Abstract
Free full text

Mitochondrial Dynamics in SARS-COV2 Spike Protein Treated Human Microglia: Implications for Neuro-COVID
Abstract
Abstract
Emerging clinical data from the current COVID-19 pandemic suggests that~
40% of COVID-19 patients develop neurological symptoms attributed to viral encephalitis while in COVID long haulers chronic neuro-inflammation and neuronal damage result in a syndrome described as Neuro-COVID. We hypothesize that SAR-COV2 induces mitochondrial dysfunction and activation of the mitochondrial-dependent intrinsic apoptotic pathway, resulting in microglial and neuronal apoptosis. The goal of our study was to determine the effect of SARS-COV2 on mitochondrial biogenesis and to monitor cell apoptosis in human microglia non-invasively in real time using Raman spectroscopy, providing a unique spatio-temporal information on mitochondrial function in live cells. We treated human microglia with SARS-COV2 spike protein and examined the levels of cytokines and reactive oxygen species (ROS) production, determined the effect of SARS-COV2 on mitochondrial biogenesis and examined the changes in molecular composition of phospholipids. Our results show that SARS- COV2 spike protein increases the levels of pro-inflammatory cytokines and ROS production, increases apoptosis and increases the oxygen consumption rate (OCR) in microglial cells. Increases in OCR are indicative of increased ROS production and oxidative stress suggesting that SARS-COV2 induced cell death. Raman spectroscopy yielded significant differences in phospholipids such as Phosphatidylinositol (PI), phosphatidylserine (PS), phosphatidylethanolamine (PE) and phosphatidylcholine (PC), which account for
~
80% of mitochondrial membrane lipids between SARS-COV2 treated and untreated microglial cells. These data provide important mechanistic insights into SARS-COV2 induced mitochondrial dysfunction which underlies neuropathology associated with Neuro-COVID.
Graphical Abstract
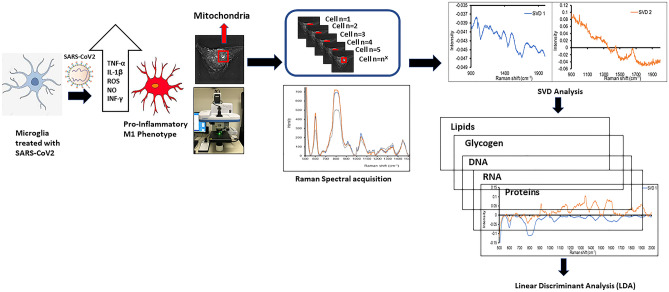
Introduction
The clinical presentation of COVID-19 is associated with significant neurological symptoms and neuropathologies such as acute ischemic stroke, meningitis/encephalitis, anosmia, neuromuscular diseases, epileptic seizures, focal neurological deficits as well as neuropsychological abnormalities such as depression, delirium, and psychosis (Al-Dalahmah et al. 2020; Beyrouti et al. 2020; Dixon et al. 2020; Oxley et al. 2020; Paterson et al. 2020; Pero et al. 2020; Poyiadji et al. 2020; Steardo et al. 2020). Emerging data suggests that 85% of COVID-19 long-haulers have multiple neurological symptoms (Clark et al. 2021). Pathological mechanisms that underlie these neurological manifestations include induction of hypoxia, sepsis, severe systemic inflammation and the cytokine storm. Chronic neuro-inflammation and neuronal damage results in a syndrome described as “Neuro-COVID”. SARS‐COV2 has been isolated from brain tissue with edema and neuronal degeneration. Electron microscopic analysis confirms the localization of SARS-COV2 in neurons, astroglia and microglia.
SARS-COV2 uses the S1 spike protein to attach the virion to the cell membrane by interacting with the hosts ACE2 receptor (Wrapp et al. 2020). The neurovirulence of SARS-COV2 is attributed to the expression of the ACE2 receptor by brain microvascular endothelial cells that line the blood brain barrier (BBB), and facilitate the entry of the virus in the brain (Baig et al. 2020). Microglia, neurons and astrocytes also express ACE2 receptors making them a potential target of SARS-COV2.
Microglia are the resident immune cells of the Central Nervous system (CNS) and represent 5–20% of the adult brain. Microglia have the capacity to migrate, proliferate and phagocytize. Under physiological conditions, microglia exist in their “resting” state, however on exposure to a pathogen, microglia transition into an activated state and quickly mobilize to the site of injury to initiate an innate immune response and therefore they are widely used to study neuro-inflammation (Zhao et al. 2018; Zhou et al. 2020). Microglia are involved in neuroprotective and neurotoxic responses in the CNS in response to SARS-COV 2 infection (Hanisch and Kettenmann 2007; Farfara et al. 2008; Harry and Kraft 2008; Bachiller et al. 2018; Fatoba et al. 2020). The severe cytokine storm in COVID -19 patients is associated with increased serum levels of proinflammatory cytokines and increased BBB permeability due to cytokine-induced damage (Jahani et al. 2020; Daniels et al. 2014). Cytokines activate microglia and astrocytes and activate microglia to secrete inflammatory mediators, inducing altered neuroplasticity, and other neuropathologies resulting in neuro-cognitive impairment (Fu et al. 2020; Liddelow et al. 2017). SARS-COV2 induces a mitochondrial-dependent intrinsic apoptotic pathway, leading to the activation of caspase-9 followed by initiation of executioner caspases-3/7 (Imre 2020; Bertheloot et al. 2021; Lee et al. 2020); SARS-COV2 spike protein manipulates mitochondrial mechanisms to evade the host immune response and may alter mitochondrial functions leading to enhanced ROS production, perturbed signaling, and blunted antiviral defenses of the host. We speculate that SAR-COV-2 induces mitochondrial dysfunction and activation of the mitochondrial-dependent intrinsic apoptotic pathway, resulting in microglial and neuronal apoptosis. We examined the effect of a recombinant SARS-COV2 spike protein and heat inactivated SARS-COV2 on cytokine production, ROS /NOS production, mitochondrial bioenergetics, and the possible mechanisms that may underlie SAR-COV2 induced mitochondrial dysfunction in human microglial cells. Our results showed that microglia treated with SARS-COV2 release an increased amount of pro-inflammatory cytokines suggesting that SARS-COV2 induces an increased inflammatory response and cytokine dysregulation. SARS-COV2 also induces an increased level of oxidative stress, HIF-1α production and inflammasome activation, contributing to both mitochondrial dysfunction and increased ROS production. Additionally, we used Raman spectroscopy for prognostic evaluation of mitochondrial function in SARS-COV2 treated microglial, which yielded real time information on SARS-COV2 induced changes in protein & lipid composition, stress response, nuclear division, respiratory activity and cell apoptosis. Our data provide important mechanistic insights into SARS-COV2 induced mitochondrial dysfunction which underlies neuropathology associated with Neuro-COVID. Understanding the pathophysiology behind the neurological symptoms associated with COVID-19 patients will allow development of targeted therapeutics for patients with Neuro-COVID.
Material and Methods
Cell Culture
Human Microglia (HMC3) were obtained from ATCC (Cat # ATCC® CRL-3304™) and grown in Eagle's Minimum Essential Medium (EMEM) (Cat #ATCC® 30–2003™) supplemented with 5% fetal bovine serum (FBS), 100 U/ml penicillin, and 100 μg/ml streptomycin. Cultures were maintained at 370C in a humidified 5% CO2 in air incubator. We treated human microglia (HMC3 -ATCC) with (0.5 µg/ml) of recombinant spike protein from SARS-COV2, Wuhan-Hu-1 (Cat # NR-52308; Lot: 70,034,410 BEI resources Inc) which is described as a recombinant form of the spike (S) glycoprotein from SARS-COV2, Wuhan-Hu-1 (GenPept: QJE37812) which was produced by transfection of purified plasmid in SF9 insect cells using a baculovirus expression system and purified by nickel affinity chromatography or 5 µl/ml of heat inactivated SARS-COV2, Isolate USA-WA1/2020, (Cat # NR-52286, Lot: 70,033,548; Pre-Inactivation Titer by TCID50 Assay in Vero E6 Cells=
1.6
×
105 TCID50 per mL, BEI resources Inc) for 24–48 h followed by cell viability assays, gene expression analyses, quantitation of mitochondrial respiration, ROS quantitation. The Raman spectroscopic analysis for these cells was done at an 3 h time point, post treatment which enables examining early changes in SARS-COV2 induced cell apoptosis.
Cell Viability Assay
Microglia (100,000 cells) were treated with 0.5 µg/ml of SARS-COV2 spike protein or 0.5 µl/ml of heat inactivated SARS-COV2 isolate for 48 h and cell viability was measured using the CCK-8 assay kit (Dojindo Molecular Technologies, Inc., Rockville, MD). The assay uses a water-soluble tetrazolium salt, WST-8, which is reduced by dehydrogenase activity in cells to produce a yellow-color formazan dye soluble in the tissue culture media and is optical density(OD) quantitated using a spectrophotometer at 450 nm. The amount of the formazan dye generated by the dehydrogenase activity in cells is directly proportional to the number of living cells.
RNA Extraction
Microglia (100,000 cells) were treated with 0.5 µg/ml of SARS-COV2 spike protein or 5 µl/ml of heat inactivated SARS-COV2 isolate for 48 h followed by extraction of cytoplasmic RNA by the acid guanidinium-thiocyanate-phenol–chloroform method using Trizol reagent (Invitrogen-Life Technologies, Carlsbad, CA). T he amount of RNA was quantified using a Nano-Drop ND-1000 spectrophotometer (Nano-Drop™, Wilmington, DE), and isolated RNA was stored at –80 °C until used.
Real Time qPCR
500 ng of total RNA extracted as outline above, was used for the First-Strand cDNA Synthesis Kit (GE Healthcare, Piscataway, NJ), according to the manufacturer’s instructions. One microliter of the resultant cDNA from the RT reaction was employed as the template in PCR reactions using well validated PCR primers obtained from the RealTimePrimers.com, that include the pro-inflammatory cytokines IL-8, IL-1β and TNF-α. Primers were provided as a 20 µl solution containing both forward and reverse primers at a final concentration of 10 µM in 10 mM Tris–HCl (pH 7.5) and 0.1 mM EDTA, which were diluted with RNAase/DNAse free water as needed prior to use. We used the SYBR® Green master mix that contained dNTPs, MgCl2, and DNA polymerase (Bio- Rad, Hercules, CA).The final primer concentration used in the PCR was 0.1 µM. PCR conditions were as follows: 95 °C for 3 min, followed by 40 cycles of 95 °C for 40 s, 60 °C for 30 s, and 72 °C for 1 min; the final extension was at 72 °C for 5 min. Gene expression was calculated using the comparative CT method. The threshold cycle (Ct) of each sample was determined, the relative level of a transcript (2ΔCt) was calculated by obtaining ΔCt (test Ct−
GAPDH Ct), and transcript accumulation index (TAI) was calculated as TAI
=
2−ΔΔCT (Livak and Schmittgen 2001).
Apoptotic Cell Quantitation using the Caspase-3/7 Detection Reagent
CellEvent™ Caspase-3/7 Green Detection Reagent (Cat #: C10423 Thermo Fisher Scientific) enables the examination of Caspase-3/7 activation in live cells, and is a four-amino acid peptide (DEVD) conjugated to a nucleic acid-binding dye with absorption/emission maxima of~
502/530 nm. The DEVD peptide sequence is a cleavage site for caspase-3/7, and the conjugated dye is non-fluorescent until cleaved from the peptide and bound to DNA. Upon Caspase-3/7 activation in apoptotic cells, the DEVD peptide is cleaved, enabling the dye to bind to DNA and produce a bright, fluorogenic response. The reagent is added to microglial cells grown to 70% confluence in a petri dish with a glass bottom, treated with 0.5 µg/ml of SARS-COV2 spike protein for 24 h, then incubated for 30 min, and visualized using the EVOS® FL Cell Imaging System (Life Technologies, Grand Island, NY). Apoptotic cells with activated caspase-3/7 exhibit bright green nuclei, while cells without activated caspase-3/7 exhibit minimal fluorescence.
Measurement of ROS- a General Oxidative stress Indicator
ROS was quantitated using CM-H2DCFDA reagent from Invitrogen™ (Cat # C6827). CM-H2DCFDA is a chloromethyl derivative of H2DCFDA, useful as an indicator for ROS generation in cells and a general oxidative stress indicator. CM-H2DCFDA passively diffuses into cells, where its acetate groups are cleaved by intracellular esterases and its thiol-reactive chloromethyl group reacts with intracellular glutathione and other thiols and subsequent oxidation yields a green fluorescent product that can be quantitated using fluorescent microscopy imaging (Ex/Em:~
492–495/517–527 nm). Microglial cells were plated on glass-bottom petri dishes and cells grow to 80% confluency. Cell were then treated with 0.5 µg/ml of SARS-COV2 spike protein for 24 h following which cells were washed with 1X PBS, following which 5 μM of CM-H2DCFDA (freshly prepared in HBSS) was added and cells incubated for 30 min in dark CO2 incubator. After 30 min, cells were washed with PBS and the ROS production was quantitated immediately by measuring the green fluorescence using the EVOS® FL Cell Imaging System (Life Technologies, Grand Island, NY).
Immunofluorescent Staining
Microglia were grown to 70% confluence in a glass petri dish and treated with 0.5 µg/ml of SARS-COV2 spike protein for 24 h. Standard immunofluoresecent staining procedures were followed. Cells were fixed for 10 min at RT using 4% formaldehyde, followed by permeabilization with ice-cold 90% methanol. Cells were then washed in 1X phosphate buffered saline (PBS) and treated with the following primary antibodies, Anti-ACE2 (E-11); mouse monoclonal (Cat # sc-390851, Santa Cruz Biotechnology, Inc.), Anti-HIF-1α mouse monoclonal (3C144); (Cat # sc-71247, Santa Cruz Biotechnology, Inc.), NOS (pan –NOS antibody, Cat #2977, rabbit polyclonal, Cell signaling technologies), TNF alpha antibody [TNF706] (Cat # GTX108585; mouse monoclonal, Gene Tex), and NLRP3 antibody (Cat # GTX00763, rabbit polyclonal, Gene Tex). The secondary antibodies used include fluorescence labeled Alexa Fluor® 647 goat anti-rabbit IgG (H+
L), Alexa Fluor®488 Anti-Rabbit Secondary Antibodies, and Alexa Fluor® 488 rabbit anti-mouse IgG, all obtained from Thermo Fisher Scientific, Grand Island, NY. DAPI (Cat #D1306 Thermo Fisher Scientific) was used to stain nuclei. The expression levels of ACE2, HIF-1α, NOS, TNFα and NLRP3 in SARSCOV-2 treated microglia were compared to an untreated control, and fluorescence imaging was done using the EVOS® FL Cell Imaging System (Life Technologies, Grand Island, NY). The intensity of the fluorescent signal was quantitated using the ImageJ software (National Institutes of Health, Bethesda, MA, USA).
Mitochondrial Staining in Microglia using the MitoTracker™ Probe
Microglial cells (5000cells/ 35 mm dish) were plated on a glass bottom petri dish filled with culture medium, once attached, cells were treated with 0.5 µg/ml of SARS-COV2 spike protein for 24 h, pre-warmed (37 °C) staining solution containing MitoTracker™ Red CMXRos dye (Thermo Fisher Scientific) was added and cells were incubated for 15 min at 37 °C, after staining was complete, staining solution was replaced with fresh pre-warmed media or buffer and MitoTracker™ Red CMXRos dye staining was imaged using the EVOS® FL Cell Imaging System (Life Technologies, Grand Island, NY). The intensity of the fluorescent signal was quantitated using the ImageJ software (National Institutes of Health, Bethesda, MA, USA).
Mitochondrial Respiration
Microglial mitochondrial energetics was determined using the XFe24 extracellular flux analyzer (Seahorse Bioscience, North Billerica, MA). Microglial cells were seeded in a Seahorse 24-well tissue culture plate at an optimized concentration of 50,000 cells per well. Cells were treated with 0.5 µg/ml SARS-COV2 spike protein or 5 µl/ml of heat inactivated SARS-Coronavirus 2, isolate for 24 h. Basal OCR and extracellular acidification rate (ECAR) were detected in the presence of physiological concentrations of 10 mM glucose, 1 mM pyruvate and 2 mM glutamine. Next, mitochondrial ATP dependent respiration, uncoupled respiration, and non-mitochondrial respiration were assessed after the addition of 1 µM oligomycin, 1.5 µM carbonylcyanide-p-trifluoromethoxyphenylhydrazone (FCCP), and the combination of 0.5 µM each of antimycin A and rotenone, respectively. All experiments were performed using 5 wells per treatment.
BioLegend's LEGENDplex™ Cytokine Assay
We used the LEGENDplex™ Cytokine assay (BioLegend San Diego, CA) to determine the levels of cytokines in the culture supernatants of the microglial cultures treated with 0.5 µg/ml of SARS-COV 2 recombinant Spike protein. The LEGENDplex™ Cytokine assay is a bead-based immunoassay where the soluble analyte is captured between two antibodies. Beads are conjugated with a specific antibody on the surface which serves as the capture bead for that particular analyte. When a selected panel of capture beads are mixed together and incubated with an unknown sample containing target analytes, each analyte will be bound by its specific capture bead. After washing, biotinylated detection antibodies are added and each detection antibody will bind to its specific analyte bound on the capture beads, thus forming capture bead-analyte-detection antibody sandwiches. Streptavidin–phycoerythrin (SA-PE) is subsequently added, which will bind to the biotinylated detection antibodies, providing fluorescent signal with intensities in proportion to the amount of bound analyte. For each bead population, the PE signal fluorescence intensity is then quantified using a flow cytometer. The concentration of a particular analyte is determined based on a known standard curve using the LEGENDplex™ data analysis software.
Non-invasive Optical Nanoscopy
Changes in mitochondrial morphology were observed using the 3D Cell Explorer (Nanolive, Nexus Scientific LLC). We performed non-invasive and label free imaging and the 3D cell images were generated with Nanolive software based on the refractive index measured. Nanolive images were produced using a 60×
microscopy objective and class 1 low power laser with wavelength of 520 nm. Nanolive imaging captured the real time response of mitochondria to drug treatment at high frequencies without experimental artefacts proving information about mitochondrial morphology and cell health. We obtained nanolive images of microglia treated with 0.5 µg/ml of SARS-COV2 spike protein or 5µl/ml of heat inactivated SARS-Coronavirus 2, isolate for 3 h and observed morphological changes in microglia.
Raman Spectroscopy in Microglia
Raman spectra were acquired by a commercial Raman microspectroscope (HORIBA XploRA PLUS). Our Raman spectrometer is equipped with a 1024×
256 TE air cooled CCD chip (pixel size 26 microns, temp -60 °C). HORIBA LabSpec6 software was used for data acquisition, fluorescent background removal, baseline correction, and peak fitting. We used 20
×
Olympus immersion objective, 532 nm excitation laser wavelength at 100% power, 1800 gr/mm grating for spectra acquisition. Entrance slit was set at 50 µm, producing an approximate spectral resolution of 4 cm
−
1.1 We used a stage top incubator chamber (IBIDI Nanolive Heating System/Temperature Controller/Gas Incubation System) mounted on a three-dimensional translation stage on the microscope to keep the environment around samples in a humidified atmosphere (5% CO2, 95% air) at 37 °C for live cells Raman imaging. The chamber allows continuous monitoring of the spectral features of SARS-COV2 treated microglial cultures for the 3 h duration of the experiment.
Statistical Analysis
Data was analyzed using Graph Pad Prism 5.0 (GraphPad Software La Jolla, CA). Results are expressed as mean±
SD and for comparisons between control and treated groups, the “t-test” was used for parametric data and Mann–Whitney test for nonparametric data. A p value was
<
0.05 was considered as a statistically significant difference. A minimum of n
=
5 Raman hyperspectral datasets were obtained per sample, following which, the fluorescence background signal was subtracted from the Raman spectra at each pixel in the image by a modified polyfit fluorescence removal technique.
Results
Effect of SARS-COV2 on Cell Viability of Microglia
Figure 1A shows 100% viability in microglial cells treated with 0.5 µg/ml of SARS-COV 2 recombinant Spike protein or 5 µl/ml of heat inactivated SARS-Coronavirus 2, isolate at 24 h and a 95% and 85% cell viability corresponding to a 5% and 14% decrease (p=
NS) in microglia treated with SARS-COV2 spike protein and heat inactivated SARS-Coronavirus 2, isolate at 48 h post treatment, both indicating that both treatments did not result in any significant cell toxicity at the exposure time and concentrations used in the in-vitro assays.
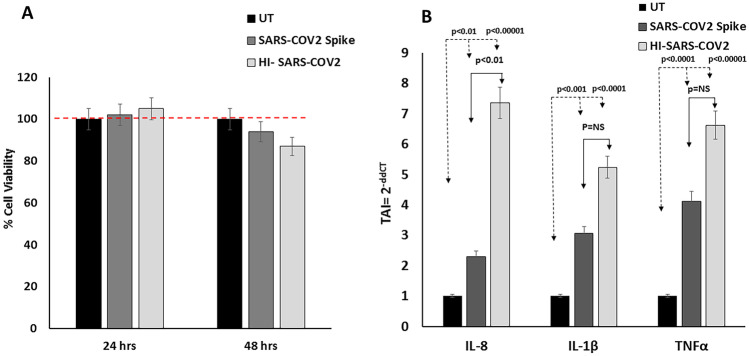
A; Effect of SARS-COV2 spike protein/Heat inactivated SARS-COV2 virus on Cell Viability of microglia. Results of the CCK-8 cell viability experiments, showing no significant difference in cell viability at 24–48 h post treatment with SARS-COV2 spike protein/Heat inactivated SARS-COV2. Data (Mean±
SD) represent % cell viability calculated with respect to the untreated control from 3 separate experiments done in triplicate. B; Effect of SARS-COV2 spike protein /heat inactivated SARS-COV2 virus on IL-8, IL1β and TNF-α gene expression in microglia as quantitated by QPCR. Results are expressed as the mean
±
SD from separate experiments (n
=
3) done in triplicate. A p value of
<
0.05 is considered a statistically significant difference
Effect of SARS-COV2 on the Gene Expression of Pro-Inflammatory Cytokines in Microglial Cells
Our data (Fig. 1B) shows that treatment of microglia with SARS-COV 2 Spike protein for 48 h leads to a significant increase in the gene expression levels of the pro-inflammatory cytokines IL-8 (TAI=
2.3
±
0.21, 1.3-fold increase p
<
0.01), IL-1β (TAI
=
3.05
±
0.19, 2.05-fold increase p <
0.001), TNF-α (TAI
=
4.12
±
0.23, 3.12-fold increase p
<
0.0001) as compared to the untreated control (TAI
=
1.0
±
0.08). Treatment with the HI SARS-COV2, isolate for 48 h also significantly increased the gene expression levels of IL-8 (TAI
=
7.36
±
0.52, 6.3 fold increase p
<
0.00001), IL-1β(TAI
=
5.24
±
0.33, 4.2 fold increase p
<
0.0001), TNF-α (TAI
=
6.62
±
0.48, 5.6 fold increase p
<
0.00001) as compared to the untreated control (TAI
=
1.0
±
0.08). Statistical comparison were made for gene expression levels of IL-8, IL-1β and TNF-α between SARS-COV 2 Spike protein and the HI SARS-COV2 treated microglia, and only IL-8 gene expression was significantly higher in the HI SARS-COV2 treated microglia. No significant differences were obtained in the IL-1β and TNF-α gene expression levels between SARS-COV 2 Spike protein and the HI SARS-COV2 treated microglia.
Effect of SARS-COV2 on Levels of Secreted Cytokines in Microglial Cells
We examined the levels of key cytokines in the culture supernatants of the microglial cultures treated with 0.5 µg/ml of SARS-COV 2 recombinant spike protein using the LEGENDplex™ bead-based cytokine immunoassay. Table Table11 shows that the levels of cytokines IL1β, TNF-α, IL-6, IL-10, IL-12, IL-17A, IL-23 and IL-33 in the culture supernatants of the microglial cultures treated with 0.5 µg/ml of SARS-COV 2 recombinant spike protein were significantly higher than the levels of these cytokine in the untreated control cultures.
Table 1
Effect of SARS-COV2 spike protein on levels of pro-inflammatory cytokines
Microglia | IL-1β | TNF-α | IL-6 | IL-10 | IL-12 | IL-17A | IL-23 | IL-33 |
---|---|---|---|---|---|---|---|---|
Conc units | pg/ml | pg/ml | pg/ml | pg/ml | pg/ml | pg/ml | pg/ml | pg/ml |
UT | 11.98![]() ![]() | 3.15![]() ![]() | 1622.7![]() ![]() | 7.12![]() ![]() | 4.29![]() ![]() | 0.89![]() ![]() | 2.89![]() ![]() | 26.04![]() ![]() |
SARS-COV2 spike | 14.12![]() ![]() | 12.91![]() ![]() | 58,880.65![]() ![]() | 16.41![]() ![]() | 9.29![]() ![]() | 0.41![]() ![]() | 7.83![]() ![]() | 33.95![]() ![]() |
p value | 0.04 | 0.0001 | 0.000001 | 0.002 | 0.01 | 0.01 | 0.001 | 0.05 |
Effect of SARS-COV2 on ACE2 Expression in Microglial Cells
Our immunofluorescent staining data (Fig. 2; panel a) showed that when compared to the untreated control, treatment of microglia with recombinant SARS-COV2 spike protein lead to a 50% increase (p<
0.05), in the ACE2 expression [Fig [Fig2;2; Panel a (i-iii); Panel b (i-iii); Panel c (i-iii); Fig. Fig.3;3; Panel a(i-iii)].

Effect of SARS-COV2 spike protein on ACE2, HIF-1α and NOS expression in microglia. Results from the Immunofluorescence staining experiments showing representative images: Panel a: ACE2 expression, Panel b: HIF-1α and Panel c: NOS that includes the following (i) Untreated control; (ii) Recombinant SARS-COV2 spike protein treated (iii) Quantitative histogram. Red stain in Panel A and B is Alexa Fluor 647 dye and Green staining in Panel C is the Alexa Fluor 488 dye. Expression was quantitated using ImageJ software and statistical comparisons were done based on a comparison with the respective untreated control. A p value of < 0.05 is considered a statistically significant difference. Standard immunostaining protocols were followed. Data are representative images from 3 separate experiments done in triplicate
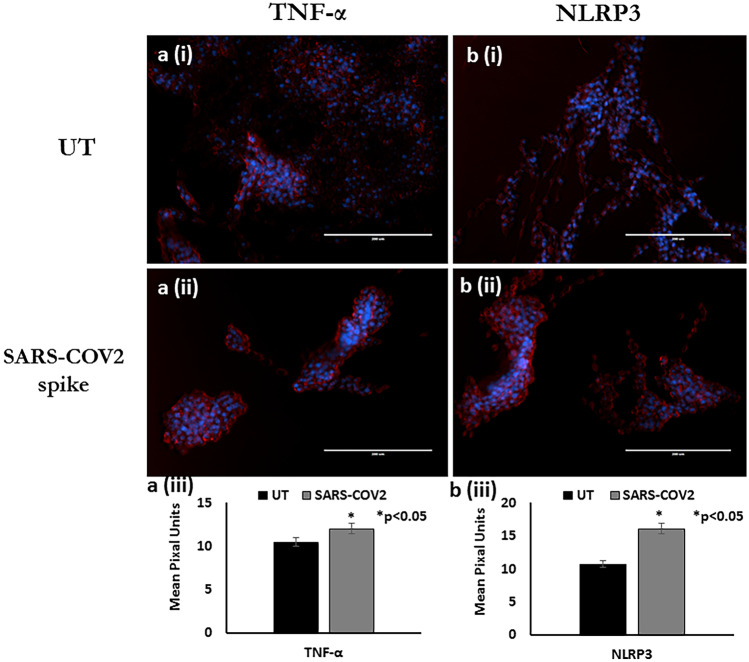
Effect of SARS-COV2 spike protein on NLRP3 and TNF- α expression in microglia. Results from the Immunofluorescence staining experiments showing representative images: Panel a: NLRP3 expression, Panel b: TNF- α expression that includes the following (i) Untreated control; (ii) Recombinant SARS-COV2 spike protein treated (iii) Quantitative histogram. Red stain in Panel A and B is Alexa Fluor 647 dye. Expression was quantitated using ImageJ software and statistical comparisons were done based on a comparison with the respective untreated control. A p value of<
0.05 is considered a statistically significant difference. Standard immunostaining protocols were followed. Data are representative images from 3 separate experiments done in triplicate
Effect of SARS-COV2 on HIF-1α Expression in Microglial Cells
Our immunofluorescent staining data (Fig. 2; panel b) showed that when compared to the untreated control, treatment of microglia with recombinant SARS-COV2 spike protein leads to a 57% increase (p<
0.05) in HIF-1α expression [Fig. 2; Panel b(i-iii)].
Effect of SARS-COV2 on NOS Expression in Microglial Cells
Our immunofluorescent staining data (Fig. 2; Panel C) showed that when compared to the untreated control, treatment of microglia with recombinant SARS-COV2 spike protein leads to a 62% increase (p<
0.05) in the NOS expression [Fig. 2; Panel c(i-iii)].
Effect of SARS-COV2 on Caspase 3/7 Expression in Microglial Cells
We observed that microglial cells treated with 0.5 µg/ml of SARS-COV 2 recombinant spike protein for 24 h, showed increased apoptosis (1.4-fold increase, p<
0.01) as evident by increased Caspase 3/7 expression as compared to the untreated control [Fig. [Fig.4;4; Panel a(i-iii)].
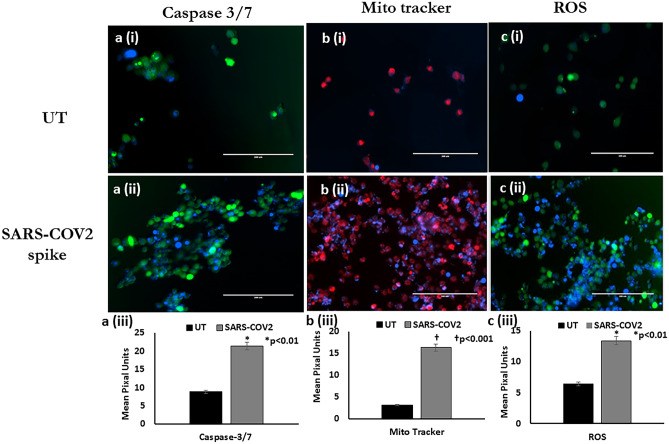
Effect of SARS-COV2 spike protein on Caspase 3/7, MitotrackerTM and ROS expression in microglia. Results from the fluorescence staining experiments showing representative images: Panel a (i-iii): Caspase 3/7 expression, Panel b (i-iii): Mitotracker and Panel c (i-iii): ROS that includes the following (i) Untreated control; (ii) Recombinant SARS-COV2 spike protein treated (iii) Quantitative histogram. Green stain in Panel A and C is a green fluorescence protein dye and Red staining in Panel B is the Red CMXRos dye. Expression was quantitated using ImageJ software and statistical comparisons were done based on a comparison with the respective untreated control. A p value of<
0.05 is considered a statistically significant difference. Standard manufacture provided protocols were followed. Data are representative images from 3 separate experiments done in triplicate
Effect of SARS-COV2 on Mitochondrial Activation in Microglial Cells
We observed increased mitochondrial activation (1.4-fold increase, p<
0.01) as quantitated by the fluorescent intensity of the MitoTracker™ Red CMXRos dye in microglial cells treated with 0.5 µg/ml of SARS-COV 2 recombinant spike protein for 24 h as compared to the untreated control [Fig. [Fig.4;4; Panel b(i-iii)].
Effect of SARS-COV2 on Reactive Oxygen Species (ROS) Production in Microglial Cells
We quantitated the fluorescent intensity of the ROS using the CM-H2DCFDA reagent in microglial cells treated with 0.5 µg/ml of SARS-COV 2 recombinant spike protein, our data shows a significant increase in ROS production (1.4-fold increase, p<
0.01) in SARS-COV2 protein treated cells as compared to the untreated control [Fig. [Fig.4;4; Panel c(i-iii)].
Effect of SARS-COV2 on Activation of the Inflammasome in Microglial Cells
Our immunofluorescent staining data (Fig. (Fig.3;3; Panel A-B) showed that compared to the untreated control, treatment of microglia with recombinant SARS-COV2 spike protein lead to a 64% increase (p<
0.05) in the expression of NLRP3 [Fig. [Fig.3;3; Panel A(i-iii)] which suggests inflammasome activation which further amplifies pro-inflammatory cytokines secretion as indicated by a 30% increase, (p
<
0.05) in expression of TNFα [Fig. [Fig.3;3; Panel B(i-iii)].
Effect of SARS-COV2 on Mitochondrial Bioenergetics in Microglial Cells
Figure 5 shows the oxygen consumption rate (OCR) is measured before and after the addition of different inhibitors to derive several parameters of mitochondrial respiration in microglial treated with 0.5 µg/ml of SARS-COV 2 recombinant spike protein or heat inactivated SARS-COV2 and comparators were the untreated microglial cells. Our data shows that treatment with both SARS-COV 2 recombinant spike protein and HI SARS-COV2 resulted in a significant increase in mitochondrial respiration. Figure 6 shows a significant increase in both basal (67% increase, p<
0.01) and maximal (149% increase, p
<
0.001) OCR in response to 0.5 µg/ml of SARS-COV 2 recombinant spike protein in microglial cells as compared to the respective untreated controls. Treatment of microglial cells with HI SARS-COV2 also resulted in significant increase in both basal (73% increase, p
<
0.01) and maximal (140% increase, p
<
0.001) OCR as compared to the respective untreated controls.

Measurement of oxygen consumption rate (OCR), in microglia treated with recombinant SARS-COV2 spike protein/ heat inactivated SARS-COV2. OCR was measured in real time, under basal condition, and in response to mitochondria inhibitors: oligomycin (Oligo, 1 μM), cyanide‐4‐(trifluoromethoxy)phenylhydrazone (FCCP, 1.5 μM), and antimycin A plus rotenone (Rot/AA, 0.5 μM). Data represent mean and standard deviation from 3 separate experiments with 5 replicates /sample. A “t- test” was used for statistical analysis. P values are reported in the figure

A–C; Representative Nanolive images of microglia treated with SARS-COV2 spike protein /Heat inactivated SARS-COV2 virus showing SARSCOV2 induced changes in Microglial morphology. The yellow box inlet highlight region of interest showing increased vacuoles, increase in membrane ruffling, micropinocytosis and mitochondrial fragmentation. The Nanolive scope used 60×
microscopy objective and class 1 low power laser with wavelength of 520 nm. Data are representative images from 3 separate experiments done in triplicate. 6D: Representative Raman spectra of microglia treated with SARS-COV2 spike protein /heat inactivated SARS-COV2
Effect of SARS-COV2 on Microglial Morphology
Figure 6A, shows a representative image of morphological features of an untreated microglial cell consistent with healthy immortalized microglia, with some ruffling which was predominantly restricted to cell boarder and presence of long filamentous mitochondria and/or endoplasmic reticulum. Figure 6B, shows a representative image of a microglial cell treated with recombinant -SARS-COV 2 spike protein showed increase in the apoptotic cells and fragmentation of organelles, including mitochondria. Figure 6C shows representative images of a microglial cell treated with HI SARS-COV2 and showed increased vertical ruffling, consistent with cells reacting to foreign particles and increased vacuoles, consistent with increase in membrane ruffling, micropinocytosis and mitochondrial fragmentation.
Use of Raman Spectroscopy for Prognostic Evaluation of Mitochondrial Function in SARS-COV2 Treated Microglial Cells
Figure 6D shows a representative Raman spectral image of microglia treated with 0.5 µg/ml of SARS-COV 2 recombinant spike protein or the heat inactivated SARS-COV2 using the untreated microglial cells as comparators. Figure 6D represents an average of all spectra which had a visible phenylalanine peak at~
1004 cm-1. Notable differences are seen in the peak centered around 800 cm-1, 1044 cm-1 and 1650 cm-1. Peak 800 cm-1 is a set of peaks related to pyrimidine bases, DNA, RNA and Peak 1044 cm-1 correspond to phosphate groups and Peak 1650 cm-1 corresponds to Amide I.
Figure Figure7A-C,7A-C, shows spectra which is restricted to values above 900 cm-1 and SVD analysis conducted. Panel A shows SVD analysis and shows the differences between the untreated control and the recombinant SARS-COV2spike protein /HI SARS-COV2 treated microglia. Panel B shows dominant SVD 1 component includes: 1044 cm-1 (phosphate groups), 1446 cm-1 (CH deformation) and 1650 cm-1 (Amide I/ C=
C stretching of lipids) and Panel C shows the dominant SVD 2 component includes: 1442 cm-1 (fatty acids) and 1650 cm-1 (Amide I/ C
=
C stretching of lipids). SVD analysis shows that the control cluster is well separated from recombinant SARS-COV2 spike protein treated cluster, but slightly closer to the HI SARS-COV2 treated cluster in SVD scatter plot, which suggest that the microglia treated with SARS-COV2 spike protein have bigger chemical composition changes as compare to microglia treated with HI SARS-COV2

Single Value Decomposition (SVD) analysis of Raman spectra of microglia treated with SARS-COV2 spike protein /heat inactivated SARS-COV2. Spectra was restricted to values above 900 cm -1. SVD analysis decomposes a matrix (stacked Raman spectra) into matrix U, ∑ and VT where U, V are real/complex unitary matrix and ∑ was a positive real diagonal matrix. Matrix U provides the critical spectra information and matrix V given the coordinate of SVD cluster for each spectrum base on matrix U. SVD scatter plot are plotted with matrix V where each data point represents one Raman spectrum. The spectra with similar feature form a cluster but spectra with different feature get separated in SVD scatter plot. The feature pickup for separation depends on the matrix U and is plotted as SVD1 and SVD2. SVD1 correspond to the x-axis separation and SVD 2 correspond to y-axis separation in SVD scatter plot
Discussion
The involvement of the CNS manifestation in COVID-19 patients is becoming increasing clear with continued neurological symptoms in long haulers of COVID-19 disease, implying an urgent need to examine the mechanisms that underlie the neuropathogenesis process (Solomon et al. 2020; Baig 2020; Graham et al. 2021). Given, the neurological manifestations of SARS-COV2, it is more evident that SARS-COV2 is potentially neurotropic in humans and neuroinvasion may occur by transsynaptic transfer across infected neurons, entry via the olfactory nerve, via the infection of brain microvascular vascular endothelial cells or leukocyte transmigration across the blood–brain barrier (BBB) (Arbour et al. 2000; Li et al. 2013; Bohmwald et al. 2018; Paniz-Mondolfi et al. 2020; Baig et al. 2020). Microglia are central players of the CNS homeostasis maintenance and inflammatory response; therefore, our goal was to examine the SARS-COV2 induced changes in microglial morphology, the mechanisms that underlie the neuroinflammatory response and molecular signatures resulting in modulation of microglial function.
Figure 1A shows that the dose of SARS-COV2 spike protein and HI SARS-COV2 at which the microglia were treated no significant toxicity at 24 h, but a small decrease in cell viability with increase in time exposure to 48 h, thus all of our subsequent assays are conducted between the 24–48-h time points. Figure 1B shows a significant increase in the gene expression levels of proinflammatory cytokines IL-8, IL-1β and TNF-α. Table 1 shows a significant increase in levels of pro-inflammatory cytokines in SARS-COV2 treated microglial cultures. Additional immunofluorescence data shows a SARS-COV2 induced increase in TNF-α expression [Fig. [Fig.3,3, Panel a(i-iii)]. Several studies have reported significant increase in the systemic levels of pro-inflammatory cytokines (Ruan et al. 2020; Marton et al. 2021; Rowaiye et al. 2021). Hyper-inflammatory response induced by SARS-COV2 is a major cause of disease severity and death and studies have reported significant increase in the systemic levels of pro-inflammatory cytokines such as IL-2, IL-10, IP-10, monocyte chemoattractant protein-1 (MCP-1), and tumor necrosis factor-α (TNFα) (Merad and Martin 2020; Ruan et al. 2020; Marton et al. 2021; Rowaiye et al. 2021). The COVID-19-related cytokine response was quite distinct from the traditional cytokine storm associated with sepsis with sustained elevated cytokine levels over days and weeks, and relative absence of coordination between cytokines. [Liu et al. 2020]. IL-8 plays a key role in the recruitment and activation of neutrophils, commonly elevated in patients with COVID-19. Elevated TNF-α, contributes to organ damage and had significant prognostic value. IL-8, is associated with survival time and IL-1β is believed to have limited predictive value for disease outcome.
In the CNS, basal levels of IL1β, TNFα, and IL6 are necessary for optimal neurons and glial function to maintain CNS homeostatis (Camacho-Arroyo et al. 2009; Borsini et al. 2015). Viral infection results in cytokine induced stimulation of neuroendocrine responses via the activation of the hypothalamus–pituitary–adrenal (HPA) axis and the elevation of the core body temperature, ultimately promoting disease-symptoms and elevated cytokine levels in the brain parenchyma which serve as mediators of neurotoxic and neurodegenerative pathology (Camacho-Arroyo et al. 2009; Borsini et al. 2015). In the context of COVID-19, pathological excess of cytokines may lead to vascular remodeling and BBB leakage, increasing the entry of danger-associated molecular pattern (DAMPs) and pathogen-associated molecular pattern (PAMPs) associated with the peripheral viral infection. Cytokine storm is a distinct immunological character of COVID-19 infection resulting in significant neuro-inflammation that is attributed to an increase in pro-inflammatory cytokines. In the CNS during SARS-COV 2 infection, the elevated level of cytokines IL1β, TNF, and IL6 serve as a mediator of neurotoxic and neurodegenerative pathology (Gonçalves de Andrade et al. 2021). Cytokine storm is the hyper-induction of pro-inflammatory cytokines, which is major hallmark of SARS-COV2 infection. Activation of the innate and adaptive immune system results in producing pro-inflammatory cytokines and a feedback loop of inflammation. Recent studies have shown that the cytokine response induced by SARS-COV2 is different from the cytokine storm caused by other respiratory viruses, as SARS-COV2 does not always induce significant amounts of cytokines such as IL-2, IL-10, IL-4 but causes dysregulation of the Type-I interferon (IFN) response and its associated cytokine activation (Olbei et al. 2021; Channappanavar and Perlman 2017)). The main effectors of the Type-I IFN signaling are IFN-α and IFN-β, which activate cytokines, such as IL-12 and IFN-γ (Kang et al. 2018; Bhardwaj et al. 1996). We did not observe significant change in IL-2, IL-4, but observed a significant increase in IL-6, IL-10, IL-12, IL-17A, IL-23 and IL-33 in SARS-COV2 treated microglia as compared to the untreated control. Our data suggests that the SARS-COV2 neuropathogenesis process may be driven by different inflammatory response patterns than other respiratory viruses and that the cytokine response during viral infection may be a dynamic process, that may results in modulation of levels of different members of the cytokine family during the course of the infection, in order to maintain a homeostatic balance in the CNS, however, should the cytokine dysregulation continue and the excessive cytokine production contributes to brain tissue inflammation and the neuropathogenesis of COVID-19.
We observed increased gene expression of IL-8, IL-1β and TNF-α in both SARS-COV2 spike protein and heat inactivated SARS-COV2 treated microglial cells, as compared to the untreated control, however only significantly higher IL-8 cytokine expression was seen in the heat inactivated SARS-COV 2 treated microglia when compared to the microglia treated with SARS-COV2 spike protein. Although, we did not measure cytokine levels from the heat inactivated SARS-COV 2 treated microglia, the increased IL-8 gene expression levels quantitated by QPCR suggests that we would also observe an increase in other cytokine levels concurrent with what we observed in SARS-COV2 treated microglial cells. Although both SARS-COV2 spike protein and heat inactivated SARS-COV2 treatment of microglia may result in a robust cytokine response, modulation of cytokine levels may be variable given that the cytokine release is a dynamic process. Predictive biomarkers of pathogenic inflammation may help guide targetable immunotherapy that may have significant benefit in controlling neuro-inflammation and the consequent SARS-COV2 associated neuropathology.
Inflammatory response is associated with the assembly/activation of a cell-intrinsic defense platform known as the inflammasome. Several reports suggest that the main role of NLRP3 inflammasome in the pathogenesis of SARS-COV2 is its overactivation contributing to cytokine storm (Freeman and Swartz 2020; Ratajczak and Kucia 2020; Fu et al. 2020). We observed a significant increase in the expression of NLRP3 expression on treatment with SARS-COV2 (Fig. (Fig.3b)3b) indicating that SARS-COV2 induces inflammasome activation in microglia. Several studies have shown that NLRP3 inflammasome is activated in response to SARS-COV2 infection (Xu et al. 2020; Irrera et al. 2020; Freeman and Swartz 2020; van den Berg and Te Velde 2020). The NLRP3 inflammasome promotes inflammation via cleavage and activation of key inflammatory molecules including active caspase-1 (Casp1p20), IL-1β, and IL-18 (Rodrigues et al. 2021). Our data shows that NLRP3 inflammasome is activated in response to SARS-COV2 treatment in human microglia.
ACE-2 is an entry receptor for SARS-CoV2. Efficient replication of SARS-CoV2 depends on the expression level of ACE-2 (Hoffmann et al. 2020). Basal level of ACE2 expression in CNS cells is sufficient for viral entry (Puelles et al. 2020). We observed a significant increase in the ACE2 expression [Fig. 2, Panel a(i-iii)] in microglia when treated with SARS-COV2. In neuronal cell cultures, ACE2 is expressed both on the surface membrane and in the cytoplasm and its prevalent expression in the brain has the potential to infect neurons and glial cells throughout the CNS (Hoffmann et al. 2020; Reynolds and Mahajan 2021). We and others have reported that SARSCOV2 induces increased ACE2 expression by brain microvascular endothelial cells (Reynolds and Mahajan 2021; Buzhdygan et al. 2020) and increases BBB permeability to SARS-COV2.
We observed a significant increase in the HIF-1α expression [Fig. 2, Panel b(i-iii)] in microglia when treated with SARS-COV2 that indicates that an accumulation of HIF-1α occurs in microglia, this is believed to be due to increased HIF-1α expression as well as inhibited proteasome degradation (Vassilaki and Frakolaki 2017). Hypoxia and HIF-1α stabilization may trigger or enhance the cytokine storm. Hypoxia plays important roles at the inflammation site by inducing pro-inflammatory cytokines production and induces HIF-1α expression (Serebrovska et al. 2020). Hypoxia and HIF-1α can either stimulate or inhibit cytokine-mediated inflammatory response. HIF-1α regulates important cellular processes such as cell proliferation, metabolism and angiogenesis. Hypoxia may trigger mitochondrial dysregulation, acidosis, altered mitochondrial membrane permeability, and eventually insufficiency of ATP biosynthesis inducing cell death. Modulation of the mitochondrial respiratory chain in hypoxia occurs via the generation of H2O2, and other reactive oxygen /nitrogen species (Zorov et al. 2014).
Mitochondrial dysfunction and associated oxidative stress drive the production of pro-inflammatory cytokines that, in turn, play an important role in the immune response. (Arnoult et al. 2011). Our data shows that SARS-COV2 treatment increases mitochondrial activation [Fig. 5, Panel b(i-iii)], ROS [Fig. [Fig.4,4, Panel c(i-iii)] and NOS [Fig. 2, Panel c(i-iii)] activation. Mitochondrial dysfunction occurs when oxidative modification of the respiratory chain complexes occurs and this event amplifies and promotes further oxidative damage. Our data suggest that SARS-COV2 treatment of microglial cells results in increased mitochondrial respiration which affects mitochondrial function, and could influences SARS-COV2 intracellular survival or enable evasion of host immune system. Mitochondrial manipulation by SARS-CoV-2 results in increased oxidative stress and increased cytokine production. A recent report indicate that mitochondrial dysfunction contributes to a systemic immune response in COVID-19 pathogenesis (Ajaz et al. 2021), thus given that microglia are the immune cells of the CNS, the SARS-COV2 induced mitochondrial dysfunction in microglia observed in our study could potentially drive Neuro-COVID progression.
Elevated ROS production, upregulates nitric oxide synthase expression, indicating development of oxidative and nitrosative stress impacting immune function, apoptosis, inflammatory response, as well as organ and tissue dysfunction in COVID-19 infection (Chernyak et al. 2020; Chang et al. 2021). H2O2 triggers the expression of many genes that upregulate pro-inflammatory cytokines, such as IL-1β and TNFα, and inducible nitric oxide synthase (iNOS) via activation of the NF-κB pathway (Nanduri 2008). These pro-inflammatory cytokines activate macrophages, neutrophils, endothelium cells via NADPH oxidase (NOx) to produce more superoxide and H2O2 and these superoxide radicals and NO are toxic to mitochondria (Tan et al. 2016). In COVID-19 patients it is likely that the inability of the mitochondria to utilize oxygen despite normal tissue oxygen saturation may be exacerbated (Cecchini and Cecchini 2020). SARS-COV2 induced excessive ROS production results in the development of oxidative stress followed by cell and tissue damage (Chernyak et al. 2020). The interaction between ROS and cytokines generates a self-sustaining cycle between cytokine storm and oxidative stress production that eventually leads to multiorgan failure in patients with COVID-19. Alternately, NO also inhibits the replication cycle of SARS-COV2 suggesting that the production of NO by inducible nitric oxide synthase (iNOS) results may produce an antiviral effect (Fang et al. 2021) and could be used as a therapeutic to kill the virus.
Evidence suggests that there is a direct relationship between apoptosis and COVID‐19 pathogenicity and that the induction of apoptosis is a hallmark of SARS-COV2 infection. (Donia and Bokhari 2021). Our data shows that SARS-COV2 treatment increased the expression of Caspase3/7 potentially initiate microglial cell apoptosis [Fig. 4, Panel a(i-iii)], Apoptosis triggered by SARS-COV2 has a complex role in host antiviral immunity, and might facilitate the viral clearance or act as a mechanism for virus-induced tissue injury and disease progression [Kaminskyy and Zhivotovsky (2010); Ampomah and Lim (2020)]. SARS-COV2 induces apoptosis via the extrinsic apoptotic pathway Ren et al. (2020).
Mitochondria play a central role in the COVID neuropathogenesis process by virtue of the fact that it is a major reactive oxygen species (ROS) producer, and a target of ROS. The proximity of mtDNA to the ROS-generating electron transport chain makes mtDNA susceptible to oxidative damage. Our data shows that treatment with both SARS-COV 2 spike protein and HI SARS-COV2 resulted in a significant increase in mitochondrial respiration. Figure 5 shows a significant increase in both basal and maximal OCR in response to both SARS-COV 2 recombinant spike protein and HI SARS-COV2. Our results suggest that SARS-COV2 increased mitochondrial respiration in microglia which could lead to bioenergetic dysfunction and production of ROS and increased oxidative stress. Host responses against SARSCOV2 depend on mitochondrial functions and mitochondrial DNA itself acts as a danger-associated molecular pattern (DAMP) and mitochondrial dysfunction drives a systemic immune response in COVID-19 pathogenesis. (Singh et al. 2020). Since mtDNA encodes vital components of the OXPHOS and protein synthesis machinery, oxidative damage-induced mtDNA mutations that impair either the assembly or the function of the respiratory chain will, in turn, further accumulation of ROS, which results in a vicious cycle leading to energy depletion in the cell and ultimately cell death. Our results show that SARS-COV2 increased mitochondrial respiration in microglia which could lead to bioenergetic dysfunction and production of ROS and increased oxidative stress.
Figure 6 (Panel A-C) shows recombinant SARS-COV 2 spike protein and HI SARS-COV 2 induced changes to microglial morphology and Nanolive images show fragmentation of cell organelles such endoplasmic reticulum, increased vacuoles, increase in membrane ruffling, micropinocytosis and mitochondrial fragmentation, all of which are indicative of cellular stress and apoptosis initiation. Figure 6D shows Raman spectra for microglial cells treated with recombinant SARS-COV 2 spike protein and HI SARS-COV 2. We used single value decomposition (SVD) to analyze the Raman spectra. (Fig. 7A-C) The SVD allow us to differentiate the Raman spectra and provided important differences between the spectra. Our data indicate that chemical changes in proteins, observed by Raman measurements, precede morphological changes.
Thus, our data suggests that SARS-COV2 induces a significant inflammatory response, increased oxidative stress, inflammasome activation and mitochondrial dysfunction in microglial cells, all of which contribute to COVID associated neuropathology. This study provides important mechanistic insights into SARS-COV2 induced mitochondrial dysfunction which underlies COVID-19 associated neuropathology.
Acknowledgements
Authors wish to acknowledge the receipt of the following reagents through BEI Resources. These reagents were produced under HHSN272201400008C and obtained through BEI Resources, NIAID, NIH and were specifically procured for this study: 1) Spike Glycoprotein (Stabilized) from SARS-Related Coronavirus 2, Wuhan-Hu-1 with C-Terminal Histidine Tag, Recombinant from Baculovirus, (NR-52308); 2) Heat Inactivated SARS- Coronavirus 2, Isolate USA-WA1/2020, (NR-52286).
Funding
This study was funded by NIH- National Institute of Drug abuse (Grant # 5R01DA047410-02) awarded to Supriya D Mahajan & Alexander Khmaladze and NIAID Grant #5R01AI129649 awarded to Jessica L Reynolds.
Declarations
(X) Ethical approval: This article does not contain any studies with human participants or animals performed by any of the authors. This is an in-vitro cell culture study.
None of the Authors have any conflicts of interests.
Footnotes
The original online version of this article was revised: Author names Chean KT and Tubbesing KE needed to be deleted from the list of authors at their request.
Publisher's Note
Springer Nature remains neutral with regard to jurisdictional claims in published maps and institutional affiliations.
Change history
12/11/2021
A Correction to this paper has been published: 10.1007/s11481-021-10043-2
References
- Ajaz S, McPhail MJ, Singh KK, Mujib S, Trovato FM, Napoli S, Agarwal K. Mitochondrial metabolic manipulation by SARS-CoV-2 in peripheral blood mononuclear cells of patients with COVID-19. Am J Physiol Cell Physiol. 2021;320:C57–C65. 10.1152/ajpcell.00426.2020. [Europe PMC free article] [Abstract] [CrossRef] [Google Scholar]
- Al-Dalahmah O, Thakur KT, Nordvig AS et al (2020). Neuronophagia and microglial nodules in a SARS-COV2 patient with cerebellar hemorrhage. Acta Neuropathol Commun. 8(1):147. 10.1186/s40478-020-01024-2 [Europe PMC free article] [Abstract]
- Ampomah PB, Lim LHK. Influenza A virus-induced apoptosis and virus propagation. Apoptosis. 2020;25:1–11. 10.1007/s10495-019-01575-3. [Abstract] [CrossRef] [Google Scholar]
- Arbour N, Day R, Newcombe J, Talbot PJ (2000). Neuroinvasion by human respiratory coronaviruses. J Virol 74(19):8913–8921. 10.1128/JVI.74.19.8913-8921.2000 [Europe PMC free article] [Abstract]
- Arnoult D, Soares F, Tattoli I, Girardin SE (2011) Mitochondria in innate immunity. EMBO Rep 12: 901–910. 10.1038/embor.2011.157 [Europe PMC free article] [Abstract]
- Bachiller S, Jiménez-Ferrer I, Paulus A, Yang Y, Swanberg M, Deierborg T, Boza-Serrano A (2018) Microglia in neurological diseases: a road map to brain-disease dependent-inflammatory response. Front Cell Neurosci 12:488. https://www.frontiersin.org/article/10.3389/fncel.2018.00488 [Europe PMC free article] [Abstract]
- Baig AM. Deleterious Outcomes in Long-Hauler COVID-19: The Effects of SARS-COV2 on the CNS in Chronic COVID Syndrome. ACS Chem Neurosci. 2020;11(24):4017–4020. 10.1021/acschemneuro.0c00725. [Abstract] [CrossRef] [Google Scholar]
- Baig AM, Khaleeq A, Ali U, Syeda H. Evidence of the COVID-19 Virus Targeting the CNS: Tissue Distribution, Host-Virus Interaction, and Proposed Neurotropic Mechanisms. ACS Chem Neurosci. 2020;11(7):995–998. 10.1021/acschemneuro.0c00122. [Abstract] [CrossRef] [Google Scholar]
- Bertheloot D, Latz E, Franklin BS. Necroptosis, pyroptosis and apoptosis: an intricate game of cell death. Cell Mol Immunol. 2021;18:1106–1121. 10.1038/s41423-020-00630-3. [Europe PMC free article] [Abstract] [CrossRef] [Google Scholar]
- Beyrouti R, Adams ME, Benjamin L, Cohen H, Farmer SF, Goh YY, et al. Characteristics of ischaemic stroke associated with COVID-19. J Neurol Neurosurg Psychiatr. 2020;91:889–891. 10.1136/jnnp-2020-323586. [Europe PMC free article] [Abstract] [CrossRef] [Google Scholar]
- Bhardwaj N, Seder RA, Reddy A, Feldman MV. IL-12 in conjunction with dendritic cells enhances antiviral CD8+ CTL responses in vitro. J Clin Invest. 1996;98:715–722. 10.1172/JCI118843. [Europe PMC free article] [Abstract] [CrossRef] [Google Scholar]
- Bohmwald K, Gálvez NMS, Ríos M, Kalergis AM (2018) Neurologic alterations due to respiratory virus infections. Front Cell Neurosci 12(386):386 10.3389/fncel.2018.00386 [Europe PMC free article] [Abstract]
- Borsini A, Zunszain PA, Thuret S, Pariante CM (2015) The role of inflammatory cytokines as key modulators of neurogenesis. Trends Neurosci 38(3):145–57. 10.1016/j.tins.2014.12.006 [Abstract]
- Buzhdygan TP, DeOre BJ, Baldwin-Leclair A, Bullock TA, McGary HM, Khan JA, Razmpour R, Hale JF, Galie PA, Potula R, Andrews AM (2020) The SARS-CoV-2 spike protein alters barrier function in 2D static and 3D microfluidic in-vitro models of the human blood–brain barrier. Neurobiol Dis 146:105131. 10.1101/2020.06.15.150912 [Europe PMC free article] [Abstract]
- Camacho-Arroyo I, López-Griego L, Morales-Montor J. The role of cytokines in the regulation of neurotransmission. NeuroImmunoModulation. 2009;16(1):1–12. 10.1159/000179661. [Abstract] [CrossRef] [Google Scholar]
- Cecchini R, Cecchini AL. SARS-COV2 infection pathogenesis is related to oxidative stress as a response to aggression. Med Hypotheses. 2020;143:110102. 10.1016/j.mehy.2020.110102. [Europe PMC free article] [Abstract] [CrossRef] [Google Scholar]
- Channappanavar R, Perlman S. Pathogenic human coronavirus infections:causes and consequences of cytokine storm and immunopathology. Semin Immunopathol. 2017;39:529–539. 10.1007/s00281-017-0629-x. [Europe PMC free article] [Abstract] [CrossRef] [Google Scholar]
- Chernyak BV, Popova EN, Prikhodko AS, Grebenchikov OA, Zinovkina LA, Zinovkin RA. COVID-19 and Oxidative Stress. Biochemistry Biokhimiia. 2020;85(12):1543–1553. 10.1134/S0006297920120068. [Europe PMC free article] [Abstract] [CrossRef] [Google Scholar]
- Chang R, Abrar Mamun AD, Le NT. SARS-COV2 mediated endothelial dysfunction: the potential role of chronic oxidative stress. Front Physiol. 2021;11:1752. 10.3389/fphys.2020.605908. [Europe PMC free article] [Abstract] [CrossRef] [Google Scholar]
- Clark SA, Clark LE, Pan J, Coscia A, McKay L, Shankar S, Johnson RI, Brusic V, Choudhary MC, Regan J, Li JZ, Griffiths A, Abraham J. SARS-COV2 evolution in an immunocompromised host reveals shared neutralization escape mechanisms. Cell. 2021;184(10):2605–2617.e18. 10.1016/j.cell.2021.03.027. [Europe PMC free article] [Abstract] [CrossRef] [Google Scholar]
- Daniels BP, Holman DW, Cruz-Orengo L, Jujjavarapu H, Durrant DM, Klein RS (2014) Viral pathogen-associated molecular patterns regulate blood-brain barrier integrity via competing innate cytokine signals. MBio 5(5):e01476-14. 10.1128/mBio.01476-14 [Europe PMC free article] [Abstract]
- Dixon L, Varley J, Gontsarova A, Mallon D, Tona F, Muir D, et al. COVID-19-related acute necrotizing encephalopathy with brain stem involvement in a patient with aplastic anemia. Neurol Neuroimmunol Neuroinflamm. 2020;7:e789. 10.1212/NXI.0000000000000789. [Europe PMC free article] [Abstract] [CrossRef] [Google Scholar]
- Donia A, Bokhari H. Apoptosis induced by SARS-COV2: can we target it? Apoptosis. 2021;26:7–8. 10.1007/s10495-021-01656-2. [Europe PMC free article] [Abstract] [CrossRef] [Google Scholar]
- Fang W, Jiang J, Su L, Shu T, Liu H, Lai S, Ghiladi RA, Wang J. The role of NO in COVID-19 and potential therapeutic strategies. Free Radical Biol Med. 2021;163:153–162. 10.1016/j.freeradbiomed.2020.12.008. [Europe PMC free article] [Abstract] [CrossRef] [Google Scholar]
- Farfara D, Lifshitz V, Frenkel D. Neuroprotective and neurotoxic properties of glial cells in the pathogenesis of Alzheimer's disease. J Cell Mol Med. 2008;12(3):762–780. 10.1111/j.1582-4934.2008.00314.x. [Europe PMC free article] [Abstract] [CrossRef] [Google Scholar]
- Fatoba O, Itokazu T, Yamashita T. Microglia as therapeutic target in central nervous system disorders. J Pharmacol Sci. 2020;144(3):102–118. 10.1016/j.jphs.2020.07.004. [Abstract] [CrossRef] [Google Scholar]
- Freeman TL, Swartz TH. Targeting the NLRP3 Inflammasome in Severe COVID-19. Front Immunol. 2020;23(11):1518. 10.3389/fimmu.2020.01518. [Europe PMC free article] [Abstract] [CrossRef] [Google Scholar]
- Fu Y, Cheng Y, Wu Y. Understanding SARS-CoV2-mediated inflammatory responses: from mechanisms to potential therapeutic tools. Virol Sin. 2020;35(3):266–271. 10.1007/s12250-020-00207-4. [Europe PMC free article] [Abstract] [CrossRef] [Google Scholar]
- Gonçalves de Andrade E, Šimončičová E, Carrier M, Vecchiarelli HA, Robert MÈ, Tremblay MÈ (2021) Microglia fighting for neurological and mental health: on the central nervous system frontline of COVID-19 pandemic. Front Cell Neurosci 15 [Europe PMC free article] [Abstract]
- Graham EL, Clark JR, Orban ZS, Lim PH, Szymanski AL, Taylor C, DiBiase RM, Jia DT, Balabanov R, Ho SU, Batra A, Liotta EM, Koralnik IJ. Persistent neurologic symptoms and cognitive dysfunction in non-hospitalized Covid-19 "long haulers". Ann Clin Transl Neurol. 2021;8(5):1073–1085. 10.1002/acn3.51350. [Europe PMC free article] [Abstract] [CrossRef] [Google Scholar]
- Hanisch UK, Kettenmann H. Microglia: active sensor and versatile effector cells in the normal and pathologic brain. Nat Neurosci. 2007;10(11):1387–1394. 10.1038/nn1997. [Abstract] [CrossRef] [Google Scholar]
- Harry GJ, Kraft AD. Neuroinflammation and microglia: considerations and approaches for neurotoxicity assessment. Expert Opin Drug Metab Toxicol. 2008;4(10):1265–1277. 10.1517/17425255.4.10.1265. [Europe PMC free article] [Abstract] [CrossRef] [Google Scholar]
- Hoffmann M, Kleine-Weber H, Schroeder S, et al. SARS-COV2 cell entry depends on ACE2 and TMPRSS2 and is blocked by a clinically proven protease inhibitor. Cell. 2020;181:271–280. 10.1016/j.cell.2020.02.052. [Europe PMC free article] [Abstract] [CrossRef] [Google Scholar]
- Imre G. Cell death signalling in virus infection. Cell Signal. 2020;76:109772. 10.1016/j.cellsig.2020.109772. [Europe PMC free article] [Abstract] [CrossRef] [Google Scholar]
- Irrera N, Russo M, Pallio G, Bitto A, Mannino F, Minutoli L, Altavilla D, Squadrito F. The Role of NLRP3 Inflammasome in the Pathogenesis of Traumatic Brain Injury. Int J Mol Sci. 2020;21(17):6204. 10.3390/ijms21176204. [Europe PMC free article] [Abstract] [CrossRef] [Google Scholar]
- Jahani M, Dokaneheifard S, Mansouri K. Hypoxia: A key feature of COVID-19 launching activation of HIF-1 and cytokine storm. J Inflamm (lond) 2020;17:33. 10.1186/s12950-020-00263-3. [Europe PMC free article] [Abstract] [CrossRef] [Google Scholar]
- Kaminskyy V, Zhivotovsky B. To kill or be killed: how viruses interact with the cell death machinery. J Intern Med. 2010;267(5):473–482. 10.1111/j.1365-2796.2010.02222.x. [Abstract] [CrossRef] [Google Scholar]
- Kang S, Brown HM, Hwang S (2018) Direct antiviral mechanisms of interferongamma. Immune Netw. 18:e33. 10.4110/in.2018.18.e33 [Europe PMC free article] [Abstract]
- Lee S, Channappanavar R, Kanneganti TD (2020) Coronaviruses: Innate Immunity, Inflammasome Activation, Inflammatory Cell Death, and Cytokines. Trends Immunol 41(12):1083–1099 [Europe PMC free article] [Abstract]
- Li YC, Bai WZ, Hirano N et al (2013) Neurotropic virus tracing suggests a membranous-coating-mediated mechanism for transsynaptic communication. J Comp Neurol. 521(1):203–212. 10.1002/cne.23171 [Europe PMC free article] [Abstract]
- Liddelow SA, Guttenplan KA, Clarke LE et al (2017) Neurotoxic reactive astrocytes are induced by activated microglia. Nature 541(7638):481–487. 10.1038/nature21029 [Europe PMC free article] [Abstract]
- Liu J et al (2020) Longitudinal characteristics of lymphocyte responses and cytokine profiles in the peripheral blood of SARS-COV2 infected patients. EBioMedicine 55:102763 [Europe PMC free article] [Abstract]
- Livak KJ, Schmittgen TD (2001) Analysis of relative gene expression data using real-time quantitative PCR and the 2(-Delta Delta C(T)) Method. Methods 25(4):402–408 [Abstract]
- Marton O, Isabelle H, Dezso M, Agatha T, Martina P, Lejla G, Lowe CD, Tamas K. SARS-COV2 Causes a Different Cytokine Response Compared to Other Cytokine Storm-Causing Respiratory Viruses in Severely Ill Patients. Front Immunol. 2021;12:381. [Europe PMC free article] [Abstract] [Google Scholar]
- Merad M, Martin JC. Pathological inflammation in patients with COVID-19: a key role for monocytes and macrophages. Nat Rev Immunol. 2020;20:355–362. 10.1038/s41577-020-0331-4. [Europe PMC free article] [Abstract] [CrossRef] [Google Scholar]
- Nanduri J, Yuan G, Kumar GK. Transcriptional responses to intermittent hypoxia. Respir Physiol Neurobiol. 2008;2008(164):277–281. 10.1016/j.resp.2008.07.006. [Europe PMC free article] [Abstract] [CrossRef] [Google Scholar]
- Olbei M, Hautefort I, Modos D, Treveil A, Poletti M, Gul L, Shannon-Lowe CD, Korcsmaros T. SARS-CoV-2 Causes a Different Cytokine Response Compared to Other Cytokine Storm-Causing Respiratory Viruses in Severely Ill Patients. Front Immunol. 2021;12:629193. 10.3389/fimmu.2021.629193. [Europe PMC free article] [Abstract] [CrossRef] [Google Scholar]
- Oxley TJ, Mocco J, Majidi S, Kellner CP, Shoirah H, Singh IP, et al. Large-vessel stroke as a presenting feature of Covid-19 in the young. N Engl J Med. 2020;382:e60. 10.1056/NEJMc2009787. [Europe PMC free article] [Abstract] [CrossRef] [Google Scholar]
- Paniz-Mondolfi A, Bryce C, Grimes Z et al (2020) Central nervous system involvement by severe acute respiratory syndrome coronavirus–2 (SARS-COV2). J Med Virol. 10.1002/jmv.25915 [Europe PMC free article] [Abstract]
- Paterson RW, Brown RL, Benjamin L, Nortley R, Wiethoff S, Bharucha T, et al. The emerging spectrum of COVID-19 neurology: clinical, radiological and laboratory findings. Brain. 2020 10.1093/brain/awaa240. [Europe PMC free article] [Abstract] [CrossRef] [Google Scholar]
- Pero A, Ng S, Cai D. COVID-19: a perspective from clinical neurology and neuroscience. Neuroscientist. 2020;26:387–391. 10.1177/1073858420946749. [Abstract] [CrossRef] [Google Scholar]
- Poyiadji N, Shahin G, Noujaim D, Stone M, Patel S, Griffith B. COVID-19-associated acute hemorrhagic necrotizing encephalopathy: imaging features. Radiology. 2020;296:E119–E120. 10.1148/radiol.2020201187. [Europe PMC free article] [Abstract] [CrossRef] [Google Scholar]
- Puelles VG, Lutgehetmann M, Lindenmeyer MT, Sperhake JP, Wong MN, Allweiss L, Chilla S, Heinemann A, Wanner N, Liu S, et al. Multiorgan and renal tropism of SARS-COV2. N Engl J Med. 2020;383:590–592. 10.1056/NEJMc2011400. [Europe PMC free article] [Abstract] [CrossRef] [Google Scholar]
- Ratajczak MZ, Kucia M. SARS-COV2 infection and overactivation of Nlrp3 inflammasome as a trigger of cytokine “storm” and risk factor for damage of hematopoietic stem cells. Leukemia. 2020;34(7):1726–1729. 10.1038/s41375-020-0887-9. [Europe PMC free article] [Abstract] [CrossRef] [Google Scholar]
- Ren Y, et al. The ORF3a protein of SARS-COV2 induces apoptosis in cells. Cell Mol Immunol. 2020;17:881–883. 10.1038/s41423-020-0485-9. [Europe PMC free article] [Abstract] [CrossRef] [Google Scholar]
- Reynolds JL, Mahajan SD. SARS-COV2 Alters Blood Brain Barrier Integrity Contributing to Neuro-Inflammation. J Neuroimmune Pharmacol. 2021;16:4–6. 10.1007/s11481-020-09975-y. [Europe PMC free article] [Abstract] [CrossRef] [Google Scholar]
- Rodrigues TS, de Sá KSG, Ishimoto AY, Becerra A, Oliveira S, Almeida L, Gonçalves AV, Perucello DB, Andrade WA, Castro R, Veras FP, Toller-Kawahisa JE, Nascimento DC, de Lima MHF, Silva CMS, Caetite DB, Martins RB, Castro IA, Pontelli MC, de Barros FC, do Amaral NB, Giannini MC, Bonjorno LP, Lopes MIF, Santana RC, Vilar FC, Auxiliadora-Martins M, Luppino-Assad R, de Almeida SCL, de Oliveira FR, Batah SS, Siyuan L, Benatti MN, Cunha TM, Alves-Filho JC, Cunha FQ, Cunha LD, Frantz FG, Kohlsdorf T, Fabro AT, Arruda E, de Oliveira RDR, Louzada-Junior P, Zamboni DS. Inflammasomes are activated in response to SARS-COV2 infection and are associated with COVID-19 severity in patients. J Exp Med. 2021;218(3):e20201707. 10.1084/jem.20201707. [Europe PMC free article] [Abstract] [CrossRef] [Google Scholar]
- Rowaiye AB, Okpalefe OA, Onuh Adejoke O, Ogidigo JO, Hannah Oladipo O, Ogu AC, Oli AN, Olofinase S, Onyekwere O, Rabiu Abubakar A, Jahan D, Islam S, Dutta S, Haque M. Attenuating the Effects of Novel COVID-19 (SARS-COV2) Infection-Induced Cytokine Storm and the Implications. J Inflamm Res. 2021;14:1487–1510. 10.2147/JIR.S301784. [Europe PMC free article] [Abstract] [CrossRef] [Google Scholar]
- Ruan Q, Yang K, Wang W, Jiang L, Song J. Clinical predictors of mortality due to COVID-19 based on an analysis of data of 150 patients from Wuhan. China Intensive Care Med. 2020;46:846–848. 10.1007/s00134-020-05991-x. [Europe PMC free article] [Abstract] [CrossRef] [Google Scholar]
- Serebrovska ZO, Chong EY, Serebrovska TV, Tumanovska LV, Xi L. Hypoxia, HIF-1α, and COVID-19: from pathogenic factors to potential therapeutic targets. Acta Pharmacol Sin. 2020;41(12):1539–1546. 10.1038/s41401-020-00554-8. [Europe PMC free article] [Abstract] [CrossRef] [Google Scholar]
- Singh KK, Chaubey G, Chen JY, Suravajhala P. Decoding SARS-COV2 hijacking of host mitochondria in COVID-19 pathogenesis. Am J Physiol Cell Physiol. 2020;319:C258–C267. 10.1152/ajpcell.00224.2020. [Europe PMC free article] [Abstract] [CrossRef] [Google Scholar]
- Solomon IH, Normandin E, Bhattacharyya S, et al. Neuropathological features of COVID-19. N Engl J Med. 2020;2020(383):989–992. 10.1056/NEJMc2019373. [Europe PMC free article] [Abstract] [CrossRef] [Google Scholar]
- Steardo L, Jr, Steardo L, Verkhratsky A. Psychiatric face of COVID-19. Transl Psychiatry. 2020;10:261. 10.1038/s41398-020-00949-5. [Europe PMC free article] [Abstract] [CrossRef] [Google Scholar]
- Tan H, Wang N, Li S, Hong M, Wang X, Feng Y. The reactive oxygen species in macrophage polarization: reflecting its dual role in progression and treatment of human diseases. Oxidative Medicine and Cellular Longevity, Article ID. 2016 10.1155/2016/2795090. [Europe PMC free article] [Abstract] [CrossRef] [Google Scholar]
- van den Berg DF, Te Velde AA. Severe COVID-19: NLRP3 Inflammasome Dysregulated. Front Immunol. 2020;11:1580. 10.3389/fimmu.2020.01580. [Europe PMC free article] [Abstract] [CrossRef] [Google Scholar]
- Vassilaki N, Frakolaki E. Virus-host interactions under hypoxia. Microbes Infect. 2017;19:193–203. 10.1016/j.micinf.2016.10.004. [Abstract] [CrossRef] [Google Scholar]
- Wrapp D, Wang N, Corbett KS, Goldsmith JA, Hsieh CL, Abiona O, Graham BS, McLellan JS (2020) Cryo-EM structure of the 2019-nCoV spike in the prefusion conformation. Science 367(6483):1260-1263 [Europe PMC free article] [Abstract]
- Xu H, Chitre SA, Akinyemi IA, Loeb JC, Lednicky JA, McIntosh MT, Bhaduri-McIntosh S (2020) SARS-CoV-2 viroporin triggers the NLRP3 inflammatory pathway. BioRxiv. 10.27.357731. 10.1101/2020.10.27.357731 [Europe PMC free article] [Abstract]
- Zhao X, Eyo UB, Murugan M, Wu LJ. Microglial interactions with the neurovascular system in physiology and pathology. Dev Neurobiol. 2018;78:604–617. 10.1002/dneu.22576. [Europe PMC free article] [Abstract] [CrossRef] [Google Scholar]
- Zhou Z, Kang H, Li S, Zhao X. Understanding the neurotropic characteristics of SARS-COV2: from neurological manifestations of COVID-19 to potential neurotropic mechanisms. J Neurol. 2020;267:2179–2184. 10.1007/s00415-020-09929-7. [Europe PMC free article] [Abstract] [CrossRef] [Google Scholar]
- Zorov DB, Juhaszova M, Sollott SJ. Mitochondrial reactive oxygen species (ROS) and ROS-induced ROS release. Physiol Rev. 2014;94(3):909–950. 10.1152/physrev.00026.2013. [Europe PMC free article] [Abstract] [CrossRef] [Google Scholar]
Full text links
Read article at publisher's site: https://doi.org/10.1007/s11481-021-10015-6
Read article for free, from open access legal sources, via Unpaywall:
https://www.ncbi.nlm.nih.gov/pmc/articles/PMC8487226
Citations & impact
Impact metrics
Citations of article over time
Alternative metrics

Discover the attention surrounding your research
https://www.altmetric.com/details/114664153
Smart citations by scite.ai
Explore citation contexts and check if this article has been
supported or disputed.
https://scite.ai/reports/10.1007/s11481-021-10015-6
Article citations
Overlapping conditions in Long COVID at a multisite academic center.
Front Neurol, 15:1482917, 25 Oct 2024
Cited by: 0 articles | PMID: 39524912
Clinical rationale for dietary lutein supplementation in long COVID and mRNA vaccine injury syndromes.
F1000Res, 13:191, 17 Oct 2024
Cited by: 0 articles | PMID: 39526116
Review
SARS-CoV-2 Spike Protein 1 Causes Aggregation of α-Synuclein via Microglia-Induced Inflammation and Production of Mitochondrial ROS: Potential Therapeutic Applications of Metformin.
Biomedicines, 12(6):1223, 31 May 2024
Cited by: 0 articles | PMID: 38927430
IL-8 (CXCL8) Correlations with Psychoneuroimmunological Processes and Neuropsychiatric Conditions.
J Pers Med, 14(5):488, 03 May 2024
Cited by: 1 article | PMID: 38793070 | PMCID: PMC11122344
Review Free full text in Europe PMC
Intervention modalities for brain fog caused by long-COVID: systematic review of the literature.
Neurol Sci, 45(7):2951-2968, 02 May 2024
Cited by: 1 article | PMID: 38695969
Review
Go to all (37) article citations
Data
Data behind the article
This data has been text mined from the article, or deposited into data resources.
BioStudies: supplemental material and supporting data
Nucleotide Sequences
- (1 citation) ENA - QJE37812
Similar Articles
To arrive at the top five similar articles we use a word-weighted algorithm to compare words from the Title and Abstract of each citation.
Mitochondrial Dysfunction: A Prelude to Neuropathogenesis of SARS-CoV-2.
ACS Chem Neurosci, 13(3):308-312, 20 Jan 2022
Cited by: 18 articles | PMID: 35049274 | PMCID: PMC8790819
Direct endothelial ENaC activation mitigates vasculopathy induced by SARS-CoV2 spike protein.
Front Immunol, 14:1241448, 10 Aug 2023
Cited by: 5 articles | PMID: 37638055 | PMCID: PMC10449264
The amplification of CNS damage in Alzheimer's disease due to SARS-CoV2 infection.
Ann Diagn Pathol, 61:152057, 28 Oct 2022
Cited by: 11 articles | PMID: 36334414 | PMCID: PMC9616485
The Future of the COVID-19 Pandemic: How Good (or Bad) Can the SARS-CoV2 Spike Protein Get?
Cells, 11(5):855, 02 Mar 2022
Cited by: 15 articles | PMID: 35269476 | PMCID: PMC8909208
Review Free full text in Europe PMC
Funding
Funders who supported this work.
NIAID NIH HHS (2)
Grant ID: R01 AI129649
Grant ID: HHSN272201400008C
NIDA NIH HHS (1)
Grant ID: R01 DA047410