Abstract
Free full text

Structural basis for activation and gating of IP3 receptors
Abstract
A pivotal component of the calcium (Ca2+) signaling toolbox in cells is the inositol 1,4,5-triphosphate (IP3) receptor (IP3R), which mediates Ca2+ release from the endoplasmic reticulum (ER), controlling cytoplasmic and organellar Ca2+ concentrations. IP3Rs are co-activated by IP3 and Ca2+, inhibited by Ca2+ at high concentrations, and potentiated by ATP. However, the underlying molecular mechanisms are unclear. Here we report cryo-electron microscopy (cryo-EM) structures of human type-3 IP3R obtained from a single dataset in multiple gating conformations: IP3-ATP bound pre-active states with closed channels, IP3-ATP-Ca2+ bound active state with an open channel, and IP3-ATP-Ca2+ bound inactive state with a closed channel. The structures demonstrate how IP3-induced conformational changes prime the receptor for activation by Ca2+, how Ca2+ binding leads to channel opening, and how ATP modulates the activity, providing insights into the long-sought questions regarding the molecular mechanism underpinning receptor activation and gating.
Introduction
IP3Rs are intracellular Ca2+ channels, predominantly localized to the ER and activated by the binding of IP3 generated in response to external stimulation of G-protein coupled receptors1–3. Opening of the IP3Rs results in the rapid release of Ca2+ from the ER lumen into the cytoplasm, triggering diverse signaling cascades that regulate physiological processes such as learning, fertilization, gene expression, and apoptosis. Dysfunctional IP3Rs cause abnormal Ca2+ signaling and are associated with many diseases, including diabetes, cancer, and neurological disorders4,5. There are three IP3R subtypes (IP3R-1, -2, and -3) that share 60–70% sequence identity, form homo- or hetero-tetramers, exhibit different spatial expression profiles, and are involved in different signaling pathways1–3. Each IP3R subunit is about 2700 amino acids in length and contains a transmembrane domain (TMD) and a large cytoplasmic region comprising two β-trefold domains (βTF1 and βTF2), three Armadillo repeat domains (ARM1, ARM2, and ARM3), a central linker domain (CLD), a juxtamembrane domain (JD), and a short C-terminal domain (CTD)6–10 (Fig. 1).

a Domain boundaries of hIP3R-3. b–d Composite maps of hIP3R-3 in pre-active A (b), active (c), inactive (d) conformations. Each domain in one of the subunits is colored as in (a). Maps within the boxes, shown transparent, are close-up views of the Ca2+ binding site (red) and the pore (blue) with ribbon representation of hIP3R-3. Select residues are shown in the sticks. Dashed circles indicate opening through the gate.
In addition to IP3, the receptor activation requires Ca2+ at nanomolar concentrations, whereas Ca2+ at higher concentrations is inhibitory, causing the receptor to be tightly regulated by Ca2+,11–15. The cryo-EM structure of human IP3R-3 (hIP3R-3) in the presence of the inhibitory Ca2+ concentrations (2
mM) revealed two binding sites6. However, their role in channel activation and inhibition has remained uncertain. Furthermore, although ATP binding potentiates the receptor by increasing the open probability and duration of the channel openings, the underlying molecular mechanism has not been uncovered16,17. In this study, we illuminate the structural framework of receptor activation and channel opening by analyzing five cryo-EM structures of hIP3R-3 in the closed-pre-activated, open-activated, and closed-inactivated conformations.
Results and discussion
Cryo-EM structures of hIP3R-3 gating conformations
Bimodal regulation of IP3R activity by Ca2+ complicates sample preparation because of the requirement for fine adjustment of Ca2+ concentration to trap the channel in the open conformation. Although free Ca2+ concentrations in solutions can easily be controlled by using Ca2+ buffers such as EGTA or BAPTA, it becomes challenging during sample preparation for cryo-EM due to the small volumes used. Typically, a 2–3µl protein sample is applied to a cryo-grid, but more than 99% of the sample volume is lost during grid preparation due to extensive blotting with filter paper18. During this time, the samples contact filter paper and cryo-grids, containing various amounts of Ca2+. Small sample volumes and short time frames may reduce these buffers’ efficiency, causing the free Ca2+ concentration to increase to inhibitory levels prior to sample freezing. In order to maximize the chances of obtaining particles in the active state, we prepared the sample in: (1) EDTA, which has ~200 fold faster binding kinetics to Ca2+ than EGTA19, a common Ca2+ chelator, and is more likely to chelate excess Ca2+ and other divalent cations within the short period prior to the sample plunging, (2) ATP, which increases the open probability of IP3Rs and dampens the inhibitory effect of Ca2+,16,17, and (3) high concentrations of IP3.
The final hIP3R-3 sample was purified in the presence of 1mM EDTA and supplemented with 0.5
mM IP3, 5
mM ATP, and 0.1
mM CaCl2 before preparing cryo-grids. Although the free Ca2+ concentration was calculated around 100
nM under these conditions using Maxchelator20, the actual free Ca2+ concentration may be higher due to potential leakage of Ca2+ during the cryo-grid preparation as mentioned above. We performed a cryo-EM analysis on a large dataset by employing exhaustive 3D classification strategies to separate particles belonging to different functional states resulting in five high resolution (3.2–3.8
Å) structures (Supplementary Figs. 1–6; Supplementary Table 1). The pore region in all structures resolved to 3.5
Å or better, allowing us to build side chains and determine if the channel was open or closed (Fig. 1; Supplementary Figs. 2–6).
Three structures have closed pores with well-resolved densities for IP3 and ATP and are referred to as pre-active A, B, and C (Fig. 1b; Supplementary Figs. 1–7). The structure named “active” displays drastic conformational changes at the TMD, leading to pore opening (Fig. 1c). In addition to the well-resolved densities for IP3 and ATP, the active structure reveals substantial density, interpreted as Ca2+, at the ARM3-JD interface, referred to as the activatory Ca2+ binding site (Fig. 1c; Supplementary Figs. 5, 7). In the fifth structure, the channel is closed, the activatory Ca2+ binding site is occupied, and the intersubunit interactions of the cytoplasmic domains are lost (Fig. 1d; Supplementary Fig. 6). The structure is highly similar to the hIP3R-3 structures obtained in the presence of inhibitory Ca2+ concentrations6, except for βTF1, which moves closer to the ARM1 (Supplementary Fig. 8). Most notably, ARM2 adopted the same conformation relative to ARM1 and CLD, creating the binding site for the second Ca2+ observed at high Ca2+ concentrations (Supplementary Fig. 8). While these similarities suggest a Ca2+ ion occupies this site and the structure represents the Ca2+ inhibited state, the quality of the map around the region did not allow accurate inspection of the presence of Ca2+ (Supplementary Fig. 8). Therefore, we refer to the structure as “inactive” while it remains unclear if it represents a desensitized state that hIP3R-3 adopts without additional Ca2+ binding or an inhibited state forced by binding of additional Ca2+ to an inhibitory site.
It is important to note that our initial 3D classification runs resulted in two major classes grouping the pre-active and active structures into one class and the inactive structure into another (Supplementary Fig. 1). It was essential to perform another round of 3D classification focusing only on the core of the protein to separate the particles in the active state from the pre-active states, potentially due to subtle differences in the overall structures and the much fewer number of particles in the active state (20,039 particles compared to 346,684 particles in the pre-active states) (Supplementary Fig. 1; Supplementary Table 1).
Priming of hIP3R-3 for activation
To compare the structures presented here, we aligned their selectivity filters and pore helices (residues 2460-2481), which reside at the luminal side of the TMD and are virtually identical in all classes. The pre-active A structure is almost identical to the previously published IP3-bound hIP3R-3 structure6 (Supplementary Fig. 9a) and reveals that IP3 binding causes the ARM1 to rotate about 23° relative to the βTF-2, causing global conformational changes within the cytoplasmic domains, as observed in previous cryo-EM and X-ray crystallography experiments6,8,21–24 (Supplementary Fig. 9b, c). The pre-active B and C structures adopt distinct conformations that are intermediates between the pre-active A and open state structures. Based on these conformational changes, we propose a sequential transition from pre-active A to B, then C, although the alternative transitions cannot be ruled out entirely. During the transition to the pre-active B state, the N-terminal domain (NTD) of each protomer comprising βTF1, βTF2, ARM1, ARM2, and CLD rotates about 4° counter-clockwise relative to the TMD and moves about 2Å closer to the membrane plane (Fig. 2a; Supplementary Movie 1). In the pre-active C state, the NTDs remain primarily unchanged compared to the pre-active B state, while the ARM3 and JD are rotated by 7°, causing mild distortions at the cytoplasmic side of the TMD without opening the channel (Fig. 2b; Supplementary Movie 1). Compared to the ligand-free conformation, the βTFs move about 7
Å closer to the membrane plane, and ARM3-JD rotates about 11° in the pre-active C conformation.

a, b Ribbon representations of hIP3R structures superposed on the residues forming the selectivity filter and P-helix of the TMDs, emphasizing the conformational changes between the states indicated above. Domains with substantial conformational changes are shown in full colors only on one subunit, while the rest of the protein is transparent. Curved and straight red arrows indicate the rotation and translation of the domains with red labels relative to the rotation axis (black bars), respectively.
Ca2+-mediated conformational changes leading to pore opening
In the absence of Ca2+, the ARM3 and JD act as a rigid body, where there are no significant conformational changes relative to each other (Fig. 2a, b). When Ca2+ is bound, the JD rotates about 11° relative to the ARM3 (Fig. 3a), resembling a clamshell closure, which leads to global conformational changes in the whole receptor, including the movement of the NTD closer to the membrane plane by 2Å (Fig. 3b; Supplementary Movie 1). In contrast to the limited rotation of the ARM3 (about 5°), the JD rotates about 14° around an axis roughly perpendicular to the membrane plane, leading to conformational changes at the TMD and resulting in pore opening in the active state (Fig. 3b).

a Comparison of the JD (shown in full colors) orientation relative to the ARM3 (shown transparent) in the pre-active-C (orange) and active (blue) structures. The black bar indicates the axis for the rotation of the JD. Ca2+ and ATP are shown as spheres. b Global conformational changes induced by Ca2+ binding are depicted similar to Fig. 2. c Close-up view of the Ca2+ binding site in the active conformation. Domains are colored as in Fig. 1.
Ca2+ is coordinated by E1882 and E1946 on the ARM3 and the main-chain carboxyl group of T2581 on the JD (Fig. 3c). H1884 and Q1949 are also close and may interact with Ca2+ through water molecules (Fig. 3c). These residues are highly conserved in the homologous ion channel family, ryanodine receptors (RyRs), suggesting a common activation mechanism in IP3Rs and RyRs25 (Supplementary Fig. 10a, b). Mutation of the corresponding residues in RyRs markedly reduced the sensitivity to Ca2+, further supporting this site’s involvement in the Ca2+ induced activation26–28.
ATP binding site
Within the JD, we observed a well-resolved cryo-EM density for ATP in all the structures (Supplementary Fig. 7). The quality of the maps obtained through local refinement allowed unambiguous modeling of ATP, revealing its key interactions with the protein residues (Fig. 4a, b). The adenosine base intercalates into a cavity surrounded by F2156, F2539, I2559, M2565, and W2566 near the zinc finger motif and forms hydrogen bonds with the sulfur of C2538, the backbone amide group of F2539, and the carbonyl groups of H2563 and I2559 (Fig. 4b). The phosphate moieties interact with K2152, K2560, and N2564 (Fig. 4b). There are no apparent structural changes around the binding site upon ATP binding, suggesting that ATP’s potentiating effect is likely due to the increased rigidity of the JD (Supplementary Fig. 9d). ATP binding site is highly conserved among the subtypes except for E2149 which corresponds to lysine and arginine in IP3R-1 and IP3R-2 (Fig. 4c). A positively charged residue instead of E2149 in the proximity of the phosphate moieties may cause tighter interaction of ATP with IP3R-1 and IP3R-2, explaining the low binding affinity of ATP to IP3R-3 compared to IP3R-1 and IP3R-217.

a The cryo-EM density of ATP (red mesh) from the composite map of the pre-active A state and the modeled ATP molecule. b Close-up view of the ATP binding site in the pre-active A state. Dashed lines indicate hydrogen bonding. c Sequence alignment of hIP3R subtypes around the residues forming the ATP binding site. Residues shown in (b) are highlighted. Select residues are indicated by arrows, and E2149 is labeled in red.
ATP binds to a similar location near the zinc finger motif in RyRs25,29,30. However, its binding mode differs, potentially due to the differences in the residues that form the binding pocket, most notably the basic residues interacting with the phosphate moieties (Supplementary Fig. 10a, c, d). In RyR-1s, the phosphate moieties interact with K4211, K4214, and R4215, all located on a single helix (Supplementary Fig. 10c, d). In hIP3R-3, there is only a single lysine residue (K2152) on the corresponding helix, and the phosphate moieties interact with K2560, located on the opposite side of the binding pocket. A leucine residue (L4980) occupies this position in RyR-1s. The differences in the number and location of the basic residues likely force the phosphate moieties of ATP to adopt different conformations. Furthermore, F2156 in hIP3R-3 points toward the adenosine binding pocket, prohibiting ATP from adopting the conformations observed in RyR-1s due to steric clash in hIP3R-3s (Supplementary Fig. 10c, d).
Structure of the TMD in the open conformation
The TMD of IP3Rs has the same overall architecture of voltage-gated ions channels with a central pore domain, consisting of S5, S6, and pore (P) helix, surrounded by pseudo-voltage-sensor domains (pVSDs), consisting of S1, S2, S3, and S4 helices along with two IP3R/RyR specific TM helices (S1’ and S1”) (Fig. 5). In the closed channel, F2513 and I2517 of the S6 helix form two layers of hydrophobic constriction at the pore, blocking the path for the permeation of hydrated ions (Fig. 5). JD’s rotation upon Ca2+ binding pushes the pVSD’s cytoplasmic side away from the pore domain by about 7Å and tilts the cytoplasmic side of the S6 (S6cyt) by 12° (Fig. 5a; Supplementary Movie 1). Concurrently, the S4-5 linker and S5 helix move away from the S6 helix, thereby inducing a distortion of S6 around the constriction site and moving F2513 and I2517 away from the pore. As a result, the diameter of the water-accessible pore increases to 8
Å, large enough to permeate hydrated cations (Fig. 5b). The flexibility introduced by the neighboring glycine residue (G2514), mutation of which to alanine in IP3R-1 is associated with spinocerebellar ataxia 29 (SCA29)31, is likely critical to the movement of F2513. The tilting of the S6cyt breaks the salt bridge between D2518 and R2524 of the neighboring subunits, moving D2518 towards the pore while pulling R2524 away, which creates an electronegative path on the cytoplasmic side of the pore (Supplementary Fig. 11). In contrast to the prediction of a π- to α-helix transition at the S6lum during channel opening6,10, the π-helix remains intact, and its tip acts as a pivot for the S6cyt tilting and bulging (Fig. 5).
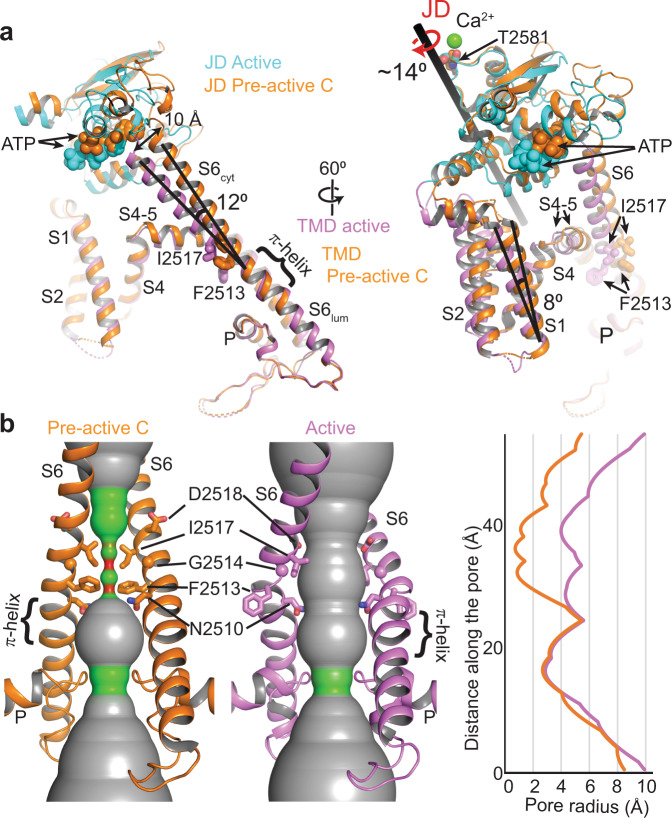
a Comparison of the hIP3R-3 structures in the pre-active C and active conformations aligned as in Fig. 2. b Ion permeation pathways of hIP3R-3 in pre-active C and active conformations (radii coloring: red, <0.8Å; green, 0.8-4.0
Å; gray, >4.0
Å) along with the 1D graph of the pore radius. The JD and TMD of the active state are shown in cyan and violet, respectively. The pre-active C structure is colored orange.
Although the TMDs of IP3Rs and RyRs are highly similar, there are noticeable differences in their pore structures (Supplementary Fig. 10e, f). In RyRs, the constriction site is formed by glutamine and isoleucine, corresponding to F2513 and I2517 in hIP3R-3, respectively32. In the open state of RyRs, the isoleucine is positioned similarly to I2517 of hIP3R-325,33,34. On the other hand, the glutamine residue faces the pore in the open state, forming part of the hydrophilic permeation pathway, unlike F2513. Interestingly, N2510 in hIP3R-3, which corresponds to alanine in RyRs, faces the permeation pathway similar to the glutamine of RyRs, suggesting that the amide group plays an important role in the ion permeation. However, since the side chain of N2510 extends from a different position on the S6 helix than the side chain of glutamine in RyRs, the binding pocket for ryanodine25, a RyR-specific inhibitor, is not present in IP3R, potentially causing IP3Rs to be unresponsive to ryanodine32.
Several missense mutations identified in the IP3R subtypes are associated with diseases, including spinocereblar ataxia, Gillespie syndrome, anhidrosis, and neck squamous cell carcinoma (Supplementary Fig. 12; reviewed in32,35,36). Perhaps not surprisingly, most of these mutations are localized around the IP3 binding site and alter IP3 binding affinity32,35–37. Another hot spot for these mutations is the constriction site of the pore, which undergoes conformational changes during channel opening (Supplementary Fig. 12). It is plausible that these mutations either affect the Ca2+ permeability (e.g., mutation of N251038 or I251739) or restrict conformational changes required for dilation of the pore (e.g., mutation of G251431). Two of the mutated residues (T251940 and F252041) interact with the residues on the S4-S5 linker, which couples the tilting of the pVSD to the bulging of the constriction site (Supplementary Fig. 12b). Mutations of these residues are likely to impair this coupling and thus hinder gating.
The flexibility of the CTD
The CTD, extending from the JD along the symmetry axis, forms a left-handed coiled-coil motif before interacting with the βTF2 of the neighboring subunit. The density for the CTD was poorly resolved in all of the states (Supplementary Fig. 13a, b). However, the coiled-coil motifs were visible in the unsharpened maps in the pre-active and active states, enabling us to model poly-alanine peptides without assigned registries (Supplementary Fig. 13a, b). The densities for the extensions from the coiled-coil motif towards the βTF2 become visible when viewed at lower thresholds, whereas the linkers between the JD and the coiled-coil motif remain invisible, indicating higher flexibility for this region (Supplementary Fig. 13a, b). We did not observe any interpretable density for the CTD in the inactive state (Supplementary Fig. 13a, b).
For IP3R-1, the CTD was proposed to transmit the conformational changes induced by IP3 at the NTD to the JD8. In IP3R-3, there are no apparent changes on the coiled-coil motif in the pre-active states, but the coiled-coil motif rotates about 20° around the symmetry axis and moves closer to the TMD by 6Å in the active state (Supplementary Fig. 13c, d). However, the linker between the coiled-coil motif and JD remains flexible, suggesting that the structural rearrangements of this domain are not directly enforcing the channel opening (Supplementary Fig. 13). In line with these observations, removing CTD residues interacting with the βTF2 or swapping the C-terminal region of IP3R-1 with the RyRs, which lack the extended CTD, did not diminish receptor activation21,24,42.
Mechanism of hIP3R-3 activation and gating
It has been long recognized that IP3 binding primes the receptor for activation by Ca2+,43, but how the priming is achieved has remained elusive. Our structures reveal that IP3 binding leads to several conformational changes at the NTD, ARM3, and JD, without any apparent structural changes at the activatory Ca2+ binding site, and that the ARM3 and JD adopt a new pre-gating conformation relative to the TMD with modest changes at the intersubunit interface between the JDs at the cytoplasmic side of the TMD (Fig. 6; Supplementary Fig. 14; Supplementary Movie 1). In addition, ARM3s are constrained in their pre-gating conformation by the tetrameric cage-like assembly of the NTDs, forcing the JDs to rotate upon Ca2+ binding. The NTD assembly is maintained by the βTF1-βTF2 intersubunit interactions (βTF ring), which remains intact in the pre-active and active states (Fig. 6; Supplementary Fig. 15; Supplementary Movie 1) and acts as a pivot for the conformational changes that stabilize the ARM3. On the other hand, its disruption in the inactive state leads to the loosening of the tetrameric assembly of the NTDs, relieving the ARM3 constraints and causing the JD and TMD to adopt the closed channel conformation despite the bound Ca2+ to the activatory site. Supporting this hypothesis, the removal of βTF1 or mutation of W168, which resides at the βTF1-βTF2 interface (Supplementary Fig. 15), was shown to abolish IP3R activity44,45.
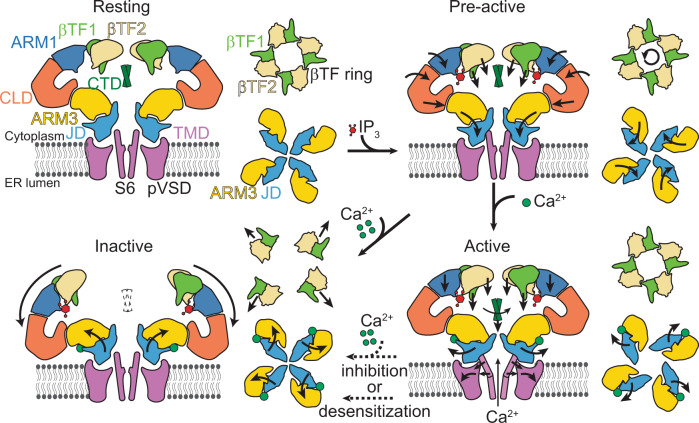
A side view of the two opposing subunits (left) and cytoplasmic views of the βTFs and ARM3-JD tetramers (right) for each indicated functional state is depicted. The ARM2 and CTD are omitted for clarity. Arrows shown on the domains indicate the direction of the rotation or translation from the previous conformation.
In conclusion, the ensemble of structures obtained from the same sample demonstrates structural heterogeneity of IP3Rs in the presence of IP3, ATP, and Ca2+. Our ability to correlate these structures with their plausible functional states allowed us to define the conformational changes at different gating states, revealing the structural features that underpin IP3R activation and gating. These structures will likely serve as foundations for future experiments addressing biophysical and functional questions related to IP3Rs. Furthermore, our study reinforces the power of cryo-EM in analyzing heterogeneous samples and highlights the importance of a thorough investigation of the data to identify physiologically relevant conformations, even when they constitute only a tiny fraction of the sample.
Methods
Protein expression and purification
Expression and purification of hIP3R-3 were performed as previously described with minor modifications10. Briefly, hIP3R-3 (residues 4-2671) with a C-terminal OneStrep tag was expressed using the MultiBac expression system46. Sf9 cells (4×
106 cells/mL) were harvested by centrifugation (4000
×
g) 48
h after infection with the baculovirus. Cells resuspended in a lysis buffer of 200
mM NaCl, 40
mM Tris-HCl pH 8.0, 2
mM EDTA pH 8.0, 10
mM β-mercaptoethanol (BME), and 1
mM Phenylmethylsulfonyl fluoride (PMSF) were lysed using Avastin EmulsiFlex-C3. After centrifugation of the lysate at 7000
×
g for 20
min to remove large debris, the membrane was pelleted by centrifugation at 185,000
×
g (Type Ti45 rotor) for 1
h. Membrane pellets were homogenized in ice-cold resuspension buffer (200
mM NaCl, 40
mM Tris-HCl pH 8.0, 2
mM EDTA pH 8.0, 10
mM BME) using a Dounce homogenizer, and solubilized using 0.5% Lauryl maltose neopentyl glycol (LMNG) and 0.1% glyco-diosgenin (GDN) at a membrane concentration of 100
mg/mL. After 4
h of gentle mixing in the cold room, the insoluble material was pelleted by centrifugation at 185,000
×
g (Type Ti45 rotor) for 1
h, and the supernatant was passed through Strep-XT resin (IBA Biotagnology) via gravity flow. The resin was washed first with 5 column volume (CV) of wash buffer composed of 200
mM NaCl, 20
mM Tris-HCl pH 8.0, 10
mM BME, 0.005% GDN, 0.005% LMNG, followed by 5 CV of wash buffer supplemented with 5
mM ATP and 20
mM MgCl2 to remove any bound chaperone proteins, and finally with 5CV of wash buffer supplemented with 1
mM EDTA. The protein was eluted using wash buffer supplemented with 1
mM EDTA and 100
mM D-Biotin (pH 8.2). The protein was further purified by size exclusion chromatography (SEC) using a Superose 6 Increase column (10/300 GL, GE Healthcare) equilibrated with the SEC buffer composed of 200
mM NaCl, 20
mM Tris-HCl pH 8.0, 1
mM EDTA pH 8.0, 2
mM TCEP, 0.005% LMNG, and 0.005% GDN. The fractions corresponding to hIP3R-3 were combined and concentrated to 4
mg/mL using a 100
kDa centrifugal filter (Sartorius). The concentrated sample was then centrifuged at 260,000
×
g using an S100AT rotor (ThermoFisher Scientific). The concentration dropped to 1.8
mg/mL.
Cryo-EM sample preparation and data collection
Purified hIP3R-3 in the SEC buffer containing 1mM EDTA was supplemented with 500
µM IP3 (from 10
mM stock in water), 0.1
mM CaCl2, and 5
mM ATP (from 100
mM stock, pH 7.2). 2.0
μL of the protein sample was applied to 300 mesh Cu Quantifoil 1.2/1.3 grids (Quantifoil Microtools) that were glow discharged for 20
s at 25
mA. The grids were blotted for 7
s at force 10 using single-layer Whatman ashless filter papers (Cat. #: 1442-055, GE Healthcare) and were plunged into liquid ethane using an FEI MarkIV Vitrobot at 8
°C and 100% humidity. The filter papers were not pre-treated with Ca2+ chelators or any other chemicals. Four grids prepared using the same sample were imaged using a 300
kV FEI Krios G3i microscope equipped with a Gatan K3 direct electron camera in four different data collection sessions at Case Western Reserve University. Movies containing 40–50 frames were collected at a magnification of ×105,000 in super-resolution mode with a physical pixel size of 0.828
Å/pixel and defocus values at a range of −0.8 to −1.6
µm using the automated imaging software SerialEM47 and EPU (ThermoFisher Scientific).
Cryo-EM data processing
Datasets from four sessions were initially processed separately using Relion 3.048. We used MotionCor249 and Gtcf50 to perform beam-induced motion correction and CTF estimations, respectively. We performed auto picking using the Laplacian-of-Gaussian option of Relion, extracted particles binned 4×
4, and performed 2D class classification. Using the class averages with apparent features, we performed another round of particle picking. We cleaned the particles, extracted as 4
×
4 binned, through 2D classification and performed 3D classification using the hIP3R-3 map (EMD-2084910), which was converted to the appropriate box and pixel size. We observed two predominant conformations. One had a compact NTD and tight interactions between subunits as in previously published IP3R structures in the absence of Ca2+, hereafter called “compact” conformation6–8,10 (Supplementary Fig. 1). The other one had the NTD of each subunit tilted away from the central symmetry axis resembling hIP3R-3 structures obtained in the presence of high Ca2+ concentrations, hereafter called “loose” conformation6 (Supplementary Fig. 1). These particles were separately selected and reextracted using a box size of 480
×
480 pixels at the physical pixel size. After 3D refinements, we performed CTF refinement and Bayesian polishing51.
We combined all the polished particles and performed another round of 3D classification, using one of the compact structures as a reference map. We grouped particles into “compact” and “loose” classes (Supplementary Fig. 1). Refinement of the particles in the “compact” conformation yielded a 3D reconstruction with an average resolution of 3.9Å. Although there were slight changes at the TMD compared to the structure in the closed state, these changes were not significant enough to suggest that the channel was open. To more clearly resolve the density around the TMD, we performed another round of 3D classification using a mask that only covers the ARM3, JD, and TMD and without performing an angular or translational alignment in Relion3 (Supplementary Fig. 1)52. 3D refinements of the particles in each class were performed using non-uniform refinement in CryoSPARC, enforcing C4 symmetry and local CTF refinements (Supplementary Fig. 1)53. Five classes led to four high-resolution (better than 4
Å) 3D reconstructions, whereas the 3D refinement of the other three classes resulted in poorly resolved maps. The particles in the “loose” conformation were processed using non-uniform refinement in CryoSPARC, but without enforcing any symmetry. Local resolution estimates were calculated using CryoSPARC53 (Supplementary Figs. 2–6). Some of the data processing and refinement software was supported by SBGrid54.
To improve the quality of the maps, we performed local refinements using masks covering parts of the original cryo-EM maps (Supplementary Figs. 2–6). We prepared five masks that cover distinct domains of one of the subunits for the pre-active A, B, C, and active conformations. After symmetry expansion using C4 symmetry, we performed local refinement using CryoSparc (Supplementary Figs. 2–5). For the inactive state, we prepared four masks that cover the cytoplasmic domains of each subunit and another mask that covers the tetrameric ARM3, JD, and TMD (Supplementary Fig. 6). The local refinements were performed using C1 symmetry for the cytoplasmic domains and C4 symmetry for the tetrameric ARM3, JD, and TMD. The resulting local refinement maps were aligned onto the original maps using Chimera55 and merged using the “VOP maximum” command of Chimera55 to prepare the composite maps (Supplementary Figs. 2–6).
Model building
Model building was performed using Coot56. We first placed the hIP3R-3 structure in ligand-free conformations (PDB ID: 6UQK10) into the composite map of Pre-active A, and performed rigid-body fitting of individual domains of one of the protomers. We then manually fit the residues into the density and expanded the protomer structure into a tetramer using the C4 symmetry. We performed real-space refinement using Phenix57. We repeated build-refine iterations till a satisfactory model was obtained. This model was used as a starting model for the other structures following the same workflow. Regions without interpretable densities were not built into the model. Residues without apparent density for their side chains were built without their side chains (i.e., as alanines) while maintaining their correct labeling for the amino acid type. The coiled-coil regions were modeled as poly-alanines without residue assignment using the unsharpened maps. Validations of the structural models were performed using MolProbity58 implemented in Phenix57.
Figure preparation
Figures were prepared using Chimera55, ChimeraX59, and The PyMOL Molecular Graphics System (Version 2.0, Schrödinger, LLC). Calculation of the pore radii was performed using the software HOLE60.
Reporting summary
Further information on research design is available in the Nature Research Reporting Summary linked to this article.
Supplementary information
Acknowledgements
We thank Dr. Kunpeng Lee for cryo-EM data collection at Case Western Reserve University. We thank Theo Humphries and other support staff at the Pacific Northwest Center for Cryo-EM (PNCC), Drs. Elad Binshtein, Melissa Chambers, and Scott Collier at the Cryo-EM facility at Vanderbilt University for their assistance with cryo-EM sample screening. We thank Drs. Hassane Mchaourab, Terunaga Nakagawa, and Silvia Ravera for discussions and review of the manuscript. This work was conducted in part using the CPU and GPU resources of the Advanced Computing Center for Research and Education (ACCRE) at Vanderbilt University. We used the DORS storage system supported by the U.S. National Institute of Health (NIH) (S10RR031634 to Jarrod Smith). This work was supported by the NIH (R01GM141251 to E.K.), Vanderbilt University, Vanderbilt Diabetes and Research Training Center (NIH P30DK020593 to E.K.), and the Molecular Biophysics Training Program (NIH T32GM008320 to E.A.S.).
Author contributions
E.K. conceived the project and performed cryo-EM data analysis; E.A.S. optimized and performed protein expression and purification; H.T. performed grid preparation for cryo-EM. All authors contributed to the preparation of the manuscript.
Peer review
Peer review information
Nature Communications thanks Amedee des Georges and the other, anonymous, reviewer(s) for their contribution to the peer review of this work. Peer reviewer reports are available.
Data availability
The data that support this study are available from the corresponding author upon reasonable request. Cryo-EM maps and atomic coordinates are deposited to the Electron Microscopy Data Bank (EMDB) and Protein Data Bank (PDB) databases, respectively. The accession codes are EMD-25667 and 7T3P for pre-active A, EMD-25668 and 7T3Q for pre-active B, EMD-25669 and 7T3R for pre-active C, EMD-25670 and 7T3T for active, and EMD-25671 and 7T3U for inactive states, respectively. The following previously published datasets were used: EMD-20849, Cryo-EM structure of type 3 IP3 receptor revealing presence of a self-binding peptide10. 6UQK, Cryo-EM structure of type 3 IP3 receptor revealing presence of a self-binding peptide10. 6DRC, High IP3 Ca2+ human type 3 1,4,5-inositol trisphosphate receptor6. 6DQV Class 2 IP3-bound human type 3 1,4,5-inositol trisphosphate receptor6. 5TAL, Structure of rabbit RyR1 (Caffeine/ATP/Ca2+ dataset, class 1 and 2)25. 7M6A, High resolution structure of the membrane embedded skeletal muscle ryanodine receptor29. Reagents and other materials will be available upon request from E.K. with a completed materials transfer agreement.
Footnotes
Publisher’s note Springer Nature remains neutral with regard to jurisdictional claims in published maps and institutional affiliations.
These authors contributed equally: Emily A. Schmitz, Hirohide Takahashi.
Supplementary information
The online version contains supplementary material available at 10.1038/s41467-022-29073-2.
References
Articles from Nature Communications are provided here courtesy of Nature Publishing Group
Full text links
Read article at publisher's site: https://doi.org/10.1038/s41467-022-29073-2
Read article for free, from open access legal sources, via Unpaywall:
https://www.nature.com/articles/s41467-022-29073-2.pdf
Citations & impact
Impact metrics
Citations of article over time
Alternative metrics

Discover the attention surrounding your research
https://www.altmetric.com/details/124799400
Smart citations by scite.ai
Explore citation contexts and check if this article has been
supported or disputed.
https://scite.ai/reports/10.1038/s41467-022-29073-2
Article citations
Dissecting the neuroprotective interaction between the BH4 domain of BCL-w and the IP3 receptor.
Cell Chem Biol, 31(10):1815-1826.e5, 26 Jul 2024
Cited by: 0 articles | PMID: 39067448
ITPR1 variant-induced autosomal dominant hereditary spastic paraplegia in a Chinese family.
Front Neurol, 15:1365787, 01 Jul 2024
Cited by: 0 articles | PMID: 39011359 | PMCID: PMC11247953
The ion channels of endomembranes.
Physiol Rev, 104(3):1335-1385, 07 Mar 2024
Cited by: 1 article | PMID: 38451235 | PMCID: PMC11381013
Review Free full text in Europe PMC
C-X-C motif chemokine ligand 12-C-X-C chemokine receptor type 4 signaling axis in cancer and the development of chemotherapeutic molecules.
Tzu Chi Med J, 36(3):231-239, 27 May 2024
Cited by: 0 articles | PMID: 38993827
Review
Nobiletin regulates intracellular Ca2+ levels via IP3R and ameliorates neuroinflammation in Aβ42-induced astrocytes.
Redox Biol, 73:103197, 16 May 2024
Cited by: 0 articles | PMID: 38781730 | PMCID: PMC11145555
Go to all (24) article citations
Data
Data behind the article
This data has been text mined from the article, or deposited into data resources.
BioStudies: supplemental material and supporting data
Electron Microscopy Data Bank
- (1 citation) Electron Microscopy Data Bank - EMD-2084
Protein structures in PDBe
-
(1 citation)
PDBe - 6UQKView structure
Electron Microscopy Data Bank (EMDB) at PDBe (5)
Similar Articles
To arrive at the top five similar articles we use a word-weighted algorithm to compare words from the Title and Abstract of each citation.
IP3 receptors - lessons from analyses ex cellula.
J Cell Sci, 132(4):jcs222463, 14 Dec 2018
Cited by: 13 articles | PMID: 30552138
Review
Structural basis for the regulation of inositol trisphosphate receptors by Ca2+ and IP3.
Nat Struct Mol Biol, 25(8):660-668, 16 Jul 2018
Cited by: 63 articles | PMID: 30013099 | PMCID: PMC6082148
Conformational motions and ligand-binding underlying gating and regulation in IP3R channel.
Nat Commun, 13(1):6942, 14 Nov 2022
Cited by: 15 articles | PMID: 36376291 | PMCID: PMC9663519
Structural titration reveals Ca2+-dependent conformational landscape of the IP3 receptor.
Nat Commun, 14(1):6897, 28 Oct 2023
Cited by: 5 articles | PMID: 37898605 | PMCID: PMC10613215
Funding
Funders who supported this work.
NCRR NIH HHS (1)
Grant ID: S10 RR031634
NIDDK NIH HHS (1)
Grant ID: P30 DK020593
NIGMS NIH HHS (2)
Grant ID: T32 GM008320
Grant ID: R01 GM141251
U.S. Department of Health & Human Services | NIH | National Institute of General Medical Sciences (1)
Grant ID: R01GM141251