMI at 37°C (in a 5% CO2 humidified atmosphere), culture supernatants from the LMs and the explants were collected and stored at −80°C for subsequent cytokine assays. Although more than 100 molecules have been described as TAS2R agonists, some TAS2Rs are still orphan receptors that lack a cognate agonist (Meyerhof et al., 2010; Di Pizio and Niv, 2015). In an initial series of experiments, the preparations were exposed to a maximal concentration (1 mM) of promiscuous TAS2R agonists acting on at least 8 different TAS2Rs (diphenidol, quinine and denatonium) (Table 1) (Meyerhof et al., 2010; Di Pizio and Niv, 2015). In a second series of experiments, the preparations were exposed to a range of concentrations of more selective TAS2R agonists (chloroquine, dapsone, strychnine, sodium cromoglycate, ofloxacin, phenanthroline, erythromycin, and carisoprodol) to infer the TAS2R subtype.
TABLE 1
Compounds selected for functional studies with regard to their ability to act as agonists (or not) of the 25 TAS2R receptors identified in humans to date.
hTAS2R | 1 | 3 | 4 | 5 | 7 | 8 | 9 | 10 | 13 | 14 | 16 | 19 | 20 | 30 | 31 | 38 | 39 | 40 | 41 | 42 | 43 | 45 | 46 | 50 | 60 |
Promiscuous agonists: | |||||||||||||||||||||||||
Diphenidol | 100 | 100 | 10 and 675 ± 186![]() | 30 | 30 | 10 | 100 | 100 | 100 | 3 | 100 | 100 | 30 | 30 | 30 | ||||||||||
Quinine | 10 and 838 ± 24![]() ![]() | 10 and 983 ± 257![]() | 10 | 10 | 10 | 10 | 10 | 10 | 10 | ||||||||||||||||
Denatonium | 300 | 1000 | 3 and 120 ± 56 | 30 | 0.03 and 0.27 ± 0.06 | 100 | 300 | 30 and 240 ± 192 | |||||||||||||||||
Partly selective agonists: | |||||||||||||||||||||||||
Colchicine | 100 and 1025 ± 1219 | 3000 | 300 and 1580 ± 170 | ||||||||||||||||||||||
Chloroquine | 10 and 172 ± 29 | +/0![]() | 10000 | 100 | |||||||||||||||||||||
Dapsone | 100 | 100 | 30 | ||||||||||||||||||||||
Strychnine | +/1000![]() | 3 and 21.8 ± 7.5 | 0.1 and 0.43 ± 0.02 | ||||||||||||||||||||||
Saccharin | + | 170 ± 10 and 1700 ± 20 | 80 ± 60 and 1100 ± 10 | ||||||||||||||||||||||
Sodium cromoglycate | 3000 and 4500 ± 1600 | 10 and 45 ± 25 | 3000 | ||||||||||||||||||||||
Selective agonists: | |||||||||||||||||||||||||
Ofloxacin | EC50 ≈ 200 | ||||||||||||||||||||||||
Phenanthroline | 100 | ||||||||||||||||||||||||
Erythromycin | 300 | ||||||||||||||||||||||||
Carisoprodol | 100 |



Cytokine Assays
The cytokine concentrations in the supernatant (TNF-α, IL-10, CCL3, and CXCL8) were measured with an ELISA (R&D Systems), according to the manufacturer’s instructions. The assays’ limits of detection were 4 pg.mL–1 for CCL3, 8 pg.mL–1 for TNF-α, 30 pg.mL–1 for IL-10, and 32 pg.mL–1 for CXCL8. The supernatants were diluted with RPMI as appropriate, and the optical density was determined at 450 nm using a microplate reader (MRX II, Dynex Technologies, Saint-Cloud, France). Cytokine concentrations were expressed in pg.10–6 LMs, unless otherwise stated. Cytotoxicity was determined by measuring the lactate dehydrogenase (LDH) activity in LM supernatants (LDH assay, Cayman Chemical, Montigny-le-Bretonneux, France) after 24h exposure to the vehicle or the TAS2R agonists.
Reverse Transcriptase – Quantitative Polymerase Chain Reaction (RT-qPCR) Analysis
RT-qPCR experiments were performed as previously described, with some modifications (Grassin-Delyle et al., 2013). Lung macrophages were stimulated (or not) for 24 h with LPS, and total RNA was prepared using TRIzol® reagent (Life Technologies, Saint Aubin, France). The amount of RNA extracted was estimated by spectrophotometry at 260 nm (Biowave DNA; Biochrom, Cambridge, United Kingdom) and its quality was assessed in a microfluidic electrophoresis system (RNA Standard Sensitivity kits for Experion®, BioRad, Marnes-la-Coquette, France). After treatment with DNase I (Life Technologies, Saint Aubin, France), 1 μg of total RNA was subjected to reverse transcription (SuperScript® III First-Strand SuperMix kit, Life Technologies). The resulting cDNA was then used for quantitative real-time PCR experiments with TaqMan® chemistry (Life Technologies). The amplification was carried out using 20 ng cDNA (Gene Expression Master Mix, Life Technologies) in a StepOnePlus thermo cycler (Life Technologies). The conditions were as follows: initial denaturation at 95°C for 10 min, and then 40 cycles of annealing/extension (95°C for 15 s and then 60°C for 1 min). Fluorescence was measured at each cycle, and the real-time PCR’s threshold cycle (Ct) was defined as the point at which a fluorescence signal corresponding to the amplification of a PCR product was detected. The reaction volume was set to 10 μL. The expression of transcripts of the 16 TAS2R-encoding genes (TAS2R3, TAS2R4, TAS2R5, TAS2R7, TAS2R8, TAS2R9, TAS2R10, TAS2R14, TAS2R19, TAS2R20, TAS2R31, TAS2R38, TAS2R39, TAS2R43 TAS2R45, and TAS2R46) in the LMs was analyzed using a specific TaqMan® array with predesigned reagents (Assay-on-Demand®, Life Technologies). In order to confirm the extraction of intact cellular mRNA and standardize the quantitative data, the reference gene coding for hypoxanthine phosphoribosyltransferase (HPRT1) was amplified as the same time.
Statistical Analysis
Values in the text and figures are expressed as the mean ± standard error of the mean (SEM) unless otherwise stated from experiments with n independent donors. The quantitative data obtained from RT-qPCR experiments was expressed as the relative expression (2–ΔCt), where ΔCt is the difference between the target gene Ct and the mean Ct of the reference gene.
Data were evaluated by using either a one-way analysis of variance for repeated measures (followed by Dunnett’s post hoc test for multiple comparisons) or a paired Student’s t-test, as appropriate. The threshold for statistical significance was set to p < 0.05.
Results
Expression of Bitter Taste Receptor Gene Transcripts in Lung Macrophages
We analyzed the expression patterns of 16 of the 25 known TAS2Rs in humans, based on our previous results in human bronchi (Grassin-Delyle et al., 2013) and other literature data in human airway smooth muscle (Deshpande et al., 2010), airway epithelial cells (Shah et al., 2009; Jaggupilli et al., 2017) and blood leukocytes (Orsmark-Pietras et al., 2013; Malki et al., 2015). Transcripts of genes coding for 15 bitter taste receptors were identified in all the preparations (n = 5 to 12), whereas TAS2R43 transcripts were found in LMs from 4 of 6 patients only. Exposure to LPS was generally associated with an increase in TAS2R receptor expression; the increase was statistically significant for TAS2R7 and TAS2R38 (Figure 1).
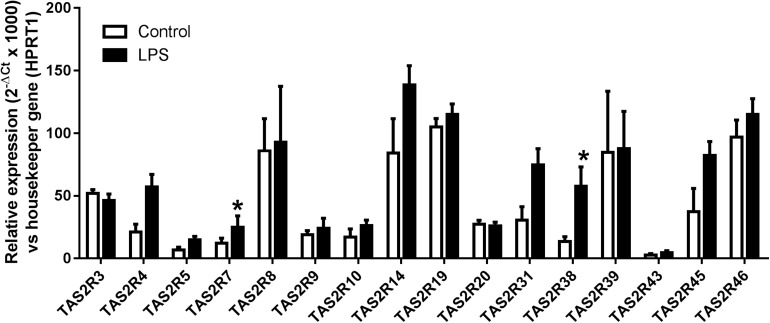
Relative expression (2–ΔCt × 1000) of TAS2R3, 4, 5, 7, 8, 9, 10, 14, 19, 20, 31, 38, 39, 43, 45, and 46 gene transcripts in human LMs (n = 6–12). All transcripts were found to be expressed in all patients, with the exception of TAS2R43 (found in LMs from 4 out of 6 patients only). p < 0.05 relative to the corresponding TAS2R in non-treated controls.
Effects of TAS2R Agonists on Cytokine Production by Human Lung Macrophages
Lipopolysaccharide induced a ~300-fold increase in TNF-α release (from 209 ± 56 pg.10–6 LMs in the absence of LPS to 63151 ± 12561 pg.10–6 LMs in the presence of LPS; n = 6 paired preparations). In the same preparations, the LPS-induced increases in chemokine release were lower: an ~80-fold increase for CCL3 (from 3467 ± 1009 pg.10–6 LMs in the absence of LPS to 276235 ± 71937 pg.10–6 LMs in the presence of LPS) and a 34-fold increase for CXCL8 (from 37 ± 9 pg.10–6 LMs in the absence of LPS to 1279 ± 347 pg.10–6 LMs in the presence of LPS); these findings are in agreement with our previous results (Buenestado et al., 2012; Abrial et al., 2015; Victoni et al., 2017; Grassin-Delyle et al., 2018). The basal release of these cytokines in unstimulated macrophages and the release caused by LPS are shown in Figure 2.
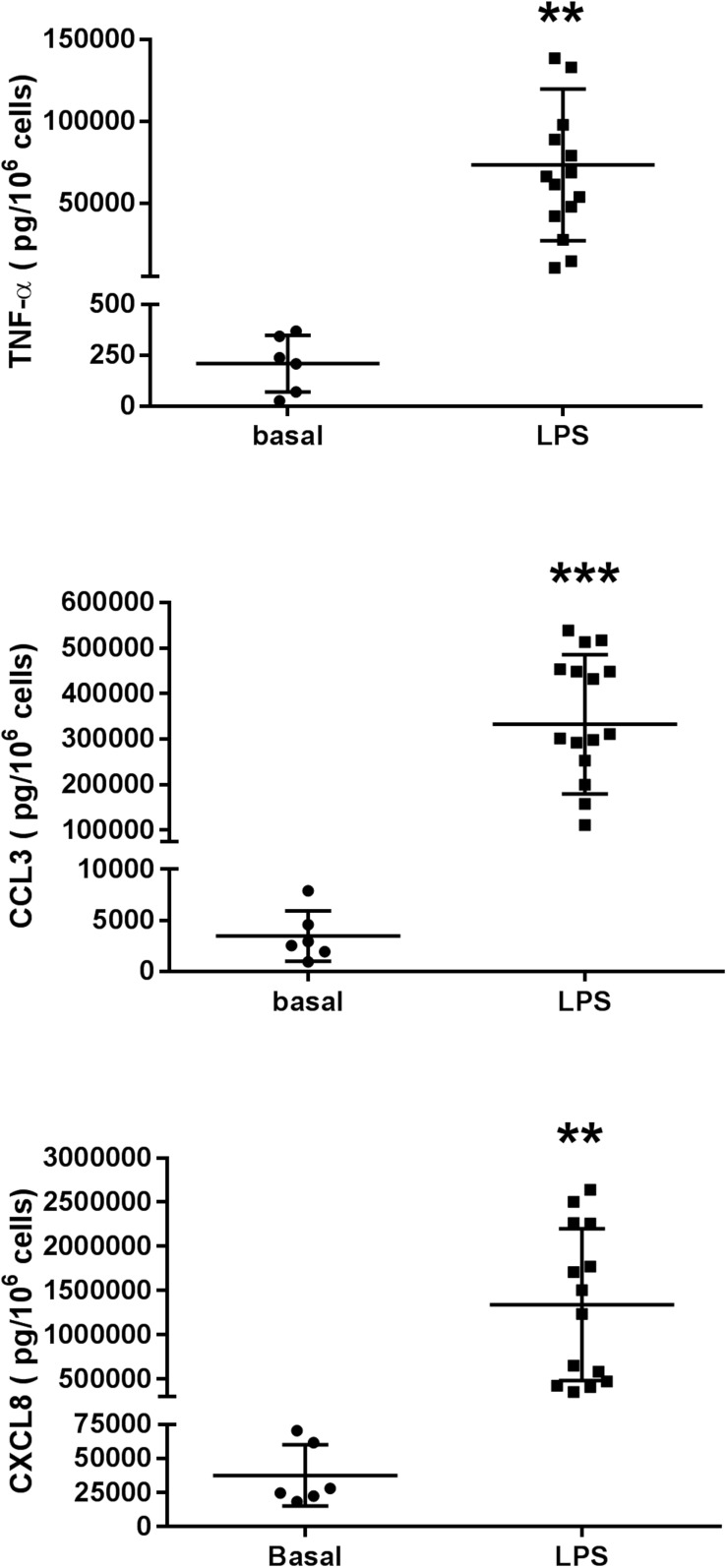
Production of TNF-α, CCL3, and CXCL8 in unstimulated human lung macrophages from 6 patients and in LPS (10 ng.mL–1)-stimulated human lung macrophages from 14 to 15 patients. The results are presented as individual plots and as the mean ± SD level for the group.
The first set of experiments was carried out with three promiscuous TAS2R agonists (diphenidol, quinine, and denatonium) at a concentration of 1 mM (in order to cover the widest possible range of receptors; Table 1). Quinine almost completely abrogated the LMs’ LPS-induced production of TNF-α, CCL3 and CXCL8 (inhibition ≥98%), and denatonium significantly inhibited the production of TNF-α and CCL3 (but not of CXCL8) to a much lesser degree (28% and 43%, respectively). Diphenidol was inactive (Figure 3). Quinine is known to activate all of its nine cognate TAS2Rs in the same relatively low concentration range (~10 μM; Table 1) (Meyerhof et al., 2010; Liu et al., 2018; Jaggupilli et al., 2019). In marked contrast, the activation ranges for denatonium and diphenidol spanned five and three orders of magnitude, respectively (Table 1) (Meyerhof et al., 2010). Given the panels of TAS2R activated by each of these three agonists and the respective activation concentrations, this first set of experiment suggested that the inhibitory effects of quinine and denatonium are almost certainly not mediated by TAS2Rs 1, 7, 13, and 31, and probably not mediated by TAS2Rs 14, 16, 20, 30, 38, 43, and 46.
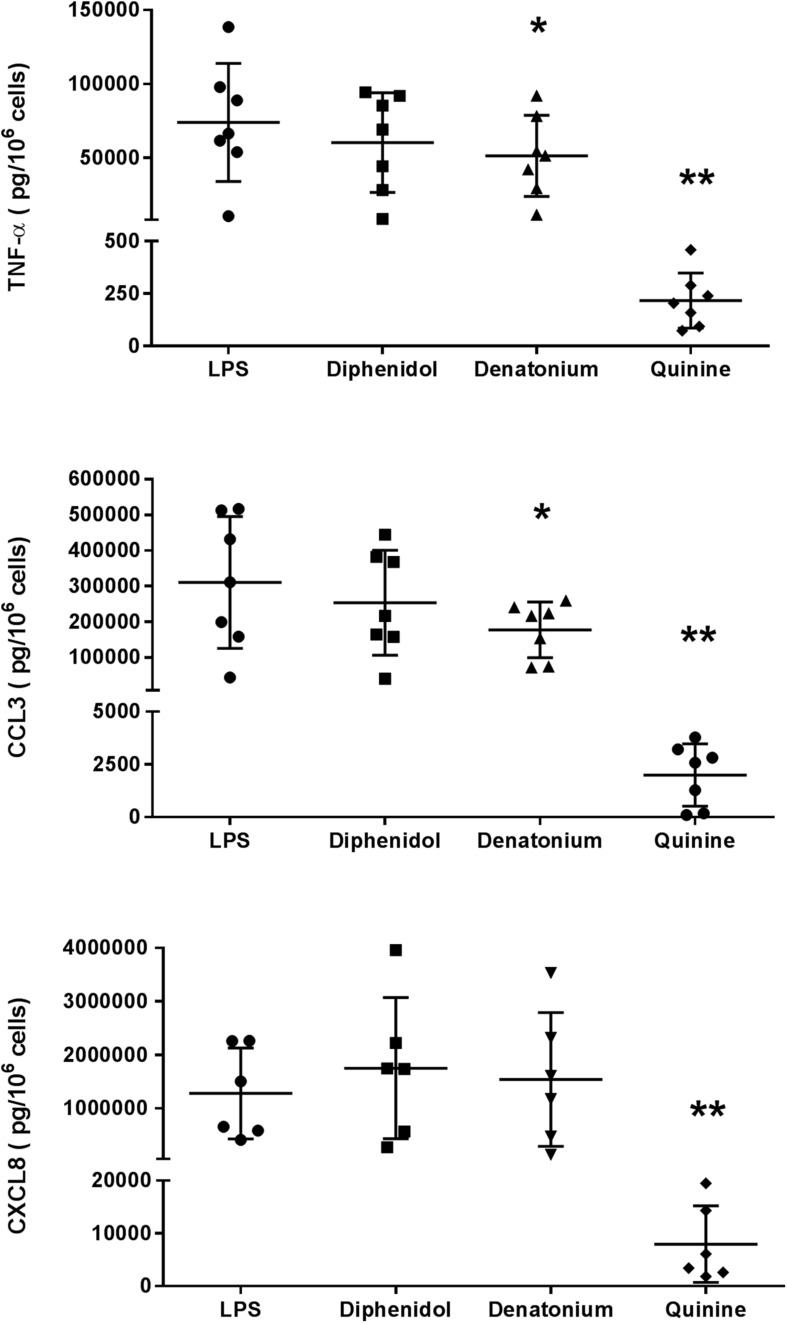
Inhibitory effects of the three promiscuous TAS2R agonists on the LPS-induced production of pro-inflammatory cytokines (TNF-α, CCL3, and CXCL8). Lung macrophages from 6 to 7 patients were stimulated with LPS (10 ng.mL–1) in the absence or presence of the TAS2R agonists (1 mM). p < 0.05,
p < 0.01 compared with LPS alone.
To further determine the TAS2R subtypes involved in the modulation of cytokine release by LMs, relatively selective agonists (Table 1) were used at concentrations ranging from 1 μM to 1 mM. The significant inhibitory activity of dapsone at 0.3 mM (increasing up to 1 mM) suggests the involvement of TAS2Rs 4, 10, and 40 (Figure 4).
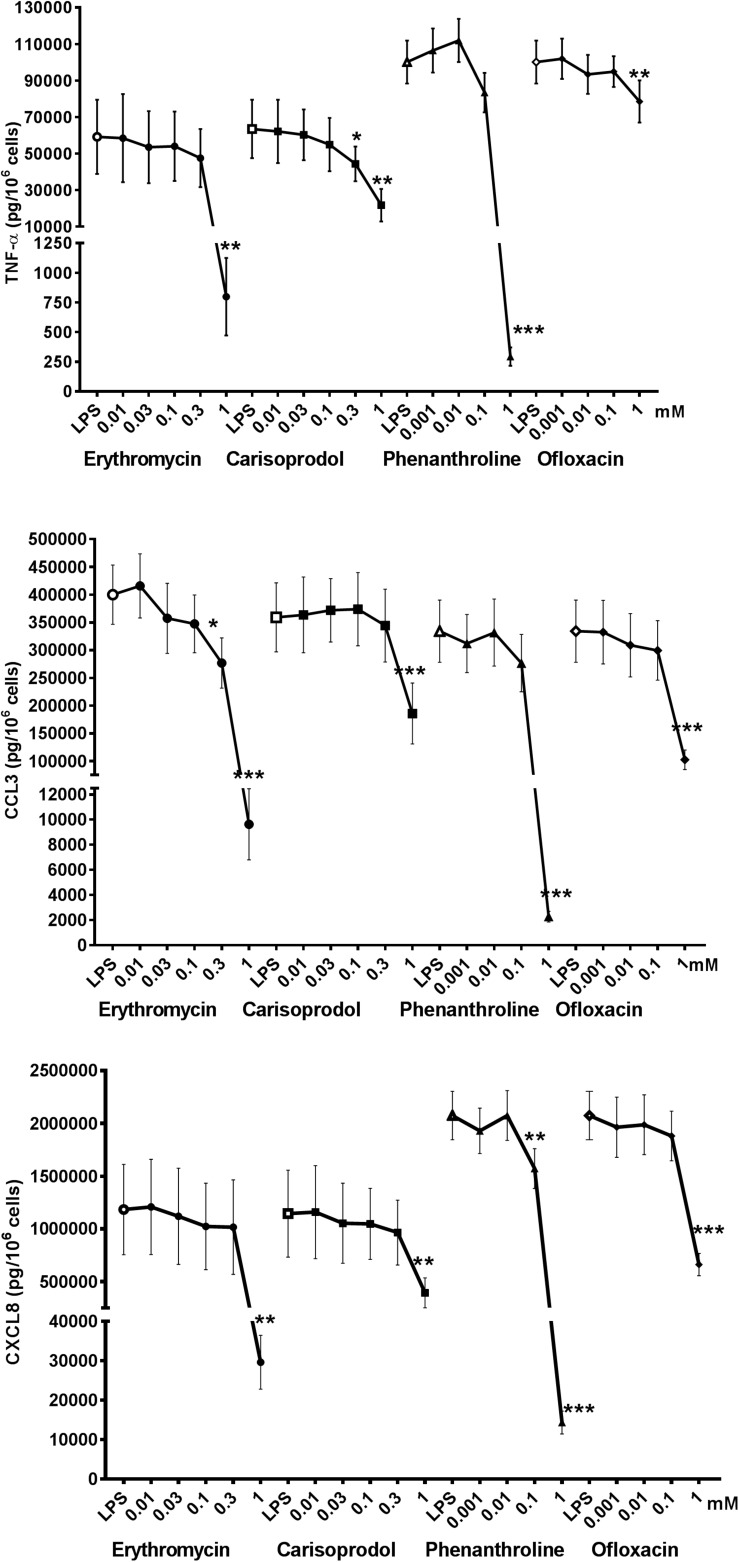
Concentration-response curves for relatively selective TAS2R agonists (colchicine, chloroquine, strychnine, and dapsone) with regard to the LPS-induced production of pro-inflammatory cytokines [TNF-α (🌑) CCL3 (■), and CXCL8 (▲)]. Lung macrophages from 5 to 9 patients were stimulated with LPS (10 ng.mL–1) in the absence or presence of TAS2R agonists. p < 0.05,
p < 0.01,
p < 0.001 compared with LPS alone.
The involvement of TAS2R4 is also indicated by the inhibitory effects of colchicine. Moreover, the observation that partial inhibition (38–57%) by strychnine was observed at a much higher concentration (1 mM) than its EC50 value for TAS2R46 expressed by transfected HEK-293T cells (Table 1) suggests that the latter receptor is not involved but does not rule out the involvement of TAS2R10. Chloroquine was fully active at a concentration of 10–4 M, suggesting the involvement of TAS2R3 and (to a lesser degree) TAS2R39.
Erythromycin, phenanthroline, ofloxacin, and carisoprodol are considered to be selective for a single (different) subtype of TAS2R. Erythromycin is a relatively weak agonist at 1 mM (activation threshold concentration: 0.3 mM) at TAS2R10, although off-target activities could also explain the compound’s inhibitory activity; solid evidence of the involvement of this subtype is hard to obtain. Phenanthroline is a selective TAS2R5 agonist; indeed, it is the only TAS2R5 agonist to have been described to date (Meyerhof et al., 2010). The compound’s potent inhibitory effect in the present study suggests the involvement of this TAS2R subtype. Ofloxacin is selective for TAS2R9, and was associated with significant inhibition of LPS-induced CCL3 and CXCL8 release, which also suggests the involvement of this TAS2R subtype. Carisoprodol is reportedly selective for TAS2R14, with an activation threshold concentration of 0.1 mM. Its significant inhibition of LPS-induced release of the three cytokines suggests the involvement of the TAS2R14, and is consistent with quinine’s inhibitory profile here (Figure 5).
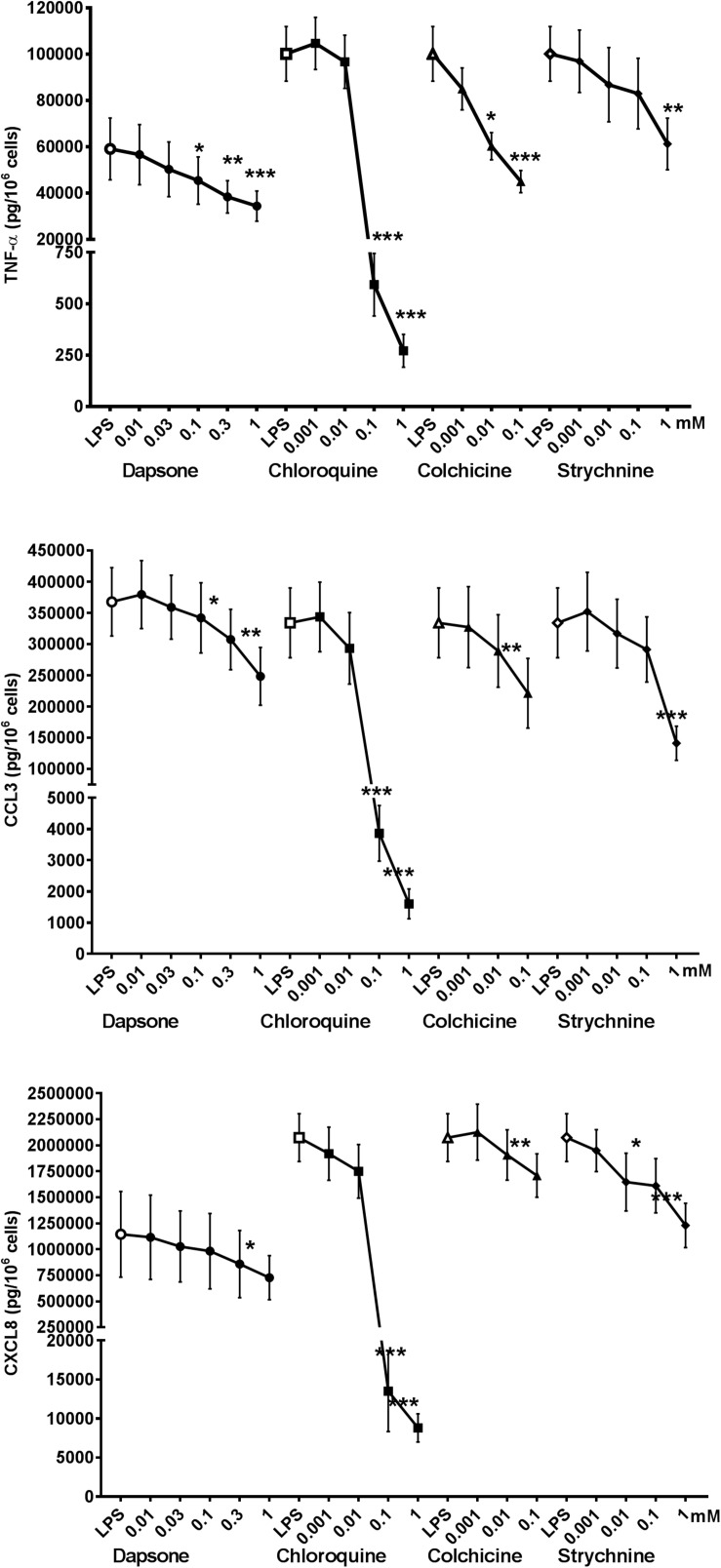
Concentration-response curves of selective TAS2R agonists [ofloxacin (TAS2R9), phenanthroline (TAS2R5), erythromycin (TAS2R10), and carisoprodol (TAS2R14)] on the LPS-induced production of pro-inflammatory cytokines [TNF-α (🌑) CCL3 (■), and CXCL8 (▲)]. Lung macrophages from 5 to 8 patients were stimulated with LPS (10 ng.mL–1), in the absence or presence of TAS2R agonists. p < 0.05,
p < 0.01,
p < 0.001 compared with LPS alone.
Lastly, sodium cromoglycate (a selective TAS2R20 agonist, at an active concentration of 1 mM) and saccharin (an active agonist of both TAS2R31 and 43 at 1 mM) were devoid of activity (data not shown; n = 7 and 8, respectively); this finding rules out the involvement of these three TAS2R subtypes, as had already been indicated by our results with the promiscuous agonists. None of the TAS2R agonists caused a significant increase in LDH release, with the exception of a ~2-fold-increase in LDH release for chloroquine and colchicine at their highest concentrations (1 mM, and 0.1 mM); this corresponds to small increases (1.6 and 1.9%, respectively) above the level of basal LDH release by LMs exposed to LPS.
Overall, cross-tabulation of the effects on LPS-induced cytokine release suggests the highly probable involvement of TAS2Rs 3, 4, 5, 9, 10, 30, 39 and 40 and (with a degree of inconsistency) TAS2R14. The high level of TAS2R14 expression further supports the involvement of this subtype. In contrast, the involvement of TAS2Rs 20, 31 and 43 can be ruled out. Lastly, the results obtained with the present set of agonists could not preclude the involvement of other TAS2Rs (particularly, the orphan receptors TAS2Rs 19, 42, and 50). It is noteworthy that the maximal level of inhibition (≥90%) observed with quinine, chloroquine, phenanthroline and erythromycin was similar to that seen for budesonide at 10–8 M (Table 2).
TABLE 2
Inhibitory effect of budesonide on the LPS-induced production of cytokines.
Cytokine (ng/106 LMs) | LPS | LPS + Bud 10 nM | Inhibition (%) |
TNF-α n = 5 | 64.8 ± 9.4 | 8.2 ± 2.3 ![]() ![]() | 88.2 ± 3.0 |
CCL3 n = 5 | 277.3 ± 29.1 | 22.1 ± 5.9 ![]() ![]() ![]() | 92.2 ± 2.1 |
CXCL8 n = 5 | 1851.7 ± 209.2 | 78.3 ± 13.1 ![]() ![]() ![]() | 95.8 ± 1.2 |
IL-10 n = 5 | 3.2 ± 2.0 | 0.16 ± 0.06 ![]() ![]() ![]() | 95.2 ± 1.0 |






Effects of TAS2R Agonists on IL-10 Production by Human Lung Macrophages
Interleukin-10 is an immunomodulatory cytokine with potent anti-inflammatory activity; as such, it may be an essential mediator of the TAS2R agonists’ ability to inhibit the LPS-induced production of pro-inflammatory cytokines (Berkman et al., 1995; Armstrong et al., 1996). All the TAS2R agonists that inhibited the production of TNF-α, CCL3 and CXCL8 also inhibited (to much the same degree) the LPS-induced production of IL-10. The compounds that did not inhibit the LPS-induced production of TNF-α, CCL3 and CXCL8 did not alter IL-10 production either (Figure 6). Budesonide (10–8 M) also markedly reduced IL-10 production (by 95%; Table 2).
Discussion
Our present results demonstrated that (i) TAS2Rs are indeed expressed in human LMs, and (ii) TAS2R agonists inhibit LPS-induced cytokine release by LMs – a process that is not mediated by the release of IL-10.
Eleven TAS2Rs (TAS2Rs 4, 5, 10, 13, 14, 19, 20, 31, 45, 46, and 50) were expressed in blood leukocytes from children, and some were upregulated in blood leukocytes from patients with severe, treatment-resistant asthma. Overall, TAS2R expression levels are higher in T lymphocytes than in monocytes in blood from adult patients with asthma (Orsmark-Pietras et al., 2013). The relatively weak expression of TAS2Rs in monocytes versus lymphocytes (i.e., B, T and natural killer cells) has been confirmed in healthy adults (Malki et al., 2015). The most abundant TAS2R transcripts in human monocytes differed in the two previously mentioned literature studies. Overall, the greatest expression levels were seen for TAS2Rs 14, 31, 41, 43, 45 and 46. Our study is the first to have demonstrated the expression of sixteen different TAS2Rs in human LMs. The most abundant transcripts in unstimulated LMs were TAS2Rs 8, 14, 19, 39 and 46, and TAS2Rs 31, 38 and 45 after exposure to LPS – highlighting differences in expression patterns between human monocytes and LMs. Since almost all the lung tissue donors were smokers or ex-smokers, we cannot rule out the possibility that smoking had altered the expression of TAS2Rs by LMs. The present work was limited by the fact that it did not investigate TAS2R expression at the protein level (using flow cytometry or Western blotting, for example). However, due the very limited availability of suitable antibodies, studies of the cell surface expression of TAS2R on human blood monocytes or monocyte-derived macrophages have been mainly restricted to TAS2R38 and TAS2R43/31 (Malki et al., 2015; Maurer et al., 2015; Gaida et al., 2016; Tran et al., 2018). However, our present results suggest that these two TAS2Rs are not involved in the inhibitory effects of the TAS2R agonists on the LPS-induced production of cytokines.
There are very few specific antagonists for use as chemical probes of TAS2R function. It has been reported that one antagonist (GIV3727) fully suppressed the activation of six TAS2Rs. GIV3727 resembles bitter agonists in that it acts on several receptors (Behrens and Meyerhof, 2013). Some 6-methoxyflavanones have been described as reversible, insurmountable antagonists of TAS2R39 (Roland et al., 2014). However, this TAS2R subtype is unlikely to be involved in inhibition of the LPS-induced production of cytokines by LMs. Given the current lack of appropriate specific TAS2R antagonists, we sought to infer the receptor subtypes involved in the inhibitory effect of the bitter taste compounds by cross-tabulation of the results for the effect of various TAS2R agonists on LPS-induced cytokine production. In Meyerhof et al.’s (2010) extensive work on HEK cells expressing the different hTAS2Rs, the researchers described the molecular receptive ranges of the 25 human TAS2Rs vs. 104 natural or synthetic bitter compounds. The “threshold concentrations” (defined as the minimum concentrations that elicit a response) and the potencies (EC50) determined for some compounds using calcium imaging analysis of transfected HEK cells may not be transposable to the LMs. However, Meyerhof et al.’s (2010) pioneering work was the basis for our choice of the different TAS2R agonists used in the present study (Table 1). In the human LM model, our cross-tabulation of the TAS2R agonists’ effects on LPS-induced cytokine release suggests that TAS2Rs 3, 4, 5, 9, 10, 14, 30, 39 and 40 (but not 20, 31, and 43) were involved. In a recent publication (Jaggupilli et al., 2019), levofloxacine (the S (-) enantiomer of ofloxacin) was shown to activate TAS2R4, TAS2R14 and TAS2R20 in transfected HEK293T cells further supporting the involvement of TAS2R4 and TAS2R14.
One of the strengths of the present study is its use of a broad panel of TAS2R agonists. One of the limitations relates to potential non-TAS2R-mediated (off-target) activities of some of the agonists, which might have interfered with our analysis and interpretation of the results. For example, the lysosomotropic compound chloroquine inhibits lysosomal functions and also reportedly causes a concentration-dependent suppression of the LPS-induced release of TNF-α, IL-1β, and IL-6 in human monocytes and in monocyte/macrophage cell lines (U937, THP-1, and RAW 264.7) (Jang et al., 2006; Schierbeck et al., 2010). The decrease in TNF-α release has been attributed to chloroquine’s ability to block the conversion of pro-TNF-α to mature TNF-α, whereas the decrease in IL-1β and IL-6 release has been attributed to destabilization the corresponding mRNAs (Jang et al., 2006; Schierbeck et al., 2010). Furthermore, chloroquine reportedly degrades in vitro cell viability at concentrations above 100 μM (Jeong and Jue, 1997; Jang et al., 2006; Schierbeck et al., 2010). However, in addition to the very weak increase in the LDH release by chloroquine (present study), the inhibition of LPS-induced cytokine production was significant at 100 μM in the previous studies (Jeong and Jue, 1997; Jang et al., 2006; Schierbeck et al., 2010) and was substantially complete in the present study – thus ruling out a cytotoxic effect. Macrolides have much the same cationic and lysosomal properties as chloroquine. In the murine monocyte/macrophage cell line J774, four macrolide antibiotics (including erythromycin and azithromycin) reduced the LPS-stimulated production of TNF-α, IL-1β, and IL-6 in a concentration-dependent manner (up to 80 μM) (Ianaro et al., 2000). The impairment of lysosomal functions by azithromycin and chloroquine deregulates TLR4 recycling/signaling and phospholipase activation – leading to an anti-inflammatory phenotype in LPS-stimulated J774 cells (Munic et al., 2011; Nujic et al., 2012). In human monocytes, azithromycin (50 μM) precisely mirrored the effects of chloroquine on LPS-induced cytokine production, with lower levels of some cytokines (CCL22 and CXCL11), higher levels of others (CCL2 and CCL18), and no changes in TNF-α and IL-6 levels (Vrancic et al., 2012). The effects of azithromycin and chloroquine may not be entirely due to impairments in lysosomal functions or in signaling pathways related to NF-κB activation, cellular accumulation and phospholipid binding (Vrancic et al., 2012). To the best of our knowledge, only one study has shown that certain macrolides (clarithromycin and azithromycin, but not erythromycin) can inhibit cytokine production by human alveolar macrophages; clarithromycin was more effective than azithromycin at ~10 μM (Cai et al., 2013). In the present study, erythromycin suppressed LPS-induced cytokine production (TNF-α, CCL3, and CXCL8) at a ~10-fold higher concentration in LMs than in J774 cells. This effect is probably related (at least in part) to activation of TAS2R10 (Meyerhof et al., 2010).
In monocytes and macrophages, cytoskeletal microtubules are involved in a number of cell activities, including cytokine production. Relative to microtubules in monocytes, alveolar macrophage microtubules are longer, more numerous and much more stable, and LPS increases the number and stability of monocyte microtubules still further (Allen et al., 1997). These differences might explain why treatment with 25 μM colchicine (a microtubule-depolymerizing drug) gave a relative increase in LPS-induced IL-1β release and a relative decrease in LPS-induced TNF-α release by human monocytes but had much weaker effects on human alveolar macrophages (Allen et al., 1991). In the present study, however, colchicine was associated with concentration-dependent inhibition of the production of TNF-α and CCL3; this inhibition was relatively weak at 10 μM but significant at 100 μM. These concentrations suggest that colchicine’s effect is exerted (at least in part) through TAS2R receptor activation.
In human blood monocytes, murine monocyte cell lines, and bone marrow-derived dendritic cells stimulated with LPS, chloroquine and azithromycin significantly enhance IL-10 release (Sugiyama et al., 2007; Murphy et al., 2008; Vrancic et al., 2012). Since IL-10 has broad anti-inflammatory properties (Berkman et al., 1995; Armstrong et al., 1996), we assessed the effects of TAS2R agonists on the production of this cytokine by LMs. TAS2R agonists that inhibited the production of TNF-α, CCL3, and CXCL8 also inhibited IL-10 production to a similar extent. Budesonide also markedly reduced the IL-10 production. Hence, in contrast to the situation in monocytes, IL-10 is not involved in the TAS2R agonists’ inhibitory effects in LMs. The molecular mechanisms underlying the anti-inflammatory effects of TAS2R activation in human lung macrophages should be investigated as soon as selective, potent TAS2R agonists and antagonists become available. Taken as a whole, these results suggest that a battery of selective TAS2R agonists and antagonists will be needed to confirm our findings and then to establish the full list of receptors involved in the inhibition of LPS-induced cytokine release. With respect to drug efficacy, some of the active TAS2R agonists (quinine, chloroquine, phenanthroline, and erythromycin) led to the essentially complete inhibition of LPS-induced cytokine production by LMs (to much the same extent as an optimal concentration of budesonide), which illustrates the potent anti-inflammatory activity of these compounds and the potential therapeutic value of inhaled TAS2R agonists in obstructive pulmonary diseases. Interestingly, it has been reported that chloroquine has beneficial effects in asthma (Charous et al., 1998). Given the potential TAS2R-mediated effects observed here, it is time to review the actions of chloroquine and related compounds in airway diseases.
Another potential study limitation relates to our use of LMs harvested from lung tissues resected from current smokers or ex-smokers. Isolation from minced lung tissue provides the large number of cells required to perform paired series of experiments, such as those described here. This work would be hardly possible with macrophages obtained from bronchoalveolar lavages. It should be noted that our preparations might contain a small proportion of interstitial resident macrophages among the alveolar macrophages. However, exposure to bacterial products (including LPS) and inhaled TAS2R agonists is not restricted to the alveolar compartment, and the use of freshly isolated human LMs mainly from the alveolar spaces but perhaps also from the lung tissue might usefully reflect the clinical response more closely.
About a quarter of the lung tissue donors studied here had COPD and almost all were smokers or ex-smokers. The impact of smoking status and COPD on LPS-induced cytokine release by LMs varies markedly from one study to another and from one cytokine to another. Some researchers have reported that LPS-stimulated cytokine production by alveolar macrophages was higher in COPD patients and smokers than in healthy non-smokers (Hodge et al., 2011). However, there were no significant differences in cytokine secretion between current smokers with COPD and non-smokers with COPD (Hodge et al., 2011). In contrast, other studies have found that smoking reduces cytokine production by alveolar macrophages upon stimulation with LPS (Chen et al., 2007) or that current smoking status had no effect (i.e., the dose–response curve for any of the cytokines stimulated by LPS was similar in current smokers and ex-smokers) (Chen et al., 2007; Armstrong et al., 2009, 2011). Furthermore, the inhibitory effect of corticosteroids on the LPS-induced release of cytokines from LMs or alveolar macrophages isolated from bronchoalveolar lavages was similar (i) in non-smokers, current smokers and COPD patients and (ii) after a short (1 h) vs. long (16 h) plate adherence step in the isolation procedure (Armstrong et al., 2011; Plumb et al., 2013; Higham et al., 2014, 2015). Hence, smoking status and COPD impair LPS-induced cytokine release to a variable extent but do not influence the inhibitory effect of corticosteroids on LMs. Therefore, the inhibitory effects of TAS2R agonists observed in the present study are probably not restricted to LMs from ex-smokers or current smokers, and are likely to accurately reflect the in vivo responsiveness of human LMs.
In conclusion, we evidenced TAS2R transcript expression in human LMs and identified the TAS2R subtypes that are most likely to be involved in the inhibition of LPS-induced cytokine production. Furthermore, the potential value of TAS2Rs as drug targets for the treatment of chronic obstructive lung diseases is enhanced by the ability of TAS2R agonists to relax airway smooth muscle (Deshpande et al., 2010; Grassin-Delyle et al., 2013), even when β2-adrenergic receptors (the current cornerstone target of bronchodilators) are subject to tachyphylaxis (An et al., 2012).
DATA AVAILABILITY STATEMENT
All datasets generated for this study are included in the manuscript/supplementary files.
ETHICS STATEMENT
Experiments on human tissue were approved by the regional independent ethics committee board (Comité de Protection des Personnes Île de France VIII, Boulogne-Billancourt, France).
Author Contributions
SG-D and HS conceived the study, performed the experiments, analyzed the data, and critically revised the manuscript. NM helped to draft the manuscript and critically revised it. CA, MB, and EN performed the experiments and analyzed the data. CF and L-JC analyzed the data and critically revised the manuscript. PD managed the study, analyzed the data, performed the statistical analysis, and drafted the manuscript. All authors read and approved the final manuscript.
Conflict of Interest
The authors declare that the research was conducted in the absence of any commercial or financial relationships that could be construed as a potential conflict of interest.
Acknowledgments
The authors wish to thank David Fraser Ph.D. for editorial assistance.
Funding
The research was funded by the French Ministry of Higher Education (Chancellerie des Universités de Paris, Legs Gaston Poix).
References
- Abrial C., Grassin-Delyle S., Salvator H., Brollo M., Naline E., Devillier P. (2015). 15-Lipoxygenases regulate the production of chemokines in human lung macrophages. Br. J. Pharmacol. 172 4319–4330. 10.1111/bph.13210 [Europe PMC free article] [Abstract] [CrossRef] [Google Scholar]
- Alexander S. P. H., Mathie A., Peters J. A. (2011). G protein-coupled receptors. Br. J. Pharmacol. 164 S5–S113. 10.1111/j.1476-5381.2011.01649_3.x [Europe PMC free article] [Abstract] [CrossRef] [Google Scholar]
- Allen J. N., Herzyk D. J., Wewers M. D. (1991). Colchicine has opposite effects on interleukin-1 beta and tumor necrosis factor-alpha production. Am. J. Physiol. 261(4 Pt 1), L315–L321. 10.1152/ajplung.1991.261.4.L315 [Abstract] [CrossRef] [Google Scholar]
- Allen J. N., Moore S. A., Liao Z., Wewers M. D. (1997). Changes in mononuclear phagocyte microtubules after endotoxin stimulation. I. changes in microtubule stability. Am. J. Respir. Cell Mol. Biol. 16 119–126. 10.1165/ajrcmb.16.2.9032118 [Abstract] [CrossRef] [Google Scholar]
- An S. S., Wang W. C., Koziol-White C. J., Ahn K., Lee D. Y., Kurten R. C., et al. (2012). TAS2R activation promotes airway smooth muscle relaxation despite beta(2)-adrenergic receptor tachyphylaxis. Am. J. Physiol. Lung Cell. Mol. Physiol. 303 L304–L311. 10.1152/ajplung.00126.2012 [Europe PMC free article] [Abstract] [CrossRef] [Google Scholar]
- Andres-Barquin P. J., Conte C. (2004). Molecular basis of bitter taste: the T2R family of G protein-coupled receptors. Cell Biochem. Biophys. 41 99–112. 10.1385/CBB:41:1:099 [Abstract] [CrossRef] [Google Scholar]
- Armstrong J., Harbron C., Lea S., Booth G., Cadden P., Wreggett K. A., et al. (2011). Synergistic effects of p38 mitogen-activated protein kinase inhibition with a corticosteroid in alveolar macrophages from patients with chronic obstructive pulmonary disease. J. Pharmacol. Exp. Ther. 338 732–740. 10.1124/jpet.111.180737 [Abstract] [CrossRef] [Google Scholar]
- Armstrong J., Sargent C., Singh D. (2009). Glucocorticoid sensitivity of lipopolysaccharide-stimulated chronic obstructive pulmonary disease alveolar macrophages. Clin. Exp. Immunol. 158 74–83. 10.1111/j.1365-2249.2009.03986.x [Abstract] [CrossRef] [Google Scholar]
- Armstrong L., Jordan N., Millar A. (1996). Interleukin 10 (IL-10) regulation of tumour necrosis factor alpha (TNF-alpha) from human alveolar macrophages and peripheral blood monocytes. Thorax 51 143–149. 10.1136/thx.51.2.143 [Europe PMC free article] [Abstract] [CrossRef] [Google Scholar]
- Balhara J., Gounni A. S. (2012). The alveolar macrophages in asthma: a double-edged sword. Mucosal Immunol. 5 605–609. 10.1038/mi.2012.74 [Abstract] [CrossRef] [Google Scholar]
- Barnes P. J. (2016). Inflammatory mechanisms in patients with chronic obstructive pulmonary disease. J. Allergy Clin. Immunol. 138 16–27. 10.1016/j.jaci.2016.05.011 [Abstract] [CrossRef] [Google Scholar]
- Behrens M., Meyerhof W. (2013). Bitter taste receptor research comes of age: from characterization to modulation of TAS2Rs. Semin. Cell Dev. Biol. 24 215–221. 10.1016/j.semcdb.2012.08.006 [Abstract] [CrossRef] [Google Scholar]
- Berkman N., John M., Roesems G., Jose P. J., Barnes P. J., Chung K. F. (1995). Inhibition of macrophage inflammatory protein-1 alpha expression by IL-10. differential sensitivities in human blood monocytes and alveolar macrophages. J. Immunol. 155 4412–4418. [Abstract] [Google Scholar]
- Buenestado A., Grassin Delyle S., Arnould I., Besnard F., Naline E., Blouquit-Laye S., et al. (2010). The role of adenosine receptors in regulating production of tumour necrosis factor-alpha and chemokines by human lung macrophages. Br. J. Pharmacol. 159 1304–1311. 10.1111/j.1476-5381.2009.00614.x [Europe PMC free article] [Abstract] [CrossRef] [Google Scholar]
- Buenestado A., Grassin-Delyle S., Guitard F., Naline E., Faisy C., Israel-Biet D., et al. (2012). Roflumilast inhibits the release of chemokines and TNF-alpha from human lung macrophages stimulated with lipopolysaccharide. Br. J. Pharmacol. 165 1877–1890. 10.1111/j.1476-5381.2011.01667.x [Europe PMC free article] [Abstract] [CrossRef] [Google Scholar]
- Cai M., Bonella F., Dai H., Sarria R., Guzman J., Costabel U. (2013). Macrolides inhibit cytokine production by alveolar macrophages in bronchiolitis obliterans organizing pneumonia. Immunobiology 218 930–937. 10.1016/j.imbio.2012.10.014 [Abstract] [CrossRef] [Google Scholar]
- Charous B. L., Halpern E. F., Steven G. C. (1998). Hydroxychloroquine improves airflow and lowers circulating IgE levels in subjects with moderate symptomatic asthma. J. Allergy Clin. Immunol. 102 198–203. 10.1016/s0091-6749(98)70086-7 [Abstract] [CrossRef] [Google Scholar]
- Chen H., Cowan M. J., Hasday J. D., Vogel S. N., Medvedev A. E. (2007). Tobacco smoking inhibits expression of proinflammatory cytokines and activation of IL-1R-associated kinase, p38, and NF-kappaB in alveolar macrophages stimulated with TLR2 and TLR4 agonists. J. Immunol. 179 6097–6106. 10.4049/jimmunol.179.9.6097 [Abstract] [CrossRef] [Google Scholar]
- Cohen N. A. (2017). The genetics of the bitter taste receptor T2R38 in upper airway innate immunity and implications for chronic rhinosinusitis. Laryngoscope 127 44–51. 10.1002/lary.26198 [Europe PMC free article] [Abstract] [CrossRef] [Google Scholar]
- Deshpande D. A., Wang W. C., McIlmoyle E. L., Robinett K. S., Schillinger R. M., An S. S., et al. (2010). Bitter taste receptors on airway smooth muscle bronchodilate by localized calcium signaling and reverse obstruction. Nat. Med. 16 1299–1304. 10.1038/nm.2237 [Europe PMC free article] [Abstract] [CrossRef] [Google Scholar]
- Di Pizio A., Niv M. Y. (2015). Promiscuity and selectivity of bitter molecules and their receptors. Bioorg. Med. Chem. 23 4082–4091. 10.1016/j.bmc.2015.04.025 [Abstract] [CrossRef] [Google Scholar]
- Dotson C. D., Zhang L., Xu H., Shin Y. K., Vigues S., Ott S. H., et al. (2008). Bitter taste receptors influence glucose homeostasis. PLoS One 3:e3974. 10.1371/journal.pone.0003974 [Europe PMC free article] [Abstract] [CrossRef] [Google Scholar]
- Ekoff M., Choi J. H., James A., Dahlen B., Nilsson G., Dahlen S. E. (2014). Bitter taste receptor (TAS2R) agonists inhibit IgE-dependent mast cell activation. J. Allergy Clin. Immunol. 134 475–478. 10.1016/j.jaci.2014.02.029 [Abstract] [CrossRef] [Google Scholar]
- Gaida M. M., Dapunt U., Hansch G. M. (2016). Sensing developing biofilms: the bitter receptor T2R38 on myeloid cells. Pathog. Dis. 74:ftw004. 10.1093/femspd/ftw004 [Europe PMC free article] [Abstract] [CrossRef] [Google Scholar]
- Grassin-Delyle S., Abrial C., Fayad-Kobeissi S., Brollo M., Faisy C., Alvarez J. C., et al. (2013). The expression and relaxant effect of bitter taste receptors in human bronchi. Respir. Res. 14:134. 10.1186/1465-9921-14-134 [Europe PMC free article] [Abstract] [CrossRef] [Google Scholar]
- Grassin-Delyle S., Abrial C., Salvator H., Brollo M., Naline E., Devillier P. (2018). The role of toll-like receptors in the production of cytokines by human lung macrophages. J. Innate Immun. 10.1159/000494463 [Epub ahead of print]. [Europe PMC free article] [Abstract] [CrossRef] [Google Scholar]
- Groot-Kormelink P. J., Fawcett L., Wright P. D., Gosling M., Kent T. C. (2012). Quantitative GPCR and ion channel transcriptomics in primary alveolar macrophages and macrophage surrogates. BMC Immunol. 13:57. 10.1186/1471-2172-13-57 [Europe PMC free article] [Abstract] [CrossRef] [Google Scholar]
- Higham A., Booth G., Lea S., Southworth T., Plumb J., Singh D. (2015). The effects of corticosteroids on COPD lung macrophages: a pooled analysis. Respir. Res. 16:98. 10.1186/s12931-015-0260-0 [Europe PMC free article] [Abstract] [CrossRef] [Google Scholar]
- Higham A., Lea S., Ray D., Singh D. (2014). Corticosteroid effects on COPD alveolar macrophages: dependency on cell culture methodology. J. Immunol. Methods 405 144–153. 10.1016/j.jim.2014.02.003 [Europe PMC free article] [Abstract] [CrossRef] [Google Scholar]
- Hodge S., Matthews G., Mukaro V., Ahern J., Shivam A., Hodge G., et al. (2011). Cigarette smoke-induced changes to alveolar macrophage phenotype and function are improved by treatment with procysteine. Am. J. Respir. Cell Mol. Biol. 44 673–681. 10.1165/rcmb.2009-0459OC [Abstract] [CrossRef] [Google Scholar]
- Ianaro A., Ialenti A., Maffia P., Sautebin L., Rombola L., Carnuccio R., et al. (2000). Anti-inflammatory activity of macrolide antibiotics. J. Pharmacol. Exp. Ther. 292 156–163. [Abstract] [Google Scholar]
- Jaggupilli A., Singh N., De Jesus V. C., Gounni M. S., Dhanaraj P., Chelikani P. (2019). Chemosensory bitter taste receptors (T2Rs) are activated by multiple antibiotics. FASEB J. 33 501–517. 10.1096/fj.201800521RR [Abstract] [CrossRef] [Google Scholar]
- Jaggupilli A., Singh N., Upadhyaya J., Sikarwar A. S., Arakawa M., Dakshinamurti S., et al. (2017). Analysis of the expression of human bitter taste receptors in extraoral tissues. Mol. Cell Biochem. 426 137–147. 10.1007/s11010-016-2902-z [Abstract] [CrossRef] [Google Scholar]
- Jang C. H., Choi J. H., Byun M. S., Jue D. M. (2006). Chloroquine inhibits production of TNF-alpha, IL-1beta and IL-6 from lipopolysaccharide-stimulated human monocytes/macrophages by different modes. Rheumatology 45 703–710. 10.1093/rheumatology/kei282 [Abstract] [CrossRef] [Google Scholar]
- Jeong J. Y., Jue D. M. (1997). Chloroquine inhibits processing of tumor necrosis factor in lipopolysaccharide-stimulated RAW 264.7 macrophages. J. Immunol. 158 4901–4907. [Abstract] [Google Scholar]
- Jeyaseelan S., Manzer R., Young S. K., Yamamoto M., Akira S., Mason R. J., et al. (2005). Toll-IL-1 receptor domain-containing adaptor protein is critical for early lung immune responses against Escherichia coli lipopolysaccharide and viable Escherichia coli. J. Immunol. 175 7484–7495. 10.4049/jimmunol.175.11.7484 [Abstract] [CrossRef] [Google Scholar]
- Jin M., Opalek J. M., Marsh C. B., Wu H. M. (2004). Proteome comparison of alveolar macrophages with monocytes reveals distinct protein characteristics. Am. J. Respir. Cell Mol. Biol. 31 322–329. 10.1165/rcmb.2004-0080OC [Abstract] [CrossRef] [Google Scholar]
- Lee R. J., Xiong G., Kofonow J. M., Chen B., Lysenko A., Jiang P., et al. (2012). T2R38 taste receptor polymorphisms underlie susceptibility to upper respiratory infection. J. Clin. Invest. 122 4145–4159. 10.1172/JCI64240 [Europe PMC free article] [Abstract] [CrossRef] [Google Scholar]
- Li J., Pritchard D. K., Wang X., Park D. R., Bumgarner R. E., Schwartz S. M., et al. (2007). cDNA microarray analysis reveals fundamental differences in the expression profiles of primary human monocytes, monocyte-derived macrophages, and alveolar macrophages. J. Leukoc. Biol. 81 328–335. 10.1189/jlb.0206124 [Abstract] [CrossRef] [Google Scholar]
- Liu K., Jaggupilli A., Premnath D., Chelikani P. (2018). Plasticity of the ligand binding pocket in the bitter taste receptor T2R7. Biochim. Biophys. Acta Biomembr. 1860 991–999. 10.1016/j.bbamem.2018.01.014 [Abstract] [CrossRef] [Google Scholar]
- Malki A., Fiedler J., Fricke K., Ballweg I., Pfaffl M. W., Krautwurst D. (2015). Class I odorant receptors, TAS1R and TAS2R taste receptors, are markers for subpopulations of circulating leukocytes. J. Leukoc. Biol. 97 533–545. 10.1189/jlb.2A0714-331RR [Europe PMC free article] [Abstract] [CrossRef] [Google Scholar]
- Maurer S., Wabnitz G. H., Kahle N. A., Stegmaier S., Prior B., Giese T., et al. (2015). Tasting Pseudomonas aeruginosa biofilms: human neutrophils express the bitter receptor T2R38 as sensor for the quorum sensing molecule N-(3-Oxododecanoyl)-l-Homoserine Lactone. Front. Immunol. 6:369. 10.3389/fimmu.2015.00369 [Europe PMC free article] [Abstract] [CrossRef] [Google Scholar]
- Meyerhof W., Batram C., Kuhn C., Brockhoff A., Chudoba E., Bufe B., et al. (2010). The molecular receptive ranges of human TAS2R bitter taste receptors. Chem. Senses 35 157–170. 10.1093/chemse/bjp092 [Abstract] [CrossRef] [Google Scholar]
- Munic V., Banjanac M., Kostrun S., Nujic K., Bosnar M., Marjanovic N., et al. (2011). Intensity of macrolide anti-inflammatory activity in J774A.1 cells positively correlates with cellular accumulation and phospholipidosis. Pharmacol. Res. 64 298–307. 10.1016/j.phrs.2011.03.011 [Abstract] [CrossRef] [Google Scholar]
- Murphy B. S., Sundareshan V., Cory T. J., Hayes D., Jr., Anstead M. I., Feola D. J. (2008). Azithromycin alters macrophage phenotype. J. Antimicrob. Chemother. 61 554–560. 10.1093/jac/dkn007 [Abstract] [CrossRef] [Google Scholar]
- Nujic K., Banjanac M., Munic V., Polancec D., Erakovic Haber V. (2012). Impairment of lysosomal functions by azithromycin and chloroquine contributes to anti-inflammatory phenotype. Cell. Immunol. 279 78–86. 10.1016/j.cellimm.2012.09.007 [Abstract] [CrossRef] [Google Scholar]
- Ogino H., Fujii M., Ono M., Maezawa K., Hori S., Kizu J. (2009). In vivo and in vitro effects of fluoroquinolones on lipopolysaccharide-induced pro-inflammatory cytokine production. J. Infect. Chemother. 15 168–173. 10.1007/s10156-009-0680-1 [Abstract] [CrossRef] [Google Scholar]
- Orsmark-Pietras C., James A., Konradsen J. R., Nordlund B., Soderhall C., Pulkkinen V., et al. (2013). Transcriptome analysis reveals upregulation of bitter taste receptors in severe asthmatics. Eur. Respir. J. 42 65–78. 10.1183/09031936.00077712 [Abstract] [CrossRef] [Google Scholar]
- Plumb J., Robinson L., Lea S., Banyard A., Blaikley J., Ray D., et al. (2013). Evaluation of glucocorticoid receptor function in COPD lung macrophages using beclomethasone-17-monopropionate. PLoS One 8:e64257. 10.1371/journal.pone.0064257 [Europe PMC free article] [Abstract] [CrossRef] [Google Scholar]
- Roland W. S., Gouka R. J., Gruppen H., Driesse M., van Buren L., Smit G., et al. (2014). 6-methoxyflavanones as bitter taste receptor blockers for hTAS2R39. PLoS One 9:e94451. 10.1371/journal.pone.0094451 [Europe PMC free article] [Abstract] [CrossRef] [Google Scholar]
- Sainz E., Cavenagh M. M., Gutierrez J., Battey J. F., Northup J. K., Sullivan S. L. (2007). Functional characterization of human bitter taste receptors. Biochem. J. 403 537–543. 10.1042/BJ20061744 [Europe PMC free article] [Abstract] [CrossRef] [Google Scholar]
- Schierbeck H., Wahamaa H., Andersson U., Harris H. E. (2010). Immunomodulatory drugs regulate HMGB1 release from activated human monocytes. Mol. Med. 16 343–351. 10.2119/molmed.2010.00031 [Europe PMC free article] [Abstract] [CrossRef] [Google Scholar]
- Shah A. S., Ben-Shahar Y., Moninger T. O., Kline J. N., Welsh M. J. (2009). Motile cilia of human airway epithelia are chemosensory. Science 325 1131–1134. 10.1126/science.1173869 [Europe PMC free article] [Abstract] [CrossRef] [Google Scholar]
- Shaykhiev R., Krause A., Salit J., Strulovici-Barel Y., Harvey B. G., O’Connor T. P., et al. (2009). Smoking-dependent reprogramming of alveolar macrophage polarization: implication for pathogenesis of chronic obstructive pulmonary disease. J. Immunol. 183 2867–2883. 10.4049/jimmunol.0900473 [Europe PMC free article] [Abstract] [CrossRef] [Google Scholar]
- Soares S., Kohl S., Thalmann S., Mateus N., Meyerhof W., De Freitas V. (2013). Different phenolic compounds activate distinct human bitter taste receptors. J. Agric. Food Chem. 61 1525–1533. 10.1021/jf304198k [Abstract] [CrossRef] [Google Scholar]
- Sugiyama K., Shirai R., Mukae H., Ishimoto H., Nagata T., Sakamoto N., et al. (2007). Differing effects of clarithromycin and azithromycin on cytokine production by murine dendritic cells. Clin. Exp. Immunol. 147 540–546. 10.1111/j.1365-2249.2007.03299.x [Abstract] [CrossRef] [Google Scholar]
- Tomechko S. E., Lundberg K. C., Jarvela J., Bebek G., Chesnokov N. G., Schlatzer D., et al. (2015). Proteomic and bioinformatics profile of paired human alveolar macrophages and peripheral blood monocytes. Proteomics 15 3797–3805. 10.1002/pmic.201400496 [Europe PMC free article] [Abstract] [CrossRef] [Google Scholar]
- Tran H. T. T., Herz C., Ruf P., Stetter R., Lamy E. (2018). Human T2R38 bitter taste receptor expression in resting and activated lymphocytes. Front. Immunol. 9:2949. 10.3389/fimmu.2018.02949 [Europe PMC free article] [Abstract] [CrossRef] [Google Scholar]
- Victoni T., Salvator H., Abrial C., Brollo M., Porto L. C. S., Lagente V., et al. (2017). Human lung and monocyte-derived macrophages differ with regard to the effects of beta2-adrenoceptor agonists on cytokine release. Respir. Res. 18:126. 10.1186/s12931-017-0613-y [Europe PMC free article] [Abstract] [CrossRef] [Google Scholar]
- Vrancic M., Banjanac M., Nujic K., Bosnar M., Murati T., Munic V., et al. (2012). Azithromycin distinctively modulates classical activation of human monocytes in vitro. Br. J. Pharmacol. 165 1348–1360. 10.1111/j.1476-5381.2011.01576.x [Europe PMC free article] [Abstract] [CrossRef] [Google Scholar]
- Yang M., Kumar R. K., Hansbro P. M., Foster P. S. (2012). Emerging roles of pulmonary macrophages in driving the development of severe asthma. J. Leukoc. Biol. 91 557–569. 10.1189/jlb.0711357 [Abstract] [CrossRef] [Google Scholar]
- Yasutomi M., Ohshima Y., Omata N., Yamada A., Iwasaki H., Urasaki Y., et al. (2005). Erythromycin differentially inhibits lipopolysaccharide- or poly(I:C)-induced but not peptidoglycan-induced activation of human monocyte-derived dendritic cells. J. Immunol. 175 8069–8076. 10.4049/jimmunol.175.12.8069 [Abstract] [CrossRef] [Google Scholar]
Articles from Frontiers in Physiology are provided here courtesy of Frontiers Media SA
Full text links
Read article at publisher's site: https://doi.org/10.3389/fphys.2019.01267
Read article for free, from open access legal sources, via Unpaywall:
https://www.frontiersin.org/articles/10.3389/fphys.2019.01267/pdf
Citations & impact
Impact metrics
Citations of article over time
Article citations
Intracellular TAS2Rs act as a gatekeeper for the excretion of harmful substances via ABCB1 in keratinocytes.
FASEB Bioadv, 6(10):424-441, 27 Aug 2024
Cited by: 0 articles | PMID: 39372126 | PMCID: PMC11452442
Relevance of Phytochemical Taste for Anti-Cancer Activity: A Statistical Inquiry.
Int J Mol Sci, 24(22):16227, 12 Nov 2023
Cited by: 2 articles | PMID: 38003415
Review
Bitter Phytochemicals as Novel Candidates for Skin Disease Treatment.
Curr Issues Mol Biol, 46(1):299-326, 30 Dec 2023
Cited by: 1 article | PMID: 38248322 | PMCID: PMC10814078
Review Free full text in Europe PMC
Transcriptome analyses reveal new insights on key determinants of perineural invasion in high-grade serous ovarian cancer.
Front Cell Dev Biol, 11:1109710, 20 Sep 2023
Cited by: 1 article | PMID: 37799274 | PMCID: PMC10548129
USNAP: fast unique dense region detection and its application to lung cancer.
Bioinformatics, 39(8):btad477, 01 Aug 2023
Cited by: 0 articles | PMID: 37527019 | PMCID: PMC10425186
Go to all (41) article citations
Similar Articles
To arrive at the top five similar articles we use a word-weighted algorithm to compare words from the Title and Abstract of each citation.
Bitter taste receptor agonists mediate relaxation of human and rodent vascular smooth muscle.
Eur J Pharmacol, 740:302-311, 15 Jul 2014
Cited by: 28 articles | PMID: 25036266
The expression and relaxant effect of bitter taste receptors in human bronchi.
Respir Res, 14:134, 22 Nov 2013
Cited by: 56 articles | PMID: 24266887 | PMCID: PMC4176101
The bitter taste receptor (TAS2R) agonist denatonium promotes a strong relaxation of rat corpus cavernosum.
Biochem Pharmacol, 215:115754, 18 Aug 2023
Cited by: 0 articles | PMID: 37597814
Bitter taste receptors on airway smooth muscle as targets for novel bronchodilators.
Expert Opin Ther Targets, 17(6):721-731, 12 Apr 2013
Cited by: 32 articles | PMID: 23577615 | PMCID: PMC4437536
Review Free full text in Europe PMC
Funding
Funders who supported this work.
Chancellerie des Universités de Paris (1)
Grant ID: Legs Gaston Poix