Abstract
Free full text

Interplay and cooperation of Helicobacter pylori and gut microbiota in gastric carcinogenesis
Abstract
Chronic Helicobacter pylori infection is a critical risk factor for gastric cancer (GC). However, only 1–3% of people with H. pylori develop GC. In gastric carcinogenesis, non-H. pylori bacteria in the stomach might interact with H. pylori. Bacterial dysbiosis in the stomach can strengthen gastric neoplasia development via generating tumor-promoting metabolites, DNA damaging, suppressing antitumor immunity, and activating oncogenic signaling pathways. Other bacterial species may generate short-chain fatty acids like butyrate that may inhibit carcinogenesis and inflammation in the human stomach. The present article aimed at providing a comprehensive overview of the effects of gut microbiota and H. pylori on the development of GC. Next, the potential mechanisms of intestinal microbiota were discussed in gastric carcinogenesis. We also disserted the complicated interactions between H. pylori, intestinal microbiota, and host in gastric carcinogenesis, thus helping us to design new strategies for preventing, diagnosing, and treating GC.
Background
Helicobacter pylori infection is the critical risk factor for gastric cancer (GC) [1–4]. Inflammation and injury induced by H. pylori can continuously damage the function and architecture of the gastric epithelium [5]. However, it should be mentioned that the successful removal of H. pylori does not necessarily inhibit the GC development [6]. Thus, there may be other factors involved in the carcinogenesis of GC which require further research. Numerous intestinal and gastric microbes have been known as procarcinogens in colorectal cancer and GC [7–11], or probiotics that increase patients’ immunotherapy response with cancer [12]. However, there are few reports about microbiota composition in precancerous lesions.
Normal intestinal flora (IF) has been indicated to accelerate the beginning of gastrointestinal intraepithelial neoplasia (GIN) and increase its development [13]. Non-H. pylori bacteria, pathogenic or commensal IF, may colonize the stomach and show the excessive risk of gastric adenocarcinoma, especially in susceptible patients with H. pylori [14, 15]. INS-GAS (insulin-gastrin) transgenic mice with high levels of circulating gastrin develop spontaneous atrophic gastritis and GIN with an 80% prevalence 6 months after H. pylori infection. Evaluation of this model revealed that commensal intestinal bacteria may promote GC. [16, 17]. Male restricted ASF (a restricted microbiota confined to three species of Altered Schaedler’s Flora) and IF INS-GAS mice presented gastric pathology as the Correa model, even without H. pylori infection [18]. Although ASFs are beneficial for mice [19], it appears that their colonization in the stomach may be involved in the production of various oxidizing agents, oxygen radicals, nitrosamines, and genotoxic compounds and mutagens. Various studies have shown that human gastric colonization with bacteria other than H. pylori such as Actinobacteria, Proteobacteria, Fusobacteria, Firmicutes, and Bacteroides (many are colonized normally in the lower intestine) can affect the gastric adenocarcinoma risk [17, 20–22]. Lactobacillus is a facultative anaerobe representing a gut microbiota component and is in general a probiotic in-transit passenger. The gastric mucosa colonization by Lactobacillus shows an alteration in cancerous patients with gastric microenvironment. A study in Taiwan showed that Lactobacillus is abundantly found in GC patients. This seems to be due to the use of probiotic microbes as a dietary supplement [23].
Gastritis can change the fecal microbiome composition, which might possibly be aggravated by H. pylori infection [24]. The gut microbiome changes could be associated with chronic gastrointestinal diseases, the close interaction of H. pylori infection, gut microbiome, and gastritis [24]. Numerous pieces of evidence show that bacteria and host response interplay may form commensal microbiota composition though the precise mechanism of gastric inflammation leading to fecal microbiota variations is not indicated properly. Gastric microbiota and luminal pH changes may drive the community structure of gut microbiota [25].
Previous studies stated that colonization with non-H. pylori bacteria, gut commensals, changes the ‘resident’ gastric microbiota and the host equilibrium [11]. This article mainly reviewed the influence of intestinal microbiota on GC. Next, it discussed the potential mechanisms of intestinal microbiota in carcinogenesis. Moreover, the interactions between H. pylori, intestinal microbiota, and host in cancer induction were disserted. In the last part of this review, the effects of H. pylori and gut microbiota on metabolic pathways were discussed.
Main text
Gut microbiota in GC
Lactobacillus, Lachnospiraceae, Escherichia-Shigella, Nitrospirae, and Burkholderia are enhanced in GC patients compared with controls [8], confirming previous results with respect to the fact that Lachnospiraceae and Lactobacillus are abundantly found in GC [15, 26–28]. The findings pose a hypothesis; gastric colonization through non-H. pylori bacteria affects the GC risk, and many of them also colonize the intestine. The study by Ferreira et al. approved a notable decline in the abundance of Helicobacter and a significant increase in the genera Achromobacter, Clostridium, Citrobacter, Rhodococcus, and Lactobacillus in Portuguese patients with GC in comparison with chronic gastritis, the ORs were 20.5 (95% CI 7.4–59), 5.7 (95
% CI 2.2–15), 9.9 (95
% CI 4.3–23), 4.2 (95
% CI 1.7–11), and 6.3 (95
% CI 2.9–14), respectively [11]. These members of microbiota are present as commensals in the intestinal mucosa but might be opportunistic pathogens [29, 30]. Evaluations on gastric microbiota show that Lactobacillus is highly abundant in progressive histological phases of gastric carcinogenesis and in GC patients [8, 10, 11, 26]. A study in Sweden found that Lactobacillus was one of the predominant genera in GC patients [15]. Increase of Lactobacillus sp from non-atrophic gastritis, to intestinal metaplasia and to GC was characterized in Mexican patients’ stomach microbiota using the microarray G3 PhyloChip [26]. In another study from Taiwan, Lactobacillus was a highly abundant species in GC patients [23]. Some commensal bacteria were overrepresented in GC. A large amount of Klebsiella pneumoniae and Escherichia–Shigella (belonging to Enterobacteriaceae taxa) was detected in GC patients’ gastric mucosa [7, 8]. A study in China showed that the dominant phyla in the feces of patients with gastric lesions were Firmicutes, Bacteroidetes, and Proteobacteria that accounted for the 99.05
% of all fecal bacteria, and Bacteroides, Escherichia-Shigella, Prevotella_9, and Ruminococcus_2 were the predominant genera [31]. Another study showed that there existed 12 bacterial genera enriched in GC, involving Prevotella_9, Klebsiella, Lactobacillus, Escherichia–Shigella, Streptococcus, Veillonella, Alistipes, Bifidobacterium, Christensenellaceae_R-7_group, Ruminococcaceae_ UCG −
002, Prevotella_2, and Parabacteroides. Enterobacteriaceae and Lachnospiraceae had to be considered at the family level [32]. Veillonella, Lactobacillus, and Streptococcus of GC were increased, in terms of relative abundance, by 32.38-fold, 58.92-fold, and 15.93-fold, and Tyzzerella_3 and Lachnospira were declined by 8.85-fold and 3.37-fold, respectively. These reports show that the genera Lactobacillus, Streptococcus, Veillonella, Tyzzerella_3, and Lachnospira were employed to predict GC [32]. A study in China has shown that some Actinobacteria and Firmicutes species were considerably decreased in patients’ feces with esophageal cancer or GC compared to healthy individuals (P
<
0.05) (Table 1) [33]. In comparison with normal and peritumoral tissues, Prevotella copri and Bacteroides uniformis showed a reduction while Propionibacterium acnes, Streptococcus anginosus, and Prevotella melaninogenica experienced an enhancement in tumor tissues [34]. Based on a recent study on various GC subtypes, Patescibacteria, Bacteroidetes, and Fusobacteria were enhanced in signet-ring cell carcinoma, while Acidobacteria and Proteobacteria showed an incremental trend in adenocarcinoma [35].
Table 1
The relationships of Gut microbiota with GC in the world
Gut microbiota (genera/species) | Related to increase ↑/decrease ↓ of GC | Country | ASR-Both sexes (GLOBOCAN 2018) | Study (Reference) |
---|---|---|---|---|
Lactobacillus, Lachnospiraceae, Escherichia-Shigella, Nitrospirae, andBurkholderia | ↑ | China | 20.7 | Wang et al., 2016 [8] |
Lactobacillus and Lachnospiraceae | ↑ | South Korea | 39.6 | Eun et al., 2014 [28] |
genera Achromobacter, Clostridium, Citrobacter, Rhodococcus, andLactobacillus | ↑ | Portugal | 11. 0 | Ferreira et al., 2018 [11] |
Lactobacillus | ↑ | China | 20.7 | Coker et al., 2018 [10] |
genera Streptococcus, Lactobacillus, Veillonella, and Prevotella | ↑ | Sweden | 3.3 | Dicksved et al., 2009 [15] |
Lactobacillus sp. and Lachnospiraceae | ↑ | Mexico City | 5.6 | Aviles-Jimenez et al., 2018 [26] |
Lactobacillus | ↑ | Taiwan | - | Hsieh et al., 2018 [23] |
Escherichia–Shigella and Klebsiella pneumoniae (belonging to Enterobacteriaceae taxa) | ↑ | South Korea | 39.6 | Jo et al., 2016 [7] |
12 bacterial genera, includingPrevotella_9,Escherichia–Shigella, Klebsiella,Lactobacillus,Streptococcus,Alistipes, Veillonella,Bifidobacterium,Ruminococcaceae_UCG–002,Christensenellaceae_R-7_group,Parabacteroides,andPrevotella_2 | ↑ | China | 20.7 | Qi et al., 2019 [32] |
Species ofFirmicutes andActinobacteria | ↓ | China | 20.7 | Li et al., 2019 [33] |
Potential mechanisms of gut microbiota in carcinogenesis
Gut microbiota mechanisms contributing to carcinogenesis are not clear yet. Dysbiotic microbial community may increase the risk for gastric carcinoma by sustaining the gastric inflammatory process and triggering immune responses [11]. Also, microbial dysbiosis can increase inflammation and dysregulate the immune response, causing DNA mutations, hence, hastening the induction and/or progression of cancers. Such a mechanism may be due to interactions between fecal microbiota, H. pylori infection, and host responses [31]. It is well known that gastritis activity is correlated with H. pylori infection. Subsequent studies also confirmed such an association between gastritis activity and fecal microbiota. Intestinal flora could also increase the inflammatory responses in the mice stomach infected by H. pylori, promoting the development of neoplasia and gastric atrophy [36].
Chronic inflammation
Inflammation aggravates the progression of tumor and hastens metastasis and invasion. Inflammatory cytokines damage DNA in the epithelium directly and induce inflammation-associated cancers [37]. The inflammation associated factors can stimulate oncogenes (e.g., KRAS mutation) and inactivate tumor-suppressor genes (e.g. p53 mutation) [38, 39]. Investigations show that there exists an association between detrimental alterations in the composition of fecal microbiota and the increase in proinflammatory cytokines that induces the disease. K. pneumoniae and Colibactin-producing Escherichia coli can cause chronic inflammation, DNA damage, and mutation [40, 41]. Biagi et al. correlated the Firmicutes and Bacteroidetes reduction and Proteobacteria proliferation with IL-6 and IL-8 increases [42]. IL-11 and IL-6 can sensitize signal transducer and activator of transcription 3 (STAT3) activator, exerting a considerable effect on epithelial cells’ transformation [43]. The symbiont Bacteroides fragilis, which expresses polysaccharide A, is able to suppress proinflammatory IL-17 generation which is developed via Helicobacter hepaticus [44]. Intestinal commensals, segmented filamentous bacteria (SFB) in particular, were correlated with the gut immune maturation regulation and IL-17 generation (Fig. 1A). [45]. Lipoteichoic acid (LTA) also binds to CD14 or Toll-like receptor 2 (TLR2), inducing the excessive secretion of proinflammatory factors [46, 47].
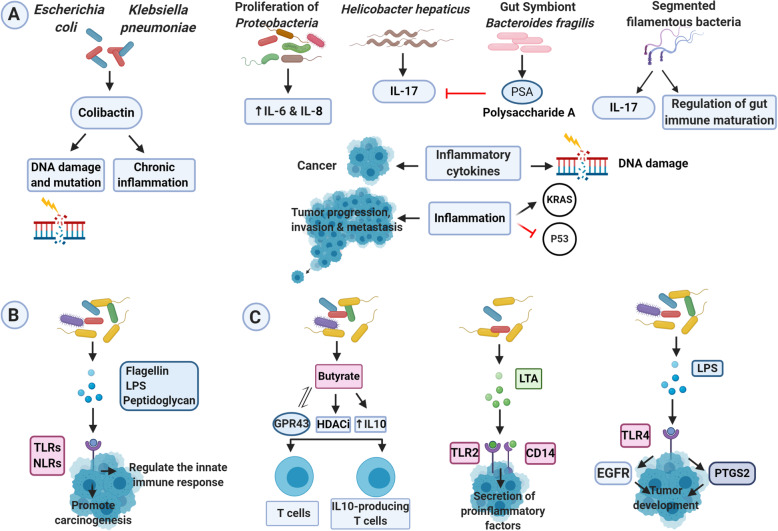
The mechanism of gut microbiota in carcinogenesis. A). Chronic inflammation: Inflammation accelerates the invasion, metastasis, and progression of the tumor. Inflammatory cytokines are directly involved in DNA damage and lead to inflammation-associated cancer. Inflammatory factors can inhibit tumor suppressor genes such as p53, and activate oncogenes such as KRAS. Colibactin produced by Escherichia coli and Klebsiella pneumoniae can cause DNA damage and mutations and trigger chronic inflammation. Proliferation of Proteobacteria is associated with an increase in IL-6 and IL-8. Polysaccharide A (PSA) expressed by symbiont Bacteroides fragilis can suppress IL-17 induced by Helicobacter hepaticus. Specific filamentous bacteria (SFB) are involved in the production of IL-17 and the regulation of gut immune maturation. B). Immune regulation: Dysbiosis of the gut microbiota is associated with cellular immunity and immune function, which affects GC development. The innate immune system can detect the structural components of gut microbiota such as flagellin, lipopolysaccharide (LPS), and peptidoglycans through Toll-like receptors (TLRs) and NOD-like receptors and regulate the innate immune response. C). Microbial metabolites: Butyrate through interaction with G protein-coupled receptor 43 (GPR43), up-regulating IL10 expression, and activating histone deacetylase inhibition (HDACi) can induce the differentiation of IL10-producing T cells and regulatory T cells. The Lipoteichoic acid (LTA) produced by the gut microbiota specifically binds to CD14 (cluster of differentiation 14) or TLR2 and causes the excessive secretion of proinflammatory agents, thus promotes malignant transformation. LPS produced by intestinal microbiota enhances tumor development by regulating the high expression of prostaglandin-endoperoxide synthase 2 (PTGS2) and activating the epidermal growth factor receptor (EGFR) signaling pathway
Microbial metabolites
Intestinal microbial dysbiosis has been associated with cellular immunity and immune function, which affects the GC development [48, 49]. NOD-like receptors (NLRs) and TLRs [50] can bridge this interplay, eventually promoting carcinogenesis in a chronic process. TLRs critically affect the innate immune system, assuming their capability in differentiating host molecules from microbial molecules. NLRs adjust the innate immune response, correspondingly activating inflammasome-mediated dysbiosis and modulating microbial composition (Fig. 1B). The gut microbiota can generate butyrate, which can differentiate regulatory T cells and IL10, generating T cells via the activation of histone deacetylase inhibition (HDACi), interactions with G protein-coupled receptor 43 (GPR43), and IL10 up-regulation [51]. LTA and short chain fatty acids (SCFAs) have opposite roles in carcinogenesis [52]. LTA accelerates malignant transformation. In contrast, SCFAs can mediate immunoregulation by Tregs, hence showing anti-carcinogenic and anti-inflammatory effects [51, 53, 54]. TLRs can develop gastrointestinal tract tumors by activating the STAT3 and NFKB signaling pathways [50]. The TLR4 activation, the receptor for LPS generated by the gut microbiome in the epithelial cells, can induce tumor development by up-regulating prostaglandin-endoperoxide synthase 2 (PTGS2) and activating the epidermal growth factor receptor (EGFR) signaling pathway in mice receiving AOM (Fig. 1C) [55]. In addition, the activation of the STAT3 signaling pathway up-regulates the expression of TLR2 in gastric epithelial cells, promoting tumor development in mice stomach [56].
Bacterial genotoxins
It has been shown that intestinal bacteria are able to potentiate carcinogenesis by the specific toxins inducing DNA damage. As the disturbed microbes overgrow, they increase accumulating endotoxins and exotoxins, like cytolethal distending toxin from Shigella dysenteriae, cytolethal distending toxin and colibactin from E. coli, hydrogen peroxide, extracellular superoxide from Enterococcus faecalis, Enterotoxigenic B. fragilis toxin, a virulence factor activating the NFKB and WNT signaling pathways in epithelial cells [57–59], from B. fragilis, etc. These toxins directly or indirectly cause genomic instability, DNA damage, and the invasion of adenocarcinomas [40, 60–62]. Colibactin in E. coli can induce DNA damage, influence genomic instability [40, 63], and promote carcinogenesis. Colibactin-producing K. pneumoniae can induce chronic inflammation, DNA damage, and mutation [41]. Cytolethal distending toxin produced by the Helicobacter species and E. coli can induce DNA damage in mammalian cells [64–67]. The accumulation of base excision repair (BER) intermediates and unrepaired DNA cause genomic instability and carcinogenesis [68, 69].
Mechanism of H. pylori in carcinogenesis
Genetic diversity can be defined as a leading characteristic of H. pylori strains due to intra-/intergenomic recombination and point mutations [70] which is correlated with the H. pylori pathogenicity, affecting the risk of malignancy [71]. H. pylori can regulate many signaling pathways, stimulate inflammation and immune responses, and trigger epithelial atrophy, achlorhydria, and dysplasia cancer [72]. H. pylori infection induces both innate and adaptive immune responses [73]. Upon recognition of H. pylori pathogen-associated molecular patterns (PAMPs) by the pattern recognition receptors (PRRs) of host cells, the initial stages of the innate immune responses are triggered [74]. As the main component of PRRs, TLRs have the ability to bind the LPS, CpG repeats, unmethylated nucleic acids, flagellin, double-stranded RNA, lipoteichoic acid, and lipoproteins of H. pylori [75]. Upon recognition of PAMPs, by activating activator protein (AP)-1, interferon regulatory factor (IRF), and NF-kB, TLRs manage to promote the expression of inflammatory mediators like TNF-α, IL-1, IL-2, IL-6, IL-8, IL-12, and IFN-γ [76, 77]. H. pylori is able to escape the recognition by the host PRRs of the innate immune response, which may lead to its long-term survival [78]. Concerning adaptive immunity, CD4+ T cells mediate the host immune response toward H. pylori infection [79]. CD4+ T cells have a higher abundance in GC samples than the peritumoral and normal tissues, while CD8+ T cells exhibited the opposite trend [80].
Inflammatory cytokines are highly accumulated in H. pylori-infected individuals’ stomach, including interferon-c, IL-1, TNF-α, IL1b, IL-7, IL-6, IL-8, IL-18, and IL-10. The oncogenic pathways’ activity containing ERK/MAPK, NF-kB, sonic hedgehog, PI3K/Akt, Ras, Wnt/beta-catenin, and STAT3 is up-regulated with H. pylori carrying cytotoxin-associated gene A (CagA). In contrast, with induced P53 mutations, tumor suppressor pathways become inactivate [81, 82]. H. pylori infection can induce methylations on E-cadherin CpG islands [83] and tumor-suppressor genes, consisting of those which encode a forkhead box transcriptional regulator (FOXD3) and the trefoil factor 2 (TFF2), which markedly increase the adenocarcinoma risk in the stomach [84]. The oncoprotein CagA and vacuolating cytotoxin A (VacA) are critical pathogenic factors of H. pylori infection [1, 85–87]. H. pylori expresses the CagA protein, which is a virulence factor that promotes cell proliferation by the activation of the signaling pathways of WNT, PI3K-AKT, and NF-kB [88–90], and reduces epithelial cell apoptosis by inhibiting TP53 [91]. Also, CagA has been approved to activate stemness features and stimulate the epithelial-mesenchymal transition (EMT) in gastric cells [92–96]. By acting on gastric epithelial cells, CagA promotes carcinogenesis through inflammation, proliferation induction, apoptosis inhibition, cell-cell bonding disruption, and loss of cell polarity [97]. The VacA toxin suppresses host immunity via inhibiting the activation of T-cells and inducing regulatory T cells [98–101]. The host immune response can be also modulated by VacA through inhibition of immune cell proliferation and stimulation of mast cells to produce proinflammatory cytokines; further promoting the development of gastritis associated with H. pylori, peptic ulceration, and GC [102]. It induces cell vacuolation [87, 103–105] and autophagy in human-derived gastric epithelial cells [106, 107] through directly affecting mitochondria [108–110], activating vascular endothelial growth factor [111, 112], up-regulating MAP kinase and ERK1/2 expression [113], up-regulating Wnt/beta-catenin pathway necessary for cell differentiation and growth [114], and suppressing GSK3 by the PI3K/Akt signaling pathway [115].
H. pylori virulence factors are involved in the host immune response [79]. The release of inflammatory mediators can activate Th1/Th17 cell responses and stimulate the production of TNF-α, IL-17, and IFN-γ [116]. Therefore, Th1/Th17 cells contribute to mediating the inflammatory response of patients suffering from H. pylori infection [116]. Inflammation may result in loss of acid-secreting parietal cells, hence, increasing the stomach pH, giving rise to declined H. pylori levels and incremental colonization of other bacteria [117]. H. pylori and chronic inflammation can promote the generation of both reactive nitrogen species (RNS) and reactive oxygen species (ROS), leading to DNA damages and induction of apoptosis or autophagy in the gastric epithelial cells [118]. Therefore, H. pylori can induce gastric carcinogenesis through genetic instability. Moreover, ROS induces DNA mutations in H. pylori, promoting its adaption to the host environments [119]. H. pylori-derived LPS can also cause specific impacts on GC cells through TLR4. H. pylori LPS stimulation activates the TLR4 signaling pathway in GC cells through affecting the expression of soluble factors or surface molecules which might help their evasion from CTLs or NK cells by IFN-γ–mediated cellular immune reaction [120]. Low induction of cellular immune response by H. pylori LPS can promote the host susceptibility toward GC development [120]. Based on Kidd et al., H. pylori LPS showed a specific mitogenic influence on gastric enterochromaffin-like cell neoplasia. LPS may exhibit poor virulence in evoking an inflammatory response while showing high potential in augmenting cell growth [121]. The enhanced LPS biosynthesis pathway of GC samples promoted microbiota-induced inflammations [122, 123].
Interplays between H. pylori and gut microbiota
H. pylori infection can affect gut microbiota [122, 124]. It is associated with altered gastric microbiota and dysbiosis implicated in gastric disease pathogenesis [10, 11]. Wang et al.’s study showed that H. pylori infection was related with variations in human intestinal microbial composition and function in Chinese people [125]. Colonization of the stomach with IF (intestinal flora) promotes H. pylori-associated GC. IF effect in developing GC during H. pylori infection has been confirmed in previous studies [36]. Some bacteria, including Bacteroides, Clostridium histolyticum, Prevotella spp., and Lactobacilli have been associated with H. pylori infection in animal models and human trials [126–128]. Prevotella copri is known as a gut microbe that plays a role in the immune system. It was enriched significantly in H. pylori-positive patients. The continuous H. pylori colonization in the stomach brings about the host immune response [128, 129]. A study on a high-risk population showed that the genera Gemella, Rhodococcus, Acidovorax, and Erysipelotrichaceae_UCG-004 in fecal samples were associated with current H. pylori infection [31]. The relative abundances of dominant phyla in the gut of patients with positive H. pylori infection, involving Firmicutes, Bacteroidetes, and Proteobacteria are markedly different from those of individuals with negative H. pylori infection and may be associated with gastric lesions. The average relative abundances, for Proteobacteria and Firmicutes, showed high trends in the past H. pylori infection group (47.11, 20.53%) in comparison with the negative group (23.44 and 9.05
%, respectively) although the p-values (0.068 and 0.246, respectively) revealed no meaningful variations [31]. A study on 1,123 Japanese adults approved more Lactobacillus in patients with H. pylori-infected patients suffering from severe atrophic gastritis [130]. According to Iino et al., infection with H. pylori initially affected the Lactobacillus species’ composition ratio in the gut microbiota prior to the progression of atrophic gastritis and proposed a greater Lactobacillus abundance in patients with H. pylori who suffered from severe atrophic gastritis [130]. Based on a German study, H. pylori increased the lactobacilli growth in fecal microbiome [126]. In the study by Yang et al., fecal microbiome was investigated in children with H. pylori-positive/-negative gastritis and healthy control groups. It was shown that at family and genus levels, the relative abundances of Enterobacteriaceae and Bacteroidaceae were common in gastritis with and without H. pylori infection, while the relative abundances of Lactobacillaceae, Bifidobacteriaceae, and Lachnospiraceae were high in healthy control group. To evaluate the H. pylori effect on gut microbiome among children, the fecal microbiome was analyzed in H. pylori-positive and -negative gastritis groups. The higher abundance of Lactobacillales and Betaproteobacteria and the lower abundance of Alphaproteobacteria were observed in H. pylori-positive group. Higher Streptococcus and Collinsella abundance was found at the genus and family levels in H. pylori-positive group relative to H. pylori-negative group [24]. The H. pylori-infected children also showed increased the number of gut microbiota including Firmicutes, Proteobacteria, Prevotella, and Clostridium compared with those without the infection [131]. In a study by Maldonado-Contreras, microbial community in H. pylori-positive subjects indicated an increase in the counts of Proteobacteria, Acidobacteria, and Spirochaetes [17]. In another study, the gut microbiota of individuals infected with H. pylori was reported to elevate in members of Succinivibrio, Enterococcaceae, Coriobacteriaceae, and Rikenellaceae. The greater abundance of these genera in individuals with H. pylori infection may be associated with the early stages of cancer development and H. pylori pathogenesis [132].
Various studies have shown that H. pylori infection affects the structure of the gut microbiota population. In contrast, some have reported that gut microbiota affects H. pylori colonization. As the diversity of intestinal flora microbiota increases, the level of H. pylori colonization decreases [36]. H. pylori eradication also incremented microbial diversity of the stomach [133]. Study of subjects at different gastric carcinogenesis histologic stages (gastritis, intestinal metaplasia, and GC) showed an inverse association between H. pylori load and microbial diversity of non-cancer gastric biopsies, whereas GC showed a lower diversity in comparison with other samples having the same H. pylori abundance; the difference could be assigned to antibiotic treatment [133]. Lactobacillus casei has been reported to inhibit the growth and colonization of H. pylori in the stomach [134]. Other studies have proved contradictory results about Lactobacillus. A study on H. pylori and Lactobacillus coisolates from humans did not prove a significant effect of lactobacilli on H. pylori strains [135]. A study on the gut microbiota of children with negative H. pylori showed the higher relative abundance of bacteroidia, gammaproteobacteria, clostridia, and betaproteobacteria, and a greater bacterial diversity and richness [136]. A study in China on children’s stool samples showed that at the genus and family levels, the lower abundances of Erysipelotrichaceae, Pseudomonadaceae, and Megasphaera were seen in H. pylori-positive group relative to H. pylori-negative group. It was also shown that the frequency of Faecalibacterium and Roseburia in the H. pylori-positive group was reduced compared to the healthy control group [24]. Many groups have employed sequencing-based and PCR-based methods to show that individuals with negative H. pylori have a very diverse gastric microbiota that is dominated by five predominant phyla: Proteobacteria, Bacteroidetes, Firmicutes, Actinobacteria, and Fusobacteria [16, 17, 137]. Conversely, H. pylori is the utmost abundant bacterium in the stomach and involves the 97 and 72% of all sequence reads among the subjects with positive H. pylori [16, 137]. In a study by Maldonado-Contreras, the microbial community in individuals with positive H. pylori was known by a decline in Bacteroidetes, Actinobacteria, and Firmicutes counts [17]. Bik et al., reported that the individuals with negative H. pylori carry the higher abundant phyla of Bacteroidetes, Firmicutes, and Actinobacteria [16]. Conversely, a study from China showed that the relative abundance of Bacteroidetes was greatly reduced from H. pylori negative to past infection community (66.16
%, 33.01
%, respectively; p
=
0.007). Rhodococcus and Acidovorax had slightly lower average relative abundance at the genus level in patients that are currently infected with H. pylori compared with others that are not currently infected (p
=
0.017 and 0.016, respectively). It was also shown that the average relative abundance of the two genera (the phylum of Bacteroidetes; Barnesiella and Parabacteroides) was decreased among the groups having the different status of H. pylori infection (negative: 1.15 and 2.44
%, past infection: 0.58 and 1.27
%, respectively) [31].
H. pylori and gut microbiota interaction in cancer
The interactive associations between H. pylori and other gastric bacteria have not been completely understood [102]. H. pylori infection has been linked to altered gastrointestinal microbiota and dysbiosis, all of which have been linked to the pathogenesis of gastric diseases [10, 11]. It is not, however, clear whether infection with H. pylori itself approves the growth of unwanted microorganisms or an altered microbiota brings about beneficial situations for the colonization of H. pylori [123]. Probably, there is a multifaceted interaction, where the H. pylori colonization contributes to the growth of some microbes and vice versa. It is likely that dysbiosis alters gastric mucosa which is highly desired for the colonization of H. pylori [124].
Some researchers believe that H. pylori is more of a latent or opportunistic pathogen than a pathogenic bacterium and can be considered a commensal organism. This is important because we know that the majority of the world’s population is infected with H. pylori and colonization occurs with bacteria that carry or do not carry critical virulence factors at an early age. However, it should be noted that severe gastrointestinal diseases or complications occur mainly in adults with age>
40 years and only in <
10
% of infected individuals. This low incidence clearly showed that H. pylori is more of a latent or opportunistic pathogen than a pathogenic bacterium, and that virulence factors play little role in the outcome of the disease [138]. Long-term colonization of H. pylori and its interaction with other gastric microbiota appear to alter gastric mucosal dysbiosis and lead to the development of severe gastrointestinal disease, including GC, by inducing persistent and long-term inflammatory responses [31, 124].
It can be speculated that the alterations of gut microbiota induced by H. pylori may affect the development of GC since the composition of microbiota stimulates immune responses at a systemic and local level; moreover, the development of GC is affected by inflammatory signaling [31]. The interaction between gut microbiota and H. pylori is not known yet and literature reveals inconsistent results [132]. The gastritis activity is perceived for its tight correlation with H. pylori infection, which is further approved by similar changes observed in the fecal microbiota from the subjects with non-active gastritis and past infection. Additionally, the same alteration tendencies were found for major genera or phyla, including reduced Bacteroidetes abundance and increased Proteobacteria or Firmicutes abundances, with gastric lesion severity and H. pylori infection status (particularly the status of past infection). Furthermore, it states that changes in intestinal microbiota may develop precancerous gastric lesions related to H. pylori and carcinogenesis [31]. It has been suggested that lactic acid-producing bacteria may promote gastric inflammatory reactions induced by H. pylori [24]. Lactic acid bacteria promote immune tolerance, providing the platform for colonization of other carcinogenic bacteria [139].
By modulating the acidity of the stomach, H. pylori could change the gastric microbiome profiles, promoting H. pylori-associated disorders. Alterations in the gastric environment that decline acid secretion can encourage the growth of NOC-producing bacteria, thus elevating the chance of gastric carcinogenesis [102]. Th1/Th17 cells contribute to the inflammatory response of H. pylori-infected patients [116, 140] Inflammation increases gastric pH, which decreases H. pylori levels and increases non-H. pylori bacteria in the stomach [117]. There is a significant difference in the microbial profiles and composition of early and advanced GC, reflecting the changes related to GC progression. The gastric microbiome alterations in early GC stages could be assigned to host genetic changes, H. pylori infection, bacterial virulence, and adaptation to the environment. Constrained principal coordinate analyses indicated the influence of H. pylori and cagA and vacA genotypes on the gastric microbiome structure. The detected microbial fingerprint can be regarded as a biomarker for clinical evaluation of GC risk among high-risk cases [141].
Effect of H. pylori and gut microbiota on metabolic pathways and carcinogenesis
Gut microbiota changes correlate with different inflammatory and metabolic illnesses. Little is known about the effect of H. pylori on downstream gut microbiota though many studies have examined the correlation between gastric microbiota and H. pylori [132]. Microbiome alterations are often followed by variations in microbial functions. The relative abundance of 19 gut microbial pathways differs significantly between H. pylori-negative and H. pylori-positive subjects [142]. Persistent H. pylori infection can induce detrimental inflammatory processes besides the impact on host microbes [143]. Epidemiological studies show that H. pylori infection is related with the lower levels of vitamin B12 (VB12) in the blood [144, 145]. The H. pylori infection-related intestinal microbiota dysbiosis can influence the VB12 production. VB12 is a cobalt corrinoid. As humans cannot produce VB12, it is generated exclusively by the microorganisms, especially anaerobes [146]. In the study of Wang et al., it was observed that the levels of plasma VB12 and gut microbial VB12 biosynthesis were meaningfully lower in the subjects with positive H. pylori in comparison to the subjects with negative H. pylori (p<
0.05, Wilcoxon test). Lower VB12 biosynthesis module was linked to the lower levels of VB12 concentrations in subjects with H. pylori infection, manifesting that H. pylori infection-related gut microbiota dysbiosis enhances the VB12 deficiency risk. This shows that some changes in gut microbial species and functions correlate with H. pylori infection, suggesting that the gut microbial shift in the patients with H. pylori infection may raise VB12 deficiency indirectly [125]. In addition, previous studies have inferred that gastric sinusitis, induced by H. pylori infection, may develop type B chronic gastritis, followed by reduced the secretion of gastric acid, leading to VB12 malabsorption [145, 147]. Thus, both the absorption capacity and production of VB12 can be attenuated by H. pylori infection, augmenting the VB12 deficiency risk. Low serum vitamin B12 levels are significantly correlated to the elevated risk of non-cardia gastric adenocarcinoma (NCGA) [148]. H. pylori infection has been also related to food-bound vitamin B12 malabsorption [149, 150] possibly because of the atrophic gastritis induction which is accompanied by achlorhydria (increased gastric pH). Furthermore, vitamin B12 absorption needs acid-producing gastric mucosa, allowing for vitamin B12 cleavage from its binding proteins [151]. As a result, any stimulus inducing chronic atrophic gastritis can enhance the risk of NCGA, disturb vitamin B12 absorption, and thus, declines its serum concentrations [148]. Since vitamin B12 uptake necessitates intact gastric mucosa for acid production, the findings proposed vitamin B12 as a potential serologic marker of NCGA-preceding atrophic gastritis [148].
A recent study on children has shown that altered intestinal microbiota, gastritis, and H. pylori interact with each other. Possibly, H. pylori changes the gut micro-environmental cues, like pH alterations that cause this compositional shift between native communities to compensate. This compensation will be translated into distinctive functional genes contributing to crucial metabolic pathways [132]. It has also been shown that gut microbiome influenced by gastritis and H. pylori infection changes the body’s basal metabolic function [24]. Seventeen KEGG pathways revealed notable variations in H. pylori-infected group and healthy control group. The results of this study manifested the meaningful increase of activity in children’s metabolic pathways, who are H. pylori-positive [24]. However, peptidoglycan biosynthesis was depleted in the H. pylori-positive group as metabolism-related pathways (fatty acid metabolism, LPS biosynthesis, beta-lactam resistance, xenobiotics metabolism by cytochrome P450, glycosphingolipid biosynthesis–ganglio series, glycosphingolipid biosynthesis–globo series, N-glycan biosynthesis, and glycosaminoglycan degradation) were enriched in the H. pylori-positive group [24]. H. pylori is dependent on unsaturated fatty acid (UFA) biosynthesis to maintain its membrane function and structure [152]. The microbial UFA level is meaningfully increased in the blood of the patients developing H. pylori-induced peptic ulceration [153]. Based on these results, it can be said that H. pylori is associated with the high metabolism of lipid. According to the microbiome’s functional analysis, lipid metabolism pathway was increased in the gastritis group, showing that gut microbiome similarly affects H. pylori-induced gastritis (Fig. 2) [24]. UFA biosynthesis plays a decisive role in the integrity of membrane structure and function. H. pylori can grow at anaerobic conditions [152], allowing for H. pylori persistence and induction of carcinogenic consequences within the gastric niche.
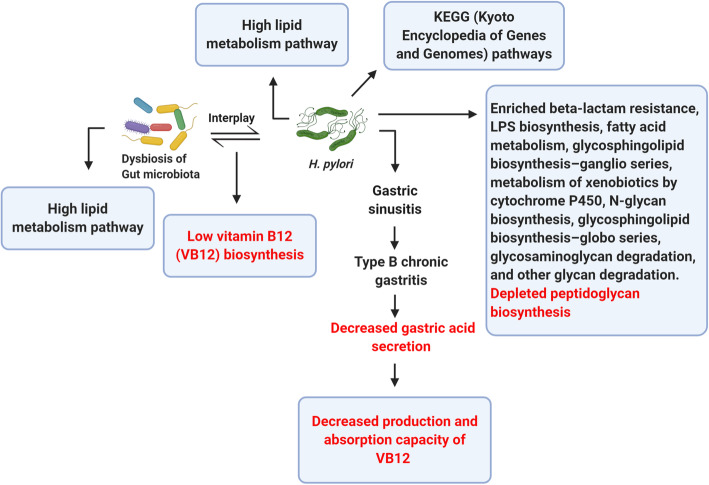
The H. pylori infection-related dysbiosis of gut microbiota correlates with the low levels of vitamin B12 (VB12) production. Gastric sinusitis caused by H. pylori infection is also associated with the decreased production and absorption of vitamin VB12. KEGG (Kyoto Encyclopedia of Genes and Genomes) pathways and pathways related to metabolisms (lipopolysaccharide (LPS) biosynthesis, beta-lactam resistance, glycosphingolipid biosynthesis–globo series, glycosphingolipid biosynthesis–ganglio series, fatty acid metabolism, xenobiotic metabolism by cytochrome P450, N-glycan biosynthesis, glycosaminoglycan degradation, and other glycan degradation) are increased in H. pylori infection, and peptidoglycan biosynthesis pathways are decreased in infection with this bacterium. Both H. pylori infection and gut microbiota dysbiosis are associated with the high metabolism of lipid
Effect of gut microbiota on cell metabolites and carcinogenesis
Intestinal bacteria generate different metabolites affecting the progression and development of gastrointestinal tract tumors [154]. Polyamines, generated by gut bacteria and host cells, largely influence different pathologic and biologic processes, such as translation, stress resistance, gene regulation, and cell differentiation and proliferation [155]. Polyamines suppress antitumor immunity and promote cancer cells’ proliferation, invasion, and metastasis [156]. SCFAs are dietary fiber fermentation products generated by intestinal microbiota, such as propionate, acetate, and butyrate. They can maintain microbiota homeostasis and the intestinal barrier integrity and suppress inflammation and cancer [157]. SCFAs such as butyrate, generated by the gut microbiota, may inhibit carcinogenesis and inflammation through blocking the activation of the NFKB signaling pathway, and differentiating IL10-producing T cells and regulatory T cells [51, 158, 159]. Moreover, butyrate can act as a histone deacetylase inhibitor to suppress the proliferation of the cells, induce apoptosis, and suppress the development of the tumor [160–162]. In contradiction, low butyrate concentrations may potentiate the tumor growth that, in a mouse model, suppresses DNA mismatch repair deficiencies (Fig. 3) [163].
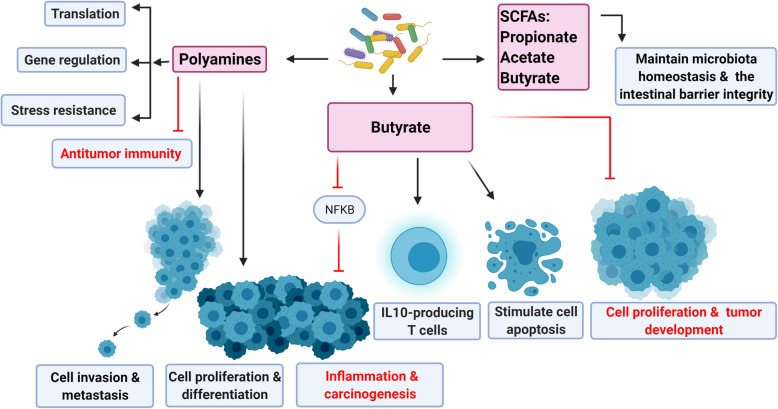
Gut microbiota produce various metabolites that are involved in the development and progression of cancer. Polyamines play important roles in translation, gene regulation, stress resistance, antitumor immunity suppression, cell proliferation, invasion, and metastasis. Short chain fatty acids (SCFAs) generated by intestinal microbiota, such as butyrate, acetate, and propionate can maintain microbiota homeostasis and the intestinal barrier integrity. Butyrate produced by gut microbiota can suppress inflammation and carcinogenesis by blocking the signaling pathway of NFKB activation. Butyrate can induce the differentiation of regulatory T cells and IL10-producing T cells. Butyrate also acts as a histone deacetylase and leads to the inhibition of cell proliferation, stimulation of apoptosis, and suppression of tumor development
Functional analysis of the gastric microbiome indicated a significant reduction in the production of urease and bacterial flagella synthesis at early GC stages, whereas fructose glycolysis and glycosides hydrolysis showed an enhancement. The frequency of glucose-6-phosphate dehydrogenase exhibited a decrease, reflecting a decrement in carbohydrate degradation. The relative frequency of 6-phosphofructokinase (COG205) showed a drastic reduction in advanced GC cases [141]. Numerous bacteria (e.g. Nitrospirae, Lactobacillus, Neisseria, Staphylococcus, Haemophilus, Clostridium, and Veillonella) promote gastric carcinogenesis through stimulation of the N-nitroso compounds (NOCs) production [8, 164]. Higher levels of lactic acid bacteria were found in GC patients [165]. These bacteria may enhance the GC risk by several mechanisms such as elevated generation of ROS, NOCs, and lactate in addition to inducing EMT and immune tolerance [102]. In vitro and in vivo investigations suggested the stimulating role of lactic acid bacteria in ROS generation which may lead to DNA damage. Enhanced the formation of NOCs can promote mutagenesis, angiogenesis, and the expression of protooncogenes, resulting in apoptosis inhibition [139, 166]. Lactic acid bacteria-produced lactate is a robust energy source for cancer cells [167] with a regulatory role in various carcinogenesis issues such as tumor angiogenesis and metastasis [168]. These bacteria are capable of promoting EMT with contributive roles in tumor invasion and metastasis [169] through induction of multipotency state [139].
Microbiome-based GC therapy
Conventional GC therapies such as surgery, chemotherapy, and radiotherapy have not shown high efficacy [102]. H. pylori eradication could be an effective approach to reduce the GC risk. Antibiotic treatment of H. pylori has been shown to alter the gastric microbiome composition [133, 164]. Regarding the increasing rate of antibiotic resistance of H. pylori, novel H. pylori eradication strategies are urgently required. Some probiotics have shown promises in the prevention of antibiotic-induced adverse impacts, an increase in H. pylori eradication rate, and the reduction of fluctuations in the gut microbiome profiles [170]. Lactobacillus supplementation can effectively eradicate H. pylori [171, 172] and reduce the chance of GC development [102]. Some Lactobacillus strains mitigated H. pylori by inhibiting its adhesion to epithelial cells, production of organic acids or bacteriocins, and suppression of mucosal inflammation [173, 174]. Lactobacillus acidophilus and Lactobacillus bulgaricus can decrement the H. pylori adhesion to gastric mucosal cells [175], L. bulgaricus showed inhibitory impacts on IL-8 production of mucosal cells by modulation of the TLR4/IkBa/NF-kB pathways [175]. A probiotic mixture containing Lactobacillus and Bifidobacterium showed helpful influences against H. pylori, at low side effects [176]. A combination of Bacillus cereus, Enterococcus faecalis, L. acidophilus, and Bifidobacterium infantis increased the host immunity and declined inflammation among GC cases undergoing gastrectomy [177].
Despite the traditional carcinogenic role of bacteria, new studies have revealed their anticancer features. The anticancer properties of bacteria can be assigned to various mechanisms such as colonization in tumors, releasing active agents, a carrier for anticancer drugs delivery, suppression of vital nutrients for tumor metabolism and proliferation, reinforcement of host immunity, and biofilm formation [102, 178, 179]. The KLA peptide is a pro-apoptosis peptide KLAKLAKKLAKLAK with anticancer activities through apoptosis induction by disrupting mitochondrial membrane; it however showed poor membrane permeability [180]. HPRP-A1 (H. pylori ribosomal protein) and its enantiomer HPRP-A2 (15-mer cationic peptides) can be derived from the N-terminus of H. pylori ribosomal protein L1 [181]. HPRP-A1 and HPRP-A2 have exhibited powerful antimicrobial and anticancer features. HPRP-A1—a membrane-active peptide—is capable of disrupting the tumor cell membrane. It is largely employed in drug delivery to cancer cells [182]. HPRP-A1 can facilitate the entry of KLA peptides to cancer cells, hence, promoting tumor cell death [183]. Apoptosis induction of HPRP-A2 in the GC cells is achieved via elevation of ROS production; activation of caspases (3, 8, and 9); reduction of mitochondrial membrane potential, and cell cycle arrest within the G1 phase [184].
Conclusions
Mechanistic studies evaluating how gut microbes regulate health and promote gastrointestinal cancers are still at the early stage. Nevertheless, researchers have determined that gut microbiota are in close relation with humans and markedly influence GC and human health. Researchers have taken some steps to regulate gut microbes. The objectives are multifaceted, including the regulation of human metabolism, immune, and inflammatory reaction, as well as inhibiting carcinogenesis and cancer progression. Significant advances have been made in understanding the interaction between H. pylori and intestinal microbiota in the development of gastritis and cancer. However, there have been controversies in the findings of different studies which seem to be due to environmental differences (e.g., diet, etc.) or genetic differences of the host. Detailed studies in well-defined human populations are still required to compare the composition differences of the gut microbiome in different anatomical regions of the stomach of individuals developing H. pylori infection with and without neoplastic lesions. Future investigations are recommended to assess the effect of the gut microbiome composition in various anatomical stomach regions on the risk of cancer. These could be carried out by the site-specific topographical mapping of the microbiota in the absence or presence of H. pylori and by assessing variations with respect to the states of the disease along the gastric carcinogenesis cascade [185]. Deeper and better understanding of the relationship between H. pylori-related precancerous gastric lesions and gut microbiota, and the complicated interaction between them can have a significant impact on the design of new strategies for the prevention, diagnosis and treatment of GC.
Authors’ contributions
S.L-N. provided direction in the preparation of the manuscript. S.Z.B. performed primary literature search. S.Z.B. wrote the first draft of manuscript. S.L-N. discussed and revised the manuscript. S.Z.B. managed the references. S.L-N. approved the version to be published. All authors have read and approved the manuscript.
Funding
The National Institute for Medical Research Development (NIMAD) (grant number 958117), Tehran, Iran. The supporter had no role in study design, data collection and analysis, decision to publish, or preparation of the manuscript.
Availability of data and materials
Data sharing is not applicable to this article as no datasets were generated or analysed during the current study.
Declarations
Not applicable.
Not applicable.
No potential conflicts of interest.
Footnotes
Publisher’s Note
Springer Nature remains neutral with regard to jurisdictional claims in published maps and institutional affiliations.
References
Articles from BMC Microbiology are provided here courtesy of BMC
Full text links
Read article at publisher's site: https://doi.org/10.1186/s12866-021-02315-x
Read article for free, from open access legal sources, via Unpaywall:
https://bmcmicrobiol.biomedcentral.com/track/pdf/10.1186/s12866-021-02315-x
Citations & impact
Impact metrics
Citations of article over time
Alternative metrics

Discover the attention surrounding your research
https://www.altmetric.com/details/113979299
Article citations
Altered Microbiome Promotes Pro-Inflammatory Pathways in Oesophago-Gastric Tumourigenesis.
Cancers (Basel), 16(19):3426, 09 Oct 2024
Cited by: 0 articles | PMID: 39410045 | PMCID: PMC11476036
Review Free full text in Europe PMC
Impact of Pulmonary microbiota on lung cancer treatment-related pneumonia.
J Cancer, 15(14):4503-4512, 17 Jun 2024
Cited by: 1 article | PMID: 39006071
Gastric Cancer, Immunotherapy, and Nutrition: The Role of Microbiota.
Pathogens, 13(5):357, 26 Apr 2024
Cited by: 0 articles | PMID: 38787209 | PMCID: PMC11124250
Review Free full text in Europe PMC
The Role of Probiotics in the Eradication of Helicobacter pylori and Overall Impact on Management of Peptic Ulcer: A Study Involving Patients Undergoing Triple Therapy in Bangladesh.
Cureus, 16(3):e56283, 16 Mar 2024
Cited by: 0 articles | PMID: 38495972 | PMCID: PMC10944298
Helicobacter pylori infection and small intestinal bacterial overgrowth: a systematic review and meta-analysis.
BMC Microbiol, 23(1):386, 06 Dec 2023
Cited by: 0 articles | PMID: 38053022 | PMCID: PMC10698970
Review Free full text in Europe PMC
Go to all (19) article citations
Similar Articles
To arrive at the top five similar articles we use a word-weighted algorithm to compare words from the Title and Abstract of each citation.
Effect of Helicobacter pylori on gastrointestinal microbiota: a population-based study in Linqu, a high-risk area of gastric cancer.
Gut, 69(9):1598-1607, 19 Dec 2019
Cited by: 126 articles | PMID: 31857433 | PMCID: PMC7456744
Suicide journey of H. pylori through gastric carcinogenesis: the role of non-H. pylori microbiome and potential consequences for clinical practice.
Eur J Clin Microbiol Infect Dis, 38(9):1591-1597, 17 Apr 2019
Cited by: 11 articles | PMID: 31114971
Review
In Vivo Analysis of the Viable Microbiota and Helicobacter pylori Transcriptome in Gastric Infection and Early Stages of Carcinogenesis.
Infect Immun, 85(10):e00031-17, 20 Sep 2017
Cited by: 31 articles | PMID: 28694295 | PMCID: PMC5607399
Funding
Funders who supported this work.
National Institute for Medical Research Development (1)
Grant ID: 958117