Abstract
Free full text

Recent progress of hypoxia-modulated multifunctional nanomedicines to enhance photodynamic therapy: opportunities, challenges, and future development
Abstract
Hypoxia, a salient feature of most solid tumors, confers invasiveness and resistance to the tumor cells. Oxygen-consumption photodynamic therapy (PDT) suffers from the undesirable impediment of local hypoxia in tumors. Moreover, PDT could further worsen hypoxia. Therefore, developing effective strategies for manipulating hypoxia and improving the effectiveness of PDT has been a focus on antitumor treatment. In this review, the mechanism and relationship of tumor hypoxia and PDT are discussed. Moreover, we highlight recent trends in the field of nanomedicines to modulate hypoxia for enhancing PDT, such as oxygen supply systems, down-regulation of oxygen consumption and hypoxia utilization. Finally, the opportunities and challenges are put forward to facilitate the development and clinical transformation of PDT.
Graphical abstract
Review of mechanisms and relationships of tumor hypoxia and photodynamic therapy as well as four nanomedicine delivery systems for manipulating tumor hypoxia to enhance the photodynamic therapy.
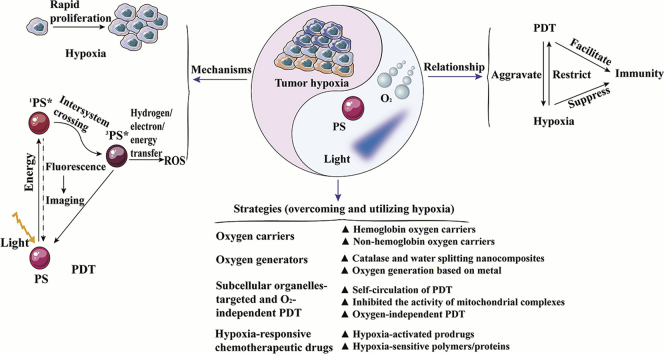
1. Introduction
Cancer remains the leading threat for human health on account of its high mortality, easy recurrence, high invasion and metastasis abilities. Although great efforts have been made in the last few years, it is still a long way to defeat cancer1,2. Among all the therapeutic regimens, the photodynamic therapy (PDT), clinically approved by the U.S. Food and Drug Administration (FDA), is one of the most effective options for treating neoplastic and non-malignant diseases3, 4, 5, 6. There are three essential components for PDT, including photosensitizer (PS), oxygen and light. Moreover, efficient singlet oxygen is produced when photosensitizers react with molecular oxygen under the irradiation of specific wavelengths of laser. The singlet oxygen causes damage to the tumor cells and vasculatures by apoptosis, necrosis and activating the immune responses7, 8, 9. Unfortunately, PDT still faces certain limitations, including the selection of effective photosensitizers, administration of light doses and the enormous limitation of tumor hypoxia10,11. Up to now, great strides and achievements have been made to conduct research on the photosensitizers and light doses12, 13, 14, 15. However, there are still many challenges ahead of us in modulating hypoxia for enhancing PDT.
Tumor hypoxia is characterized by a low oxygen concentration of tissues and rapid proliferation of neoplasms. It causes dysfunctional neovascularization by affecting the tumor microenvironment and facilitates tumor progression through cell mobility, invasion, and metastasis16, 17, 18. Additionally, treatment resistance also occurs in hypoxic tumors due to the production of hypoxia-inducible factors (HIF) and immune scaffolds. In PDT, molecular oxygen is consumed to exacerbate tumor hypoxia, which could lead to treatment failure and drug resistance19.
Over recent decades, nanocarriers have been developed for the tumor specific delivery of PSs based on the following advantages, such as the enhanced permeability and retention (EPR) effect and the selective tumor accumulation20,21. Consequently, the application of multifunctional nanomedicine delivery systems is promising to modulate hypoxia for improving PDT. This review highlights recent progress in nanomedicine delivery systems to manipulate hypoxia for enhancing PDT. We fall into three parts in this review. Firstly, the mechanism and relationship between tumor hypoxia and PDT are summarized. Additionally, we emphasize the nanomedicine delivery systems to modulate hypoxia for enhancing the effectiveness of PDT, including i) oxygen carriers, ii) intelligent oxygen generators in situ, iii) new paradigms of subcellular organelles-targeted and O2-independent PDT and iv) hypoxia-responsive chemotherapeutic drugs-involved PDT. Finally, we further discuss the opportunities and challenges to facilitate the clinical trials transformation of PDT.
2. Mechanism and cytolytic activity of oxygen-consumed PDT
2.1. Mechanism of oxygen-consumed PDT
When PS absorbs photons in light, it changes from the ground state to a transient singlet state (1PS). This unabiding singlet state has two alternative pathways. Firstly, the singlet state is converted back to the ground-state by emitting fluorescence or conversion with loss of energy as heat; secondly, the singlet state is changed into the excited triplet state (3PS
) with a long life time through the intersystem cross22,23. This triplet state could transfer energy to molecular oxygen by two reaction processes (types I and II)24. In the type I process, the radical species are formed by transferring hydrogen or electron when PSs react with the organic molecules directly. The radical species could also react with oxygen and produce various types of reactive oxygen species (ROS), such as superoxide anion (O2.−), hydroxyl radical (OH.), or hydrogen peroxide (H2O2)25. In the type II process, the PSs in the excited triplet state transfer energy to molecular oxygen (3O2) directly, leading to the production of highly active singlet oxygen. In PDT, these ROS could oxidize the subcellular organelles and destroy the vasculatures, resulting in the light-induced cell death26. The mechanism of PDT is shown in Fig. 1. It is obvious that PDT is an oxygen-consuming process that aggravates tumor hypoxia and leads to resistance. Therefore, it is important to address hypoxia in PDT.
2.2. Cytolytic activity of oxygen-consumed PDT
When photosensitizers are exposed to light, the generating ROS causes irreversible damage to the tumor cells and vascular systems, as well as triggers an inflammatory and immune response27. As shown in Fig. 2, tumors are ablated by PDT as the following reasons. Firstly, the singlet oxygen production by PDT could directly kill the tumor cells. The three main mechanisms of photodamage-induced cell death are necrosis, apoptosis, and autophagy. Necrosis is the primarily form of tumor cell death when high doses of PDT are located in the plasma membrane. Apoptosis could be triggered when the mitochondria are damaged by mild doses of PDT. Moreover, although autophagy tries to repair low-dose PDT-induced damage to organelles and cells, it also could induce cell death when its protective capacity is overwhelmed or malfunctioned. Secondly, hydrophobic photosensitizers loaded with nanomedical delivery systems are effectively accumulated in tumors due to leakage in the tumor blood vessels28. Simultaneously, owing to the structural characteristics of the photosensitizers, it exhibits an admirable affinity with the tumor endothelial cells and vascular endothelium. This partially shuts down the vascular system and augments hypoxia29. Thirdly, inflammation and immune responses are triggered by killing cancer cells and damaging blood vessels after treatment with PDT. It is beneficial to increase the activity of the antigen-presenting cells (APCs) and stimulate the secretion of cytokines and chemokines. Meanwhile, immune cells are recruited to amplify immunity, such as the macrophages, monocytes, neutrophils, dendritic cells, CD4+ and CD8+ cells30. Hence, PDT is an effective treatment modality to tumor ablation.
3. Mechanism and challenges of tumor hypoxia
3.1. Mechanism of tumor hypoxia
Hypoxia is a hallmark of most locally advanced tumors, which promotes tumor progression and has adversely impacts on the efficacy of different anticancer therapies due to its multiple contributions to chemo-/radio-resistance, invasiveness, metastasis, infiltration, altered tumor metabolism, and genomic instability31,32. As shown in Fig. 3, tumor hypoxia is mainly caused by differences in function and morphology between tumor and physiological blood vessels. Firstly, the tumor cells could not rationally coordinate with pro-angiogenic and anti-angiogenic factors, which results in abnormal vascular structure, increased vascular gap, and disordered blood flow33. Secondly, the distance among the tumor capillaries exceeds the diffusion range of oxygen due to disorder in the vascular networks34,35. Thirdly, transient and unstable blood flow in the tumor microvessels also limit the diffusion of oxygen, which further enlarges the region of tumor hypoxia. Moreover, these tumor cells with uncontrollable proliferation consume oxygen and nutrients from the normal vascular system because of dysfunction and high abnormalities of the blood vascular system, which further reduces the oxygen concentration of tumors36. In addition, the persistence of mild hypoxia in tumors induces the occurrence of severe or acute hypoxia and reoxidation37,38. In this regard, tumor cells adapt to the anaerobic microenvironment through the release of tumor HIF-139,40. The up-regulated hypoxia-inducible factor-1α (HIF-1α) also facilitates immunosuppression and immune escape by activating lymphocytes, myeloid-derived suppressive cells (MDSC), dendritic cells (DC), and tumor-associated macrophages (TAM)41.
3.2. Challenges of tumor hypoxia
Tumor hypoxia refers to the zone of solid tumors with an oxygen pressure of less than 10 mm Hg, which is significantly lower than normal tissues (40–60 mm Hg). In addition, radiation therapy is resistant when the oxygen concentration is less than 20 mm Hg42. However, the differences in photosensitizers, light doses, and the type of tumors could affect the oxygen demand during PDT. In addition, different determination methods also have influence on the specific values of oxygen demand during PDT. Currently, there are three strategies for evaluating the degree of tumor hypoxia. Firstly, the Eppendorf oxygen electrode is used to evaluate the oxygen concentration, which is the gold standard for determining local oxygen pressure. Secondly, the degree of tumor hypoxia is evaluated by monitoring endogenous markers, such as HIF1α, HIF2α, carbonic anhydrase 9, osteopontin, lysyl oxidase and hypoxic gene signature. Thirdly, oxygen concentrations are confirmed using exogenous probes, such as pimonidazole and etanidazole pentafluoride. Recently, 18F-labeled nitroimidazole compounds based on positron emission tomography (PET) have also been reported to be useful for the evaluation of tumor hypoxia during PDT43. Hypoxia contributes to treatment resistance, heterogeneity, invasion and metastasis of tumors through regulation of various mechanisms44. In particular, hypoxia is the main stumbling block of PDT which requires oxygen to produce ROS45,46. The specific reasons are listed as follows. Firstly, the hypoxic cells are inadequately exposed to PDT due to a certain distance from the blood vessels. Meanwhile, the expression of hypoxia-associated regulatory genes (such as gene encoding P-glycoprotein) and proteins (such as HIF-1α) facilitates the emergence of multi-drug resistance and further impedes PDT47,48. In addition, hypoxia interferes with the physiological immune regulation and promotes variation and expansion of the immune-suppressed stromal cells49,50. To sum up, hypoxia restricts PDT and inhibits immune response, as well as strengthens tumor invasion and metastasis. Thus, tumor hypoxia confers great challenges on the development of PDT and the treatment of tumors.
4. Nanomedicines for overcoming and utilizing hypoxia in PDT
Currently, nanomedicine delivery systems provide a promising option towards enhancing tumor-selective delivery, especially in photodynamic therapy and combination therapy. Herein, we attempt to offer the current state-of-the-art regarding nanomedicine strategies for manipulating hypoxia and promoting PDT. Hence, multifunctional nanomedicine delivery strategies are reviewed, including oxygen supply systems (oxygen carriers and generators), down-regulation of oxygen consumption and O2-independent strategies (subcellular organelles-targeted and O2-independent PDT), as well as hypoxia utilization (hypoxia-responsive chemotherapeutic drugs-involved PDT). These strategies not only play an important role in addressing hypoxia in PDT, but also emphasize the importance of this review in PDT and combination therapy. The specific strategies are shown in Table 151, 52, 53, 54, 55, 56, 57, 58, 59, 60, 61, 62, 63, 64, 65, 66.
Table 1
An overview of strategies for addressing tumor hypoxia in PDT, including nanocarriers, typical cases and functions, advantages, disadvantages and related references.
Strategy | Nanocarrier | Typical case and function | Advantage | Disadvantage | Ref. |
---|---|---|---|---|---|
Oxygen carriers | |||||
Hb oxygen carriers | PLGA nanoparticle | Artificial RBCs (composed of lecithin, DSPE-PEG and PLGA) loaded Hb and ICG for oxygen supply | Excellent biocompatibility and safety | Limited oxygen loading efficiency and release | 51 |
HSA and Hb hybrid compound | HSA and Hb hybrid protein loaded with oxygen, doxorubicin (DOX) and Ce6 for oxygen supply | 52 | |||
Non-Hb oxygen carriers | Cholesterol, DSPE-PEG2000 and lecithin lipid monolayer | Lipid monolayer loaded IR780 and PFC for oxygen supply and transportation | 53 | ||
MoSx nanoparticle | HSA and chloroaluminum phthalocyanine modified and PFH loaded hollow MoSx nanoparticles for imaging and photodynamic and photothermal therapy | 54 | |||
Oxygen generators | |||||
Catalase and water splitting | Imidazolate framework (ZIF-8) | Cancer membrane-coated and catalase and AlPcS4 loaded ZIF-8 nanoparticle for homologous targeting and oxygen generation | Utilize excess H2O2 in tumors | Uneven oxygen production and limited oxygen generation efficiency | 55 |
TiO2 nanotubes | Carbon-nanodot-modified TiO2 nanotubes for PDT and water splitting | 56 | |||
Oxygen generation based on metal | PCLA-PEG-PCLA copolymer | PCLA-PEG-PCLA loaded DOX, Ce6 and colloidal MnO2 for oxygen generation and imaging | 57 | ||
PAMAM polymer | Amino-terminal PAMAM polymer-coated gold nanoclusters for H2O2 degradation | 58 | |||
Liposome | Liposome loaded methylene blue (MB) and CaO2 for PDT and oxygen generation | 59 | |||
Subcellular organelles-targeted and O2-independent PDT | |||||
Self-circulation | Gold nanospheres and liposome | FAL peptides modified and ICG conjugated-hollow gold nanospheres together with liposome loaded Hb for endoplasmic reticulum targeting and immunotherapy | No need additional oxygen supply | Complicated chemical synthesis and manufacturing processes | 60 |
Inhibitors of mitochondrial complexes | Liposome | Liposome co-encapsulated hydrophobic HCe6 and metformin for inhibiting mitochondrial complexes I | 61 | ||
Gelatin nanoparticle | Gelatin nanoparticle loaded ICG-BSA and atovaquone for inhibiting mitochondrial complexes I–III | 62 | |||
O2-independent PDT | Phthalocynine nanostructures | Phthalocynine-triethylene glycol-biotin nanostructures for synergy of PDT and photothermal therapy | 63 | ||
Hypoxia-responsive chemotherapeutic drugs-involved PDT | |||||
Hypoxia-activated prodrugs | Liposome | Liposome loaded AQ4N and64Cu-hCe6 for hypoxic activation and PDT | Utilize the hostile tumor hypoxic environment | Limited numbers of hypoxia activated prodrugs for clinical application | 64 |
LASA complex | LASA loaded ENAB and TPZ for hypoxic activation and PDT | 65 | |||
Hypoxia-sensitive polymers/proteins | Polyvinylpyrrolidone (polymer) | Azobenzene bridged and polymer nanocarriers loaded Ce6 and camptothecin for hypoxia utilization | 66 |
4.1. Oxygen carriers—“Trojan effect”
Oxygen carriers could preserve and release a large amount of oxygen for PDT consumption, forming a “Trojan effect”. The oxygen carriers are divided into hemoglobin (Hb)- and non-Hb-based carriers. Non-Hb oxygen carriers are fluorocarbons.
4.1.1. Hb oxygen carriers
Hb, a ferritin in the red blood cells (RBCs), is able to transport oxygen to tissues67. This inherent oxygen carrier has admirable application prospects. However, some shortcomings limit the development of dissociative Hb, such as short circulation time, poor stability and potential adverse effects68. Thus, Hb-based nanotechnology has been developed to overcome these disadvantages of dissociative Hb. For instance, Luo et al.51 developed a nanoscale artificial red cell (composed of lecithin, DSPE-PEG and PLGA) loading Hb and photosensitizers (indocyanine green, ICG) to enhance oxygen supply and the effect of PDT. In this study, plenty of oxygen deriving from Hb promoted the formation of ROS by PDT and Hb was oxidized by ROS into the more cytotoxic ferryl-hemoglobin that synergized with PDT to antitumor. Interestingly, dynamic self-monitoring could be achieved during PDT through the fluorescence of ICG and the photoacoustic response of Hb and ICG. Besides, Hb-loaded copolymer nanomicelle was also been studied69, which also held the potential for treatment tumor hypoxia.
Moreover, the hybridization of tumor-targeted human serum albumin (HSA) with oxygen carrier Hb was effective in loading oxygen, chemotherapeutic drugs doxorubicin (DOX) and chlorin e6 (Ce6, Fig. 4). The presence of HSA and Hb guaranteed the precise oxygen retention and release in tumor sites, which induced oxygen interference and increased the degree of tumor oxidation. In addition, in vitro cell assays demonstrated that the amount of oxygen carried by Hb down-regulated the expression of HIF-1α, multidrug resistance 1 (MDR1) and P-glycoprotein, which disrupted the DOX resistance and increased DOX accumulation in tumors52. Subsequently, this research group further demonstrated the anti-tumor effect of hybrid Hb from the perspective of immunology70. The results showed that the hybrid protein of HSA and Hb loaded with Ce6 was able to enhance the infiltration of CD8+ T cells in tumors and induce immunogenic cell death, as well as activate immune response, such as activation of DC, NK cells and T lymphocytes. Hence, this strategy could suppress distant tumors and lung metastases of triple-negative breast cancer70. Compared to the other PDT combined therapies (such as the combination of PDT and immunological adjuvants or immunological checkpoint inhibitors)71, 72, 73, this hybrid protein oxygen carrier had the advantages of low cost and active targeting. Meanwhile, HSA and Hb, as inherent endogenous proteins, exhibited excellent safety and biocompatibility compared to exogenous vehicles, which contributed to the widespread application of the oxygen carriers. However, further enhancing the immune effect of the oxygen carrier may increase tumor ablation. In addition, the design of cascade-responsive oxygen carriers may be conducive to enhance the synergistic anti-tumor effect of PDT and combination therapy.
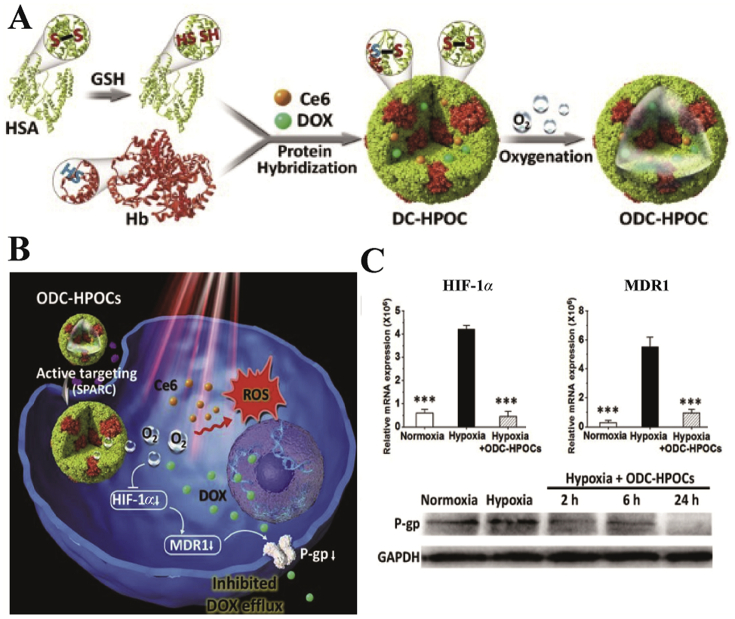
(A) and (B) Schematic diagram of the formation and mechanism of Hb oxygen carriers. (C) mRNA levels of HIF-1α and MDR1, and protein expression levels of P-gp. Reprinted with the permission from Ref. 52. Copyright© 2019 Ivyspring International Publisher.
In conclusion, Hb as oxygen carrier not only provides sufficient oxygen for PDT but also augments tumor-targeted activation of the immune response. These multifunctional nanomedicine delivery vehicles overcome the hypoxia-induced drug resistance and exert strong anti-tumor effects.
4.1.2. Non-Hb oxygen carriers
Perfluorocarbon (PFC) is chemically inert molecule consisting of carbon and fluorine atoms. Meanwhile, PFC as artificial blood substitute is widely used in the field of biomedicine for oxygen delivery due to its high oxygen-affinity, oxygen-carrying capacity, and excellent biocompatibility74, 75, 76. Therefore, PFC-based self-enriching oxygen carriers are presented for enhancing the efficacy of oxygen-consumed PDT. The representative PFC nanodroplets were designed by Hu's group53. They loaded the photosensitizer IR780 into a lipid monolayer (composed of cholesterol, DSPE-PEG2000 and lecithin) together with the PFC in nanodroplets, which made the photosensitizer more stable53. Furthermore, this PFC nanodroplet elevated the oxygen concentration for PDT and increased the lifetime of singlet oxygen and the level of reactive oxygen, thereby enhancing the cytotoxicity. In vivo studies showed that the photodynamic effect of this PFC nanodroplet was better than the group with non-perfluorocarbon and the traditional PDT due to the oxygen supply, indicating that PFC could overcome hypoxia and enhance the effect of PDT significantly. To further promoted the release of oxygen, Song et al.77 developed a PFC (perfluoro-15-crown-5-ether) nanodroplet stabilized by HSA and injected intravenously into tumor-bearing mice under respiratory hyperoxia. PFC nanodroplet carried a large amount of oxygen to tumors after passing through the lungs and increased the tumor oxidation under the trigger of local low-frequency ultrasound. This process released sufficient oxygen for PDT and increased tumor destruction. Finally, the modified nanodroplet could recycle back to lungs for reoxygenation due to continuous hyperoxic inhalation. Although this PFC nanodroplet shows high affinity towards oxygen and enhances PDT, it still has the following disadvantages. Firstly, the experimental operation based on the PFC nano-formulation is complicated because this strategy requires additional oxygen supply. Secondly, it is necessary to explore the sequential administration time of nano-PFC system and inhalation of oxygen to ensure the maximum efficacy of PDT. Therefore, it is important to develop a convenient and efficient drug delivery system.
Perfluorohexane (PFH), a liquid fluorocarbon with good biocompatibility, can carry more oxygen. Wang et al.54 utilized HSA and chloroaluminum phthalocyanine as modifiers to prepare multifunctional PFH and hollow MoSx-based nanoparticles for triple-modal imaging and photodynamic/photothermal synergistic anti-tumor. The results demonstrated that the oxygen-saturated PFH nanoparticles were capable of producing sufficient oxygen to overcome tumor hypoxia for enhancing PDT54. Moreover, triple-modal imaging also conferred the function of tumor diagnosis of the nano-formulation. Importantly, due to the presence of photosensitizer and enough oxygen, this PFH nano-formulation overcame the disadvantages of PFC nanodroplet. Overall, these self-enriching oxygen carriers based on fluorocarbon could increase tumor oxidation and enhance photodynamic effects (Fig. 5).
4.2. Intelligent oxygen generators—“self-sufficiency”
Oxygen generators react with hydrogen peroxide or water generating oxygen through a “self-sufficient” process. The enzyme- and water-splitting oxygen generators involve catalase and carbon nanodot. In addition, the metal-based oxygen generators include manganese (II), manganese dioxide (MnO2), calcium dioxide (CaO2), gold nanoclusters, platinum (IV) prodrugs, and cerium oxide (CeO2). Fig. 6 shows the mechanism of intelligent oxygen generation.
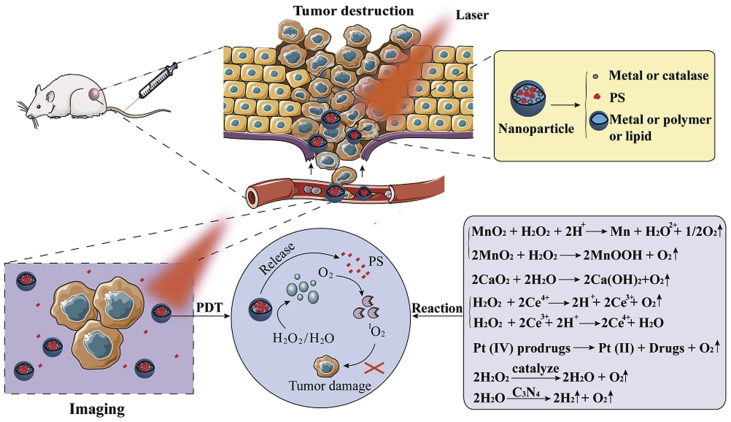
Imaging and PDT functions of oxygen generators. The specific mechanisms of oxygen generation are represented by equations.
4.2.1. Catalase and water splitting nanocomposites
Up till now, catalase-based and water-splitting oxygen generators have drawn widely attention in the treatment of tumors. These generators catalyze hydrogen peroxide (H2O2) and water (H2O) to generate oxygen for enhancing PDT. For example, Li et al.78 conjugated the photosensitizer (Ce6) with catalase by amide bond and formed intravesical instillation-based co-assembled nanoparticles through the non-covalent interaction with fluorinated polyethyleneimine. This nanoparticle exhibited excellent transmembrane, transmucosal and intratumoral permeability since the introduction of fluorinated polyethyleneimine. Fluoroalkyl could dramatically reduce the retention of fluoroamphiphiles on the cell membrane, which was beneficial to tumor accumulation of photosensitizers and catalase. Importantly, the catalase-containing nanoparticles could catalyze the decomposition of tumor endogenous H2O2, thereby effectively increasing the oxidation level of tumors and the antitumor effect of PDT. In addition, the intravesical instillation of nanoparticles markedly weakened the systemic toxicity of photosensitizer in comparison with the hemoporphyrin—the first-line medication for bladder cancer in clinic. Although the fluorinated nanoparticles had numerous merits, the use of organic reagent (dimethyl sulfoxide) in the preparation of the nanoparticles inevitably affected the bioactivity of catalase.
In a similar study, the application of the imidazolate framework (ZIF-8) could eliminate the effects of organic reagents. Cheng et al.55 proposed cancer membrane-coated nanoparticles based on 2-methyl imidazole and Zn2+ metal organic framework (ZIF-8). Moreover, catalase and photosensitizer (AlPcS4) were embedded into the nanoparticles. Notably, ZIF-8, the carrier of catalase and photosensitizers, could be engineered in aqueous solution, which avoided the inactivation of catalase in organic reagents. In addition, the nanoparticles had homologous targeting properties and immunological functions because of cancer cell membranes in the outer shell (ZIF-8) of nanoparticles. Recently, HSA-based self-assembled nanoparticles were developed to co-deliver paclitaxel, Ce6 and catalase79. In this design, nanoparticles exhibited high tumor targeting and catalase could decompose H2O2 to relieve hypoxia, which also enhanced tumor oxygenation and increased the suppression of tumors. Moreover, Zhang's group80 designed a cancer-targeted nanoparticles for synergistic starvation and PDT, which embedded glucose oxidase and catalase into the cancer cell membrane and encapsulated the porphyrin metal organic framework (MOF). Due to the biomimetic surface, the nanoparticles could homologous target to tumors and increase retention in tumors. After being internalized by tumor cells, the catalase degraded the H2O2 to generate oxygen and accelerated the decomposition of glucose oxidase, which achieved synergistic tumor cell killing. This strategy inspired more ideas for the application of PDT and combination therapy80,81.
In addition to catalase, water splitting nanocomposites could also generate oxygen for potentiating the efficiency of PDT82. Yang et al.56 synthesized carbon-nanodot-modified TiO2 nanotubes for PDT. The nanotubes overcame low efficiency and instability of the inorganic–organic hybrid materials in PDT because the TiO2 had good photostability and high catalytic efficiency. Moreover, the introduction of carbon nanodots reduced the band gap and transferred electrons to the surface of TiO2 in order to stabilize the nanoparticles and increase the photosensitization effect. Especially, the carbon nanodots performed an efficient water decomposition reaction to relieve tumor hypoxia and provided sufficient oxygen for PDT.
4.2.2. Oxygen generation based on metal
Manganese (II) and MnO2, the components of the classic oxygen generators, can effectively decompose intracellular H2O2 to generate oxygen and alleviate hypoxia of PDT83,84. In addition, manganese (II) and MnO2 also possess the properties of nuclear magnetic resonance (NMR) bioimaging. The oxygen generation based on metal nanoparticles enhances PDT and diagnosis. Jia et al.85 used manganese (II) phthalocyanine as a precursor to synthesize the magnetofluorescent manganese-carbon dots (Mn-CDs) multifunctional nanoparticles. Subsequently, the authors modified DSPE-mPEG on the surface of Mn-CDs by hydrophobic–hydrophobic interaction to increase the water solubility and biocompatibility of Mn-CDs. Interestingly, this nanoparticle platform had properties of near-infrared fluorescence and T1-weighted magnetic resonance imaging due to the presence of Mn. In addition, Mn was able to efficiently catalyze H2O2 into oxygen under acidic conditions. Moreover, the sufficient oxygen facilitated carbon dots to generate ROS for PDT.
Similarly, MnO2-based nanomedicine delivery systems have gained increasing attention. For instance, Hu et al.57 utilized copolymer (PCLA-PEG-PCLA) preparing nanoparticles through W/O/W emulsification solvent volatilization and hierarchically loaded with DOX, Ce6 and colloidal MnO2. The multi-layered encapsulation showed good stability and biocompatibility. Tumor hypoxia was alleviated since endogenous H2O2 could be decomposed by MnO2. Most importantly, oxygen produced by H2O2 could eliminate the multi-drug resistance-mediated drug resistance of DOX and significantly increase the anti-tumor effect of chemo-phototherapy. In addition, the imaging function of MnO2 endowed more opportunities for the therapeutic diagnosis and tracking of cancer57. Besides, Liu's group86 designed pH/H2O2-responsive nanoparticles, which successfully coated MnO2 nanoclusters onto the albumin-drug complexes (chlorine e6 and cis-platinum prodrugs conjugated HSA) through biomineralization process. The nanoparticles could be gradually degraded into different albumin–drug complexes with small sizes (<10 nm), which significantly enhanced intratumoral infiltration and antitumor effect. And then, the nanoparticles could be decomposed by H2O2 inside the tumors after permeating into the tumors and generate oxygen in situ. Consequently, the intelligent pH/H2O2-triggered PDT could reduce the damage of blood vessels and improve cancer therapy86.
Except for manganese compounds, gold nanoclusters, CaO2 and CeO2 nanoparticles, platinum (IV) prodrugs are well-studied oxygen generators58,59,87,88. For example, Lin's group58 showed that the amino-terminal PAMAM polymer-coated gold nanoclusters had catalase-like activity, which could catalyze the decomposition of H2O2 into oxygen, thereby inhibiting the growth of hypoxic tumor cells. The specific mechanism could be due to the protonation of the abundant tertiary amines in the dendrimer under acidic conditions, which promoted the pre-adsorption of hydroxy groups on the metal surface and acted as catalase. Surprisingly, the gold nanoclusters exhibited catalase-like activity under a wide range of pH conditions, especially under physiological and acidic conditions. This extensive pH adaptability conferred broader application prospects to gold nanoclusters than catalase. Furthermore, CaO2 is widely used due to its high bioavailability, biodegradability and oxygen production. Liu et al.59 reported that methylene blue (MB) and CaO2 could release from the damaged liposomes since induced lipid peroxidation after laser irradiation. This process increased the contact area of CaO2 and H2O, as well as promoted the formation of oxygen, which improved the tumor hypoxia microenvironment.
4.3. New paradigms of subcellular organelles-targeted and O2-independent PDT—“take away the firewood from under the cauldron”
Recently, reducing oxygen consumption and O2-independent therapy are promising for overcoming hypoxic microenvironment and improving PDT. Strategies to reduce oxygen consumption are mainly through targeting subcellular organelles, including plasma membrane, mitochondria and lysosomes. Mitochondria, the primary subcellular organelle of oxidative phosphorylation, play an important role in oxygen consumption. Moreover, endoplasmic reticulum and golgi apparatus-targeted PDT also exhibit impressive anti-tumor effects. The strategies are as follows: 1) self-circulation of PDT: the subcellular organelles-targeted photosensitizers could reduce the consumption of oxygen and provide sufficient oxygen for PDT, forming a good self-circulating state; 2) inhibiting the activity of mitochondrial function: some mitochondrial respiratory chain inhibitors are used to suppress mitochondrial complexes I–IV, leading to mitochondrial dysfunction and down-regulation of oxygen consumption; 3) O2-independent PDT. The mechanism of mitochondrial targeting nanoparticles is shown in Fig. 7.
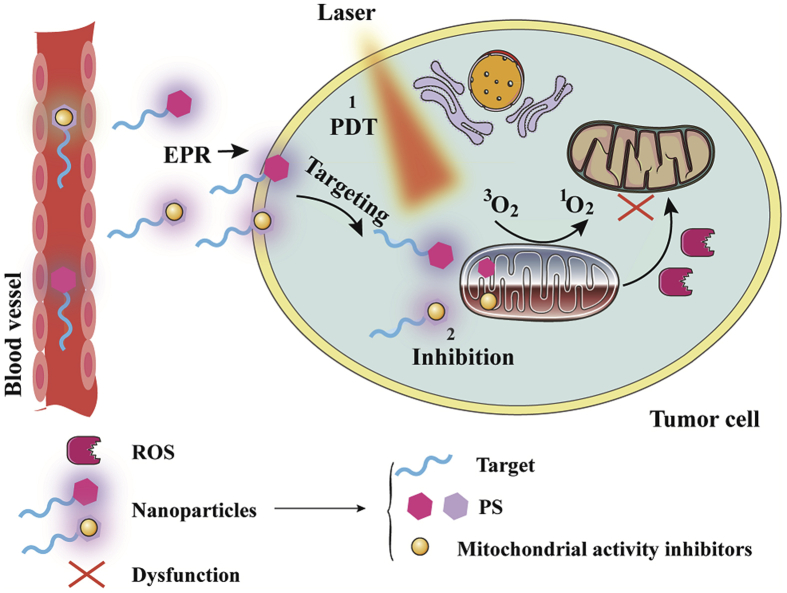
Schematic of the mechanism of mitochondrial targeting nanoparticles. The mitochondria-targeted nanoparticles are mainly divided into two types, one is the nanoparticle containing metal photosensitizer, and the other is the nanoparticle including photosensitizer and mitochondrial activity regulator.
4.3.1. Self-circulation of PDT
Photosensitizers based on iridium (III) and Ru(II) complexes are capable of selectively targeting mitochondria and lysosomal for PDT89, 90, 91. Recently, an iridium-based photosensitizer showed that not only could target mitochondria but also could reduce the mitochondrial respiration rate, which increased intracellular oxygen concentration92. Lv et al.92 designed and synthesized two compounds based on iridium (III). They bridged the iridium (III) compound and triphenylphosphine with 1,6-dibromohexane for mitochondria targeting (Ir-P (ph)3) and a triphenylphosphine-free compound (Ir-alkyl) for lysosomal targeting. Then, the light absorption properties of two compounds were verified, demonstrating their utility for PDT. It was worth noting that the authors evaluated the phototoxicity and rate of oxygen consumption of the two compounds. Among them, the results of cytotoxicity showed that Ir-P (ph)3 had stronger tumor cell killing. In addition, photobleaching experiments of living cells demonstrated that the cellular uptake mechanism of the compounds was energy-dependent endocytosis. Finally, the PDT effects of the two compounds were conducted under hypoxia and normoxia. The results showed that Ir-P (ph)3 could enhance PDT even under hypoxia, the main reason was that Ir-P (ph)3 inhibited mitochondrial oxidative respiration and provided more oxygen for PDT92.
In addition, Zhang's group93 designed a bioactive peptide and photosensitizer (protoporphyrin IX) conjugated nanoparticle for targeting mitochondria and plasma membrane in PDT. Since the nanoparticle could target the vascular membrane, the membrane permeability and cellular uptake of the nanoparticles were increased. Meanwhile, the chimeric peptide was able to target mitochondria, which significantly reduced the membrane potential of mitochondria and increased cell apoptosis. In vitro and in vivo experiments indicated that the combinatorial PDT had a remarkable therapeutic effect and reduced side effects due to the dual-targeted function93.
Moreover, endoplasmic reticulum and golgi apparatus control the processing and synthesis of proteins, which can also be used as therapeutic targets for PDT94. Li et al.60 applied pardaxin (FAL) peptides for endoplasmic reticulum-specific localization and designed endoplasmic reticulum-targeted and ICG conjugated-hollow gold nanospheres for photodynamic and photothermal immunotherapy. Meanwhile, liposome-loaded Hb and FAL-Hb were used to reverse tumor hypoxia. Under laser irradiation, the nanosystems were capable of inducing intense endoplasmic reticulum stress and exposure of calreticulin on the cell surface, as well as activating a series of immune responses60. In summary, the endoplasmic reticulum-targeted nanosystem not only reversed tumor hypoxia but also increased the immune effect in photodynamic and photothermal therapy. In addition, golgi-targeted PDT had also developed. Villanueva and colleagues95 demonstrated that the photosensitizer zinc(II)-phthalocyanine could target golgi for the synergistic treatment of PDT. Studies showed that the photosensitizer was able to induce the activation of golgi caspase-2 in A-549 cells and trigger apoptosis. Therefore, endoplasmic reticulum and golgi-targeted PDT could effectively inhibit tumors.
4.3.2. Inhibiting the activity of mitochondrial complexes
Metformin, a clinically approved oral hypoglycemic agent, can be used to alleviate tumor hypoxia96,97. Song et al.61 reported a liposome that co-encapsulated hydrophobic HCe6 (Ce6 was covalently linked to hexamine) and hydrophilic metformin into the outer membrane and inner cavity of the liposome. Due to the membrane-like properties of liposome and the presence of PEG, this nanoparticle was capable of long-circulating in vitro and in vivo. Importantly, continuous release of metformin could inhibit the activity of complex I in the mitochondria oxidative respiratory chain and reduce tumor oxygen consumption. Therefore, Ce6 utilized the remaining oxygen to generate ROS and inhibited tumor growth. In addition, the liposome showed excellent anti-tumor effects both in CT26 and SCC-7 tumor models, which demonstrated that the combination of mitochondrial complex modulators and PDT overcame the limitations of hypoxia on PDT.
Atovaquone, another typical mitochondrial complexes regulator, affects oxidative phosphorylation by inhibiting mitochondrial complexes I–III98. Xia et al.62 loaded the ICG-BSA nanocomposite and atovaquone into gelatin nanoparticles (Fig. 8). Among them, BSA delayed the release of ICG. In addition, matrix metalloproteinase 2 was highly expressed in tumors and effectively degraded the gelatin nanoparticles, which increased the penetration of nanoparticles into hypoxic tumors. Specifically, atovaquone inhibited electron transfer in the oxidative respiratory chain and maintained mitochondrial membrane potential, ultimately sparing oxygen. While metformin inhibited mitochondrial complex I, atovaquone was more effective in sparing oxygen because atovaquone inhibited mitochondrial complexes I–III. In vivo anti-tumor experiments further validated the significant anti-tumor effect of the nanoparticles62. Similarly, the photosensitizer verteporfin and atovaquone co-loaded nanoparticles could also inhibit the electron transfer of mitochondrial respiratory chain and enhance PDT99. Noteworthy, the size of this nanoparticle was below 50 nm, which facilitated the deep permeation into tumors. In addition, verteporfin could target tumor cells through the transport of lipoprotein.
4.3.3. Oxygen-independent PDT
Traditional PDT relies on oxygen to generate toxic ROS, whereas tumor hypoxia severely limits the therapeutic effects of PDT. Therefore, oxygen-independent PDT is a powerful strategy to overcome the therapeutic limitations of PDT. In recent years, the combination of photothermal and photodynamic therapy and the emergence of free radicals have become promising candidates for hypoxic tumor therapy100,101. For instance, Li et al.63 fabricated a versatile nanoparticle with photothermal, photodynamic, and photoacoustic imaging properties (consisting of phthalocynine-triethylene glycol-biotin), which generated fluorescence and ROS after partial degradation reactions triggered by biotin receptors. Due to the potential of both photodynamic and photothermal treatment, the nanoparticles were capable of inhibiting tumor growth after systemic administration.
In addition, Zhang's group102 proposed an intelligent free radicals generator, which could ablate tumors without the limitation of tumor hypoxia. This nanosystem modified mitochondrial targeting ligands (triphenylphosphonium), thermal initiators (V057), multifunctional peptides and fluoresceinamine on the surface of gold nanocage. Among them, the fluoresceinamine could be used for real-time imaging. Importantly, due to the presence of mitochondrial targeting ligands, the nanosystems could efficiently target mitochondria and release free radicals in mitochondria, which reduced mitochondrial membrane potential and induced apoptosis of cancer cells.
4.4. Hypoxia-responsive chemotherapeutic drugs-involved PDT—“turn corruption into wonder”
The emergence of hypoxia-responsive chemotherapeutic drugs provides new insight into enhancing the efficacy of oxygen-dependent PDT. This way of utilizing the adverse environment (such as hypoxia) to kill tumor cells is referred to as “turn corruption into wonder”. Hypoxia-responsive chemotherapeutic drugs are divided into hypoxia-activated prodrugs and polymers/proteins, respectively.
4.4.1. Hypoxia-activated prodrugs
Hypoxia-activated prodrugs can be transformed into therapeutic agents by various intracellular reductases under hypoxia103, 104, 105, 106. There are many well-studied hypoxia-activated prodrugs, such as AQ4N, TPZ, TH-302, PR-104A, NLCQ-1 and SN 2386264,107. The mechanism of activation of these hypoxia-activated prodrugs is shown in Fig. 9. However, the use of hypoxia-activated prodrugs alone exerts unsatisfactory antitumor effects since tumors show hypoxic heterogeneity. Interestingly, PDT aggravates tumor hypoxia and promotes the bioactivation of hypoxia-activated prodrugs. Therefore, more attention has been paid to the synergistic effect of hypoxia-activated prodrugs and PDT on antitumor. Feng et al.64 conjugated the amino group of hexadecylamine with the carboxyl groups of Ce6 forming a hydrophobic photosensitizer (hCe6). Additionally, the isotope 64Cu was chelated with the hCe6 (64Cu-hCe6) for tumor imaging. Subsequently, the hypoxia-activated prodrug banoxantrone (AQ4N) and 64Cu-hCe6 were loaded into the hydrophilic cavity and hydrophobic layer of the liposome, respectively. In vivo fluorescence and imaging results showed that the liposomes exhibited potent passive homing ability after intravenous injection due to the EPR effects. Importantly, laser-induced PDT led to severe tumor hypoxia, which activated AQ4N. Non-toxic AQ4N was reduced to AQ4 with antitumor effects under hypoxic conditions64.
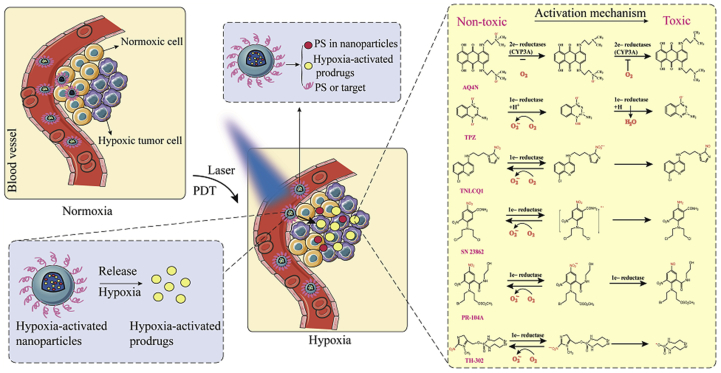
Activation mechanism of hypoxia-activated prodrugs. Under laser irradiation, the photosensitizer produced PDT that aggravated tumor hypoxia and activated hypoxia-activated prodrugs. Non-toxic hypoxia-activated prodrugs were reduced to toxic molecules to damage tumor cells.
In addition to AQ4N, tirapazamine (TPZ) is also a commonly used hypoxia-activated prodrug108, 109, 110. Chen et al.65 encapsulated the acid-sensitive photosensitizers ENAB and TPZ into LASA (a complex of linoleic acid and stearyl alcohol) forming hydrophilic nanoparticles (TENAB). Under 808 nm laser irradiation, the LASA material melted and released the hypoxia-activated prodrugs. Meanwhile, in the acidic environment of the tumors, the photosensitizer ENAB generated significant ROS and synergized with photothermal therapy. In addition, PDT aggravated tumor hypoxia and activated TPZ. Activated TPZ could increase tumor cell killing. Ultimately, photodynamic therapy, photothermal therapy, and hypoxia-activated prodrugs worked synergistically. It was worth noting that the nanoparticles had negligible toxicity to normal tissues and skin because of the excellent biocompatibility of LASA and the highly-selective tumor acid-sensitive photosensitizer. Moreover, Wang et al. fabricated an iRGD (a dual targeting ability cyclic peptide with targeted tumor neovascularization and tumor cells) modified nanoparticles that loaded ICG and TPZ for enhancing penetration and anti-tumor. Due to the active targeting of iRGD, the nanoparticles showed significantly increased penetration both in vitro and in vivo. Importantly, PDT could activate TPZ for synergistic anti-tumor because of amplifying tumor hypoxia. Similarly, Li et al.111 loaded TPZ with a porphyrin MOF and coated the cancer cell membrane on the surface of the nanoparticles. The nanoparticles were able to selectively accumulate in tumors. Meanwhile, PDT aggravated tumor hypoxia further activated TPZ. The combination of PDT and hypoxia-activated prodrugs could suppress tumors.
4.4.2. Hypoxia-sensitive polymers/proteins
In addition to the hypoxia-activated prodrugs, the combination of synthetic hypoxia-sensitive polymers/proteins with PDT has recently evolved. Hypoxia-sensitive polymers/proteins are formed primarily by conjugating the hypoxia-sensitive groups (azobenzene, nitro groups, quinones, aromatic N-oxides, aliphatic N-oxides and transition metals112,113) to polymers/proteins. Then, these polymers/proteins utilize the hypoxic microenvironment of tumors to cleave the hypoxia-sensitive groups and release the chemotherapeutic drugs and photosensitizers. These hypoxia-triggered PDT achieve accurate drug release in tumor tissues, which reduces vessel damage and systemic toxicity. For instance, Zhang et al.66 reported that a hydrophobic photosensitizer (Ce6) and chemotherapeutic drug (camptothecin) loaded polymer nonocarriers containing azobenzene linkers for synergistic antitumor. Among them, Ce6 and camptothecin were released from polymers after cleaving azobenzene group in the tumor hypoxic environment. Meanwhile, the nanoparticles effectively produced ROS and aggravated tumor hypoxia under laser irradiation to promote the release of camptothecin, showing a remarkable synergistic anti-tumor effect.
Furthermore, the discovery of protein-based hypoxia response systems improved the therapeutic depth and hypoxia resistance behavior of PDT. Yang et al.114 synthesized a size-switchable nanoparticle. Firstly, the photosensitizer Ce6 was covalently linked to HAS (HC). Then, chemotherapeutic drug oxaliplatin was conjugated with HAS (HO). Finally, hypoxia responsive azobenzene linker bridged HC with HO forming nanoparticles (HCHOA, Fig. 10). Interestingly, this nanosystem was stable in the normoxic environment with a particle size of 100–150 nm. However, it was decomposed into nanoparticles with a diameter of <10 nm in the hypoxic environment, showing superior tumor permeability. Moreover, although Ce6 was quenched during the preparation process, the fluorescence could be recovered to enhance PDT when the nanoparticles were decomposed. The antitumor results showed that the nanoparticles had significant anti-tumor effects.
5. Outlook
Tumor hypoxia is one of major restrictions when applying PDT. Hypoxia causes poor prognosis, resistance, malignant progression and metastasis of most tumors. Additionally, hypoxia also recruits tumor-suppressed stromal cells inhibiting tumor immunity. PDT is a non-invasive and effective tumor treatment modality by electron or energy transfer for a promising tumor therapy. However, PDT is an oxygen-consuming process, which aggravates tumor hypoxia. Therefore, it is important to understand the mechanisms and challenges of hypoxia and PDT, as well as develops efficient drug delivery strategies for tumor ablation. The application of nanomedicine delivery systems is a superior technique to modulate hypoxia for enhancing PDT. Hence, we summarize recent effective strategies to manipulate tumor hypoxia for enhancing PDT, such as oxygen carriers, oxygen generators, subcellular organelles-targeted and O2-independent strategies, as well as hypoxia-responsive chemotherapeutic drugs. All these approaches facilitate oxygenation of tumor cells and increase the tumor destruction. Especially, multifunctional and intelligent nanoparticles act as drug carriers overcome the systemic barriers for alleviating tumor hypoxia and enhancing PDT. Further research should focus on more effectively oxygen delivery and explore more subcellular organelles-targeted drugs and hypoxia-activated prodrugs in clinic. We hope that these hypoxia-operating and PDT-amplifying systems provide further opportunities for the development of PDT in clinical cancer therapy.
Although we have systematically reviewed the most recent nanomedicine strategies for manipulating tumor hypoxia and enhancing PDT, several challenges remain to be addressed in hypoxia and PDT. Firstly, the traits of tumor hypoxia are heterogeneity of tumor species and steric heterogeneity in a tumor. Hence, it is difficult to precisely manipulate hypoxia to relieve or treat tumors. Secondly, single treatment protocol of PDT does not achieve the desired therapeutic effects due to limited light permeation and the use of photosensitizers with phototoxicity. Finally, although a large number of nanoparticles have been developed to modulate tumor hypoxia for improving PDT in animal models, few of nanoparticles have been approved by FDA for clinical application. The question arises how can we bridge the gap between preclinical animal models and clinical trials? This is the bottleneck not only for nanoparticles but also for directions of future research. Some novel trends in nanotechnology have emerged in recent years to be useful in hypoxia for promoting PDT, such as stimuli-sensitive drug release and combination drug therapy. For future nano-DDS (drug delivery system) design, further heterogeneous features of tumors and multiple drug delivery strategies (e.g., active targeting functionalization, reversal of multi-drug resistance and combination drug therapy) should be taken into consideration. Designing a more precise and multifunctional nano-DDS is supposed to be a research focus in future.
Acknowledgments
This work was financially supported by National Natural Science Foundation of China (Nos. 81573371 and U1608283), Liaoning Revitalization Talents Program (No. XLYC1808017, China), Key projects of Technology Bureau in Shenyang (No. 18400408, China), Key Projects of Liaoning Province Department of Education (No. 2017LZD03, China).
Footnotes
Peer review under responsibility of Institute of Materia Medica, Chinese Academy of Medical Sciences and Chinese Pharmaceutical Association.
Author contributions
Yixin Sun contributed significantly to analysis and manuscript preparation. Dongyang Zhao provided suggestions for the improvement of manuscript. Gang Wang, Yang Wang and Linlin Cao contributed to the correction of the manuscript. Jin Sun, Qikun Jiang and Zhonggui He contributed to the conception of the study and helped perform the analysis with constructive discussions.
Conflicts of interest
The authors have no conflicts of interest to declare.
References
Articles from Acta Pharmaceutica Sinica. B are provided here courtesy of Elsevier
Citations & impact
Impact metrics
Article citations
Ferroptosis-associated genes and compounds in renal cell carcinoma.
Front Immunol, 15:1473203, 27 Sep 2024
Cited by: 0 articles | PMID: 39399506 | PMCID: PMC11466770
Review Free full text in Europe PMC
Stable triangle: nanomedicine-based synergistic application of phototherapy and immunotherapy for tumor treatment.
J Nanobiotechnology, 22(1):635, 18 Oct 2024
Cited by: 0 articles | PMID: 39420366 | PMCID: PMC11488210
Review Free full text in Europe PMC
Fe-HCOF-PEG<sup>2000</sup> as a Hypoxia-Tolerant Photosensitizer to Trigger Ferroptosis and Enhance ROS-Based Cancer Therapy.
Int J Nanomedicine, 19:10165-10183, 07 Oct 2024
Cited by: 0 articles | PMID: 39399828 | PMCID: PMC11468433
Deciphering the Protective Role of HIF-1α Downregulation on HIBD through the MALAT1/miR-140-5p/TGFBR1/NF-κB Signaling Pathway.
Mol Neurobiol, 16 Sep 2024
Cited by: 0 articles | PMID: 39278884
Targeted regulation of autophagy using sorafenib-loaded biomineralization nanoenzyme for enhanced photodynamic therapy of hepatoma.
Mater Today Bio, 29:101270, 24 Sep 2024
Cited by: 0 articles | PMID: 39403315 | PMCID: PMC11472109
Go to all (63) article citations
Similar Articles
To arrive at the top five similar articles we use a word-weighted algorithm to compare words from the Title and Abstract of each citation.
Supramolecular micelles as multifunctional theranostic agents for synergistic photodynamic therapy and hypoxia-activated chemotherapy.
Acta Biomater, 131:483-492, 13 Jul 2021
Cited by: 11 articles | PMID: 34265471
Tumor-targeted hybrid protein oxygen carrier to simultaneously enhance hypoxia-dampened chemotherapy and photodynamic therapy at a single dose.
Theranostics, 8(13):3584-3596, 07 Jun 2018
Cited by: 35 articles | PMID: 30026868 | PMCID: PMC6037038
O2-generating MnO2 nanoparticles for enhanced photodynamic therapy of bladder cancer by ameliorating hypoxia.
Theranostics, 8(4):990-1004, 01 Jan 2018
Cited by: 69 articles | PMID: 29463995 | PMCID: PMC5817106
The application of nanoparticles in cancer immunotherapy: Targeting tumor microenvironment.
Bioact Mater, 6(7):1973-1987, 26 Dec 2020
Cited by: 228 articles | PMID: 33426371 | PMCID: PMC7773537
Review Free full text in Europe PMC
Funding
Funders who supported this work.
National Natural Science Foundation of China (2)
Grant ID: U1608283
Grant ID: 81573371
Program for Liaoning Innovative Talents in University (2)
Grant ID: 81573371
Grant ID: U1608283