Abstract
Free full text

Viral load is associated with mitochondrial dysfunction and altered monocyte phenotype in acute severe SARS-CoV-2 infection
Abstract
Monocytes play a major role in the initial innate immune response to SARS-CoV-2. Although viral load may correlate with several clinical outcomes in COVID-19, much less is known regarding their impact on innate immune phenotype. We evaluated the monocyte phenotype and mitochondrial function in severe COVID-19 patients (n = 22) with different viral burden (determined by the median of viral load of the patients) at hospital admission. Severe COVID-19 patients presented lower frequency of CD14 + CD16- classical monocytes and CD39 expression on CD14 + monocytes, and higher frequency of CD14 + CD16 + intermediate and CD14-CD16 + nonclassical monocytes as compared to healthy controls independently of viral load. COVID-19 patients with high viral load exhibited increased GM-CSF, PGE-2 and lower IFN-α as compared to severe COVID-19 patients with low viral load (p < 0.05). CD14 + monocytes of COVID-19 patients with high viral load presented higher expression of PD-1 but lower HLA-DR on the cell surface than severe COVID-19 patients with low viral load. All COVID-19 patients presented decreased monocyte mitochondria membrane polarization, but high SARS-CoV-2 viral load was associated with increased mitochondrial reactive oxygen species. In this sense, higher viral load induces mitochondrial reactive oxygen species generation associated with exhaustion profile in CD14 + monocytes of severe COVID-19 patients. Altogether, these data shed light on new pathological mechanisms involving SARS-CoV-2 viral load on monocyte activation and mitochondrial function, which were associated with COVID-19 severity.
1. Introduction
The coronavirus disease 2019 (COVID-19) pandemic caused by infection with severe acute respiratory syndrome coronavirus-2 (SARS-CoV-2) has been rapidly spreading worldwide and afflicted millions following first reports at the end of 2019 [1]. Disease mortality (2% of the cases) is primarily caused by severe pneumonia and acute respiratory distress syndrome, whereas a significant proportion of patients is asymptomatic (20%) or develop mild COVID-19 symptomatic condition. Several risk factors, including age, sex, body mass index, and the presence of chronic diseases, are associated with COVID-19 severity and mortality [2]. More recently, some studies demonstrated that the high SARS-CoV-2 viral load was an independent predictor of mortality in large hospital cohorts [2], [3], [4]. Moreover, a higher prevalence of detectable SARS-CoV-2 plasma viral load is associated with worse respiratory disease severity, lower lymphocyte counts, and hypercytokinemia [4].
SARS-CoV-2 possesses the ability to evade the innate immune defenses and to promote dysfunction and exacerbated inflammatory responses that are linked to the severity of acute infection. Increased viral load was previously [5] linked to hypercytokinemia, endothelial dysfunction, and coagulation activation, suggesting that RNA viremia may directly impact the innate immune response during the first days of infection. Although alterations in the myeloid cell phenotype and function was previously associated with severe COVID-19 [6], [7], the association with viral load remains poor studied.
Monocytes play a key role in the initial immune response against several viruses and acquire an intermediate or nonclassical CD16 + phenotype during SARS-CoV-2 infection, and this response against virus request an adequate available energy source, principally in the mitochondrial function, to leads an adequate antiviral response without exacerbation of inflammation [8]. Once activated by sensing some pathogen-associated molecular pattern, monocytes activate the aerobic glycolysis axis through the Akt-mTOR-HIF-1α pathway to increase the energetic demand during the infection [9]. Similarly, SARS-CoV-2 infection favors the HIF-1α/Glycolysis-Dependent Axis [10] associated with mitochondrial dysfunction [11] in monocytes that may be linked to the hyperinflammatory condition observed in severe COVID-19. On the other hand, monocytes from severe COVID-19 patients are phenotypically characterized by the downregulation of human leukocyte antigen DR isotype (HLA-DR) concomitant with the increased expression of chemokine receptors and pathogen recognition receptors [12]. Interestingly, a relationship between leukocyte mitochondrial oxygen consumption and HLA-DR expression was previously described in septic shock patients [13], suggesting a key link between metabolic disturbance and changes in the activation patterns during acute infection or sepsis conditions. Moreover, we recently showed that blood factors, such as inflammatory cytokines and microbial products, found in the plasma of severe COVID-19 modulate monocyte phenotype to a dysfunctional hyperactivated profile [14]. Here, our study aimed to evaluate the role of viral load on innate cytokines and monocyte phenotype in severe COVID-19 patients.
2. Methods
2.1. Participants and sampling procedures
We prospectively evaluated patients diagnosed with severe COVID-19 acute infection that were admitted to Hospital Moinhos de Vento and a sample of uninfected healthy individuals. The diagnosis of COVID-19 was confirmed by SARS-CoV-2 nucleic acid testing of nasal and pharyngeal throat swab specimens using real-time RT-PCR assay and the severity of COVID-19 was classified according to the WHO interim guidance [15]. This study was approved by the Moinhos de Vento Ethics Committee N 3,977,144 (Porto Alegre/Brazil) and informed consent was obtained from all participants.
Blood samples (5 mL) were obtained from controls and severe COVID-19 patients into K2EDTA tubes (Becton & Dickinson, USA) within 6 h from hospital admission.
Concomitantly, nasopharyngeal samples were obtained from the participants according to the CDC recommendations [16] using FLOQSwab® with eNAT medium (COPAN Diagnostics Inc.).
Flow cytometry experiments (described below) were conducted with fresh blood. The remaining blood was centrifuged (1500 g, 10 min), plasma was aliquoted and kept at −80 °C until plasma analysis.
2.2. Viral load determination
Nucleic acid extraction was carried out using 300 µL of each nasopharyngeal sample with the Maxwell® RSC Viral Total Nucleic Acid Purification Kit according to the manufacturer's instructions. Total nucleic acids were eluted in 50 µL of water.
SARS-CoV-2 viral load of the samples was determined using a real-time quantitative RT-PCR (RT-qPCR) assay for absolute quantification of the viral RdRp RNA previously developed by our group [17]. All samples were run in duplicates. Serial 10-fold dilutions of known quantities of contrived RdRp transcripts (102 to 109 molecules per reaction) were utilized by plate, as reference, for establishing the standard curve.
2.3. Cytokine and prostaglandin E2 analyses
The plasma concentrations of granulocyte–macrophage colony-stimulating factor (GM-CSF), interferon-alpha (IFN-α), prostaglandin E2 (PGE-2), tumor necrosis factor-alpha (TNF-α) (all from Invitrogen, ThermoFisher, USA) were quantified by enzyme‐linked immunosorbent assay (ELISA) in microplate reader (EzReader, EUA). The detection limits of each cytokine were GM-CSF, 6–750 pg/mL, IFN-α, 2–300 pg/mL, TNF-α, 2‐400 pg/mL; PGE-2, 39.1–10.000 pg/mL.
2.4. Systemic redox state analysis
Lipid peroxidation was measured through the thiobarbituric acid reactive substances (TBARS) in plasma following a previously described protocol [18]. Reactive oxygen species (ROS) production was evaluated in the plasma through the fluorescence intensity of the redox-sensitive dye 2′,7′-dichlorodihydrofluorescein diacetate (DCFH, 100 μM, Sigma-Aldrich, St. Louis, MO, USA) (excitation and emission wavelengths of 480 and 535 nm, respectively) using SpectraMax M2e (Molecular Devices, St. Louis, MO, USA). The total antioxidant capacity (TAC) of plasma using 2,2′-azino-bis (3-ethylbenzothiazoline-6-sulfonic acid) diammonium salt (ABTS) (Sigma-Aldrich) incubated with potassium persulphate (Sigma- Aldrich) to produce ABTS+, a green/blue chromophore. The inhibition of ABTS
+ formation by antioxidants in the samples was expressed as Trolox equivalents, determined at 740 mm using a calibration curve plotted with different amounts of Trolox (Sigma-Aldrich). Nitrite levels were determined by Griess method as previously described [19].
2.5. Immunophenotyping
Whole blood samples (100 µL) were incubated with monoclonal surface antibodies (all anti-human) at 4 °C for 20 min in accordance with the following combination: a) FITC-conjugated anti-CD14, Pe-conjugated anti-CD16; b) FITC-conjugated anti-CD4, Pe-conjugated anti-CD8; c) FITC-conjugated anti-CD14, Pe-conjugated anti-CD39; d) FITC-conjugated anti-CD14, APC-conjugated anti-CD73; e) FITC-conjugated anti-CD14, PerCP-Cy5.5-conjugated anti-HLA-DR; f) FITC-conjugated anti-CD14, APC-conjugated anti-PD-1. Then, samples were incubated for 10 min with lysing buffer (BD Biosciences) and then centrifuged at 500g for 5 min. The supernatant was discarded, and samples were resuspended in phosphate buffered saline (PBS 1 mL) and then centrifuged at 500g for 5 min. Finally, the samples were resuspended in 0.5 mL PBS and analyzed in flow cytometry. Cell phenotype was acquired using CELLQuest Pro Software (BD Bioscience) on a FACSCalibur flow cytometer (BD Bioscience).
2.6. Mitochondrial membrane polarization and mitochondrial ROS
The mitochondrial membrane potential (ΔΨm) was quantified according to a method previously described [20], using the fluorescent dye rhodamine 123 (Rh 123, Sigma-Aldrich). Mitochondrial superoxide generation in live cells was assessed with MitoSOX Red (Invitrogen, ThermoFisher, USA). Analyses were performed by using CELLQuest Pro Software (BD Bioscience) on a FACSCalibur flow cytometer (BD Bioscience).
2.7. Statistical analysis
Normality of data was checked by Kolmogorov‐Smirnov, and the values were presented as mean ± standard deviation (SD). A one‐way ANOVA followed by Bonferroni's post‐hoc for multiple comparisons was used to verify between-groups differences. Correlations analyses were performed by Pearson’s Coefficient Correlation. P values ≤ 0.05 were considered statistically significant. The SPSS 20.0 (IBM Inc, EUA) software was used in all analysis.
3. Results
3.1. Participant’s characteristics
The characteristics of severe COVID-19 patients and controls were presented in Table 1 . COVID-19 patients were assigned to low or high viral load in accordance with the median of SARS-CoV-2 log copies/mL. The mean viral load of severe COVID-19 patients was 3.57 ± 1.57 log copies/mL, and high viral load group presented superior SARS-CoV-2 copies than low viral load group as expected (4.8 ± 0.9 vs. 2.2 ± 0.8 log copies/mL, p = 0.001). Severe COVID-19 patients with high viral load presented low time interval between onset of symptoms and blood collection (7.8 ± 3.4 vs. 11.9 ± 3.2 days, p = 0.01). The low and high COVID-19 viral load groups also differed in the number of days from symptoms onset and blood sample collection (p = 0.01), heart rate (p = 0.02), the prevalence of sore throat symptom (p = 0.02), and type 2 diabetes mellitus (p = 0.02).
Table 1
Demographic and clinical participant’s characteristics.
Healthy controls (n = 9) | Severe COVID19 patients | p | ||
---|---|---|---|---|
Low Viral Load(n = 11) | High Viral Load (n = 11) | |||
Viral Load (log copies/mL) | 2.2 ± 0.8 | 4.8 ± 0.9 | 0.001 | |
Age (y) | 52.3 ± 18.5 | 56.4 ± 12.1 | 63.6 ± 21.1 | 0.22 |
Sex (male) | 3 (23.1) | 7 (63.6) | 6 (54.5) | 0.66 |
Racial or ethnic group
| 8 (72.7)3 (27.3) | 9 (81.8)2 (18.2) | 0.61 | |
Height (m) | 1.68 ± 0.07 | 1.67 ± 0.12 | 0.33 | |
Body mass (kg) | 82.1 ± 11.7 | 82.1 ± 17.7 | 0.83 | |
BMI (kg/m2) | 29.0 ± 4.2 | 28.3 ± 3.9 | 0.99 | |
BMI
| 3 (27.3)2 (18.2)6 (54.5) | 2 (20)6 (60)2 (20) | 0.12 | |
Days between symptoms onset and blood collection | 11.9 ± 3.2 | 7.8 ± 3.4 | 0.01 | |
SpO2 at hospital admission | 93.0 ± 5.0 | 94.3 ± 3.3 | 0.52 | |
Oxygen use during hospitalization | 6 (54.5) | 8 (72.7) | 0.12 | |
Respiratory rate | 19.1 ± 1.5 | 21.7 ± 4.3 | 0.09 | |
SBP (mmHg) | 135.1 ± 16.6 | 124.9 ± 12.6 | 0.13 | |
DBP (mmHg) | 77.0 ± 11.5 | 77.0 ± 12.2 | 1.0 | |
HR (bpm) | 89.8 ± 15.4 | 105.3 ± 14.2 | 0.02 | |
Underlying medical condition
| 4 (36.4)00001 (9.1)1 (9.1) | 5 (45.5)5 (45.5)2 (18.2)1 (9.1)2 (18.2)1 (9.1)1 (9.1) | 0.140.020.150.140.111.01.0 |
Quantitative data presented as mean ± standard deviation. Between groups comparison through one way ANOVA (p < 0.05).
BMI, body mass index; COPD, chronic obstructive pulmonary disease; HIV, human immunodeficiency virus; SBP, systolic blood pressure; DBP, diastolic blood pressure; HR, heart rate.
Qualitative data presented as relative frequency. Between groups comparison through Qui-square test (p < 0.05).
3.2. Effects of SARS-CoV-2 viral load on inflammatory mediators, oxidative status and frequency of T lymphocytes and monocytes subsets in severe COVID-19 patients
Severe COVID-19 patients presented increased proportions of CD14 + CD16 + intermediate (p < 0.05) and CD14-CD16 + nonclassical monocytes (p < 0.01), and lower frequencies of CD14 + CD16- monocytes (p < 0.001), as well as CD4 + and CD8 + T cells (p < 0.01 for both) in the peripheral blood as compared to healthy controls. Furthermore, severe COVID-19 patients presented higher systemic levels of GM-CSF (p < 0.001), TNF-α (p < 0.001), PGE-2 (p < 0.001), but diminished IFN-α levels (p < 0.001) as compared to uninfected controls. We also analyzed some markers of systemic redox state and found higher ROS generation (p < 0.001) and lipid peroxidation (TBARS analysis, p < 0.001) in severe SARS-CoV-2-infected patients, despite similar TAC activity (p > 0.05). In addition, lower nitrite levels were identified in severe COVID-19 patients (p < 0.01). When the patients were assigned in groups in accordance with their viral load, severe COVID-19 patients with high SARS-CoV-2 viral load presented higher GM-CSF (p < 0.001), PGE-2 (p < 0.01) plasma concentrations and ROS generation (p < 0.001), and lower IFN-α levels (p < 0.05) than those with low SARS-CoV-2 viral load (Fig. 1 ).
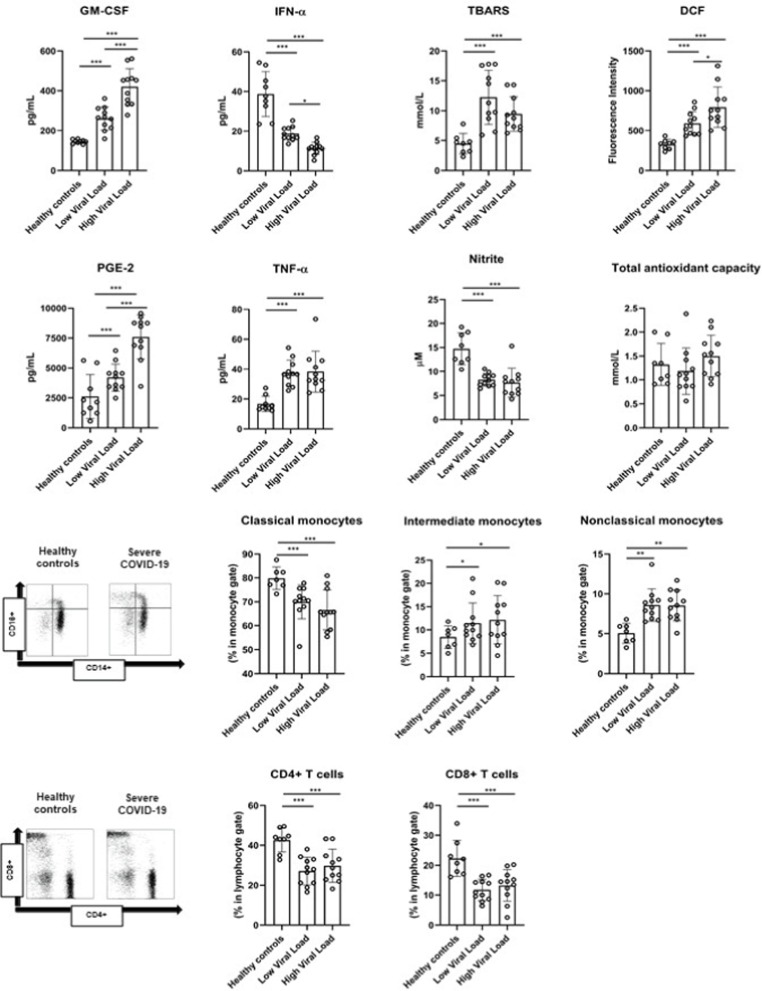
Systemic levels of inflammatory mediators, redox state markers, T cell frequency and monocyte subset distribution in severe COVID-19 patients according to viral load.
GM-CSF, granulocyte–macrophage colony-stimulating factor; TNF-α, tumor necrosis factor-alpha; PGE-2, prostaglandin-E2; IFN-α, interferon-alpha; DCF, dichlorofluorescin diacetate; TBARS, thiobarbituric acid reactive substances.
Statistical analysis was conducted through one-way ANOVA followed by Bonferroni’s post-hoc (p < 0.05).
3.3. The expression of costimulatory and coinhibitory immunocheckpoints are deregulated in COVID-19
The expression of CD39, CD73, PD-1 and HLA-DR were analyzed on the cell surface of CD14 + monocytes. Monocytes of healthy controls presented higher CD39 expression (p = 0.008) and HLA-DR (p = 0.001) and lower PD-1 (p = 0.001) as compared to severe COVID-19 patients. Furthermore, monocytes from severe COVID-19 patients presented lower expression of Rh 123 (p < 0.001), indicating a state of depolarization of the mitochondrial membrane, and higher anion superoxide production (p < 0.001), a mitochondrial reactive oxygen species as compared to controls. High SARS-CoV-2 viral load was associated with increased PD-1 expression in CD14 + monocytes (p = 0.01) and mitochondrial ROS generation by monocytes (p = 0.05), but lower HLA-DR expression in CD14 + monocytes as compared to patients with low SARS-CoV-2 viral load (Fig. 2 ).
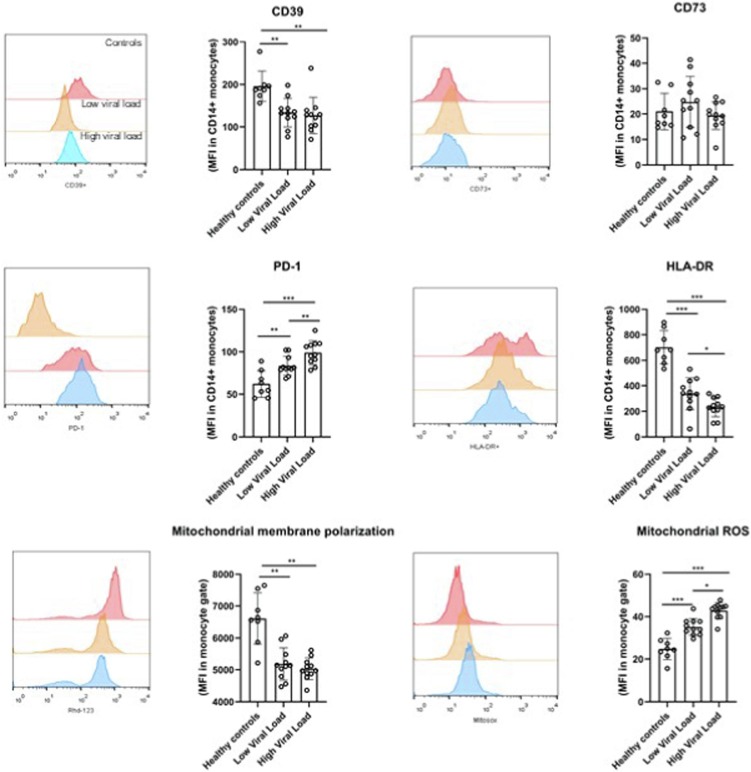
Unbalance in costimulatory and coinhibitory molecules and mitochondrial redox markers in CD14 + monocytes in severe COVID-19 patients according to viral load.
The expression of CD39, CD73, PD-1 and HLA-DR were analyzed on the cell surface of CD14 + monocytes of severe COVID-19 patients and healthy controls. Mitochondrial membrane polarization and mitochondrial reactive oxygen species generation in monocytes. Statistical analysis was conducted through one-way ANOVA followed by Bonferroni’s post-hoc (p < 0.05).
3.4. The relationship between clinical measurements and immune variables
The variable “days of symptoms onset” was significantly positively correlated to peripheral CD14 + CD16- monocytes frequency (r = 0.61, p = 0.02), CD73 + expression in CD14 + monocytes (p = 0.58; p = 0.04) and TBARS (r = 0.42; p = 0.04), and inversely related to mitochondrial ROS (r = -0.43; p = 0.03). Systolic blood pressure related to HLA-DR expression in CD14 + monocytes (r = 0.58; p = 0.01) and inversely related to nitrite levels (r = -0.44; p = 0.05). Heart rate inversely related to CD14 + CD16- classical monocytes (r = -0.59; p = 0.02) and IFN-α (r = -0.48; p = 0.03), but positively related to GM-CSF (r = 0.56; p = 0.04) and PGE2 (r = 0.54; p = 0.05). Finally, SARS-CoV-2 viral load positively related to PD-1 expression in CD14 + monocytes (r = 0.50; p = 0.02), mitochondrial ROS production by monocytes (r = 0.64; p = 0.03) and systemic ROS levels (r = 0.42; p = 0.03), but inversely related to HLA-DR expression in CD14 + monocytes (r = -0.49; p = 0.02). Body mass positively related to TAC (r = 0.49; p = 0.03), and age related to ROS production (r = 0.36; p = 0.05) but inversely related to nitrite levels (r = -0.35; p = 0.02) and IFN-α (r = -0.04; p = 0.03) (Fig. 3 ).
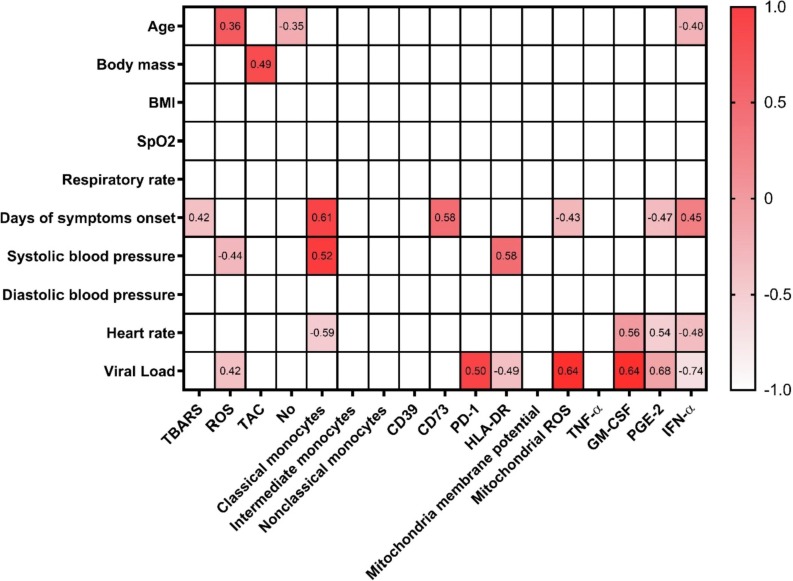
The Correlation heat map including clinical variables and monocyte immune variables at admission to the Hospital. Pearson’s correlation coefficients are plotted. Cells were colored according to the strength and trend of correlations. Statistical analysis performed through Pearson’s coefficient correlation.
4. Discussion
Here, we report that monocytes from patients with severe acute COVID-19 infection with different degrees of SARS-CoV-2 viral load with important alterations on immunophenotype, cytokines profile and mitochondrial function. Our main finding revealed that the viral load in severe COVID-19 patients directly impacted the systemic ROS generation, and the production of GM-CSF, PGE-2 and IFN-α. Furthermore, severe COVID-19 patients with high viral load presented higher expression of PD-1, but lower HLA-DR in CD14 + monocytes, and increased mitochondrial ROS generation by monocytes as compared to those with low viral load. Collectively, our data revealed changes in systemic innate cytokines and redox state, as well as in the phenotype and function of monocytes linked to the viral load at time of hospital admission that may contribute to the pathological state of hyperinflammation in COVID-19.
Interestingly, high viral load leads to higher PGE-2 and GM-CSF concomitant with lower circulating IFN-α levels which corroborate our previous reports demonstrating an association between inflammatory cytokines and SARS-CoV-2 viral load, detected by N1 protein, in severe COVID-19 patients [21], [22]. Furthermore, SARS-CoV-2 can shut-off the host antiviral cytokines, including IFN-α gene expression, by inhibiting IRF9 and STAT1 phosphorylation [23]. In addition, Ricke-Hok and colleagues [24] demonstrated that SARS-CoV-2 itself upregulates PGE2 in infected host cells, impairing B-cell mediated immune response by reducing PAX5, and higher PGE2 blood levels are a hallmark of immune deregulation in severe COVID-19. PGE2 can exert immunosuppressive effects during viral infection, and its elevation inhibits IFN-α secretion and Th1 costimulation by myeloid cells [25]. Thus, it is possible that high viral load may be a determinant factor to inhibit type 1 IFN secretion and innate antiviral cytokine response during the initial phase of the disease through the augment of PGE2 and others bioactive lipid mediators in COVID-19. On the other hand, it is interesting to note that viral load did not impact on nitrite levels, suggesting that SARS-CoV-2 infection by itself, but not the number of viral copies, impairs the bioavailability of protective nitric oxide (NO).
Host immune responses to SARS-CoV-2 are directly dependent of viral load and infection course [26]. Interestingly, the days from symptom onset to sample collection in our cohort were lower in higher viral load group suggesting that symptomatic phase may occur early in these patients. However, viral load was not determinant to change the peripheral frequency of monocyte subsets and T cells. A methodological limitation of our study in the context of T cell phenotype was that we gated the CD4 + and CD8 + cells without the use of CD3 staining. In fact, CD4 is also expressed in a small frequency of myeloid innate cells (i.e., monocytes and dendritic cells) and in B cells, while CD8 can also be identified on NK cells and dendritic cells [27]. Although there is usually only a small proportion of others immune cells, this aspect should be considered in future studies. In addition, we were unable to differentiate CD14- monocyte subsets to evaluate the expression of CD39, CD73, PD-1 and HLA-DR due to methodological aspects. Furthermore, viral load was not determinant to the diminished CD39 expression in CD14 + monocytes of severe COVID-19 patients.
By regulating purine concentrations in the extracellular space, the CD39/CD73 ectonucleotidase axis is important for fine-tuning innate differentiation and activity [28]. In this sense, the lower CD39 expression without changes in CD73 found in our study may indicate impaired capacity to metabolize extracellular ATP by monocytes which leads to the production of proinflammatory cytokines [29]. Interestingly, we [28] recently found an imbalance in CD39/CD73 axis in CD4 + and CD8 + T cells linked to alterations in adenine-based purine molecules and increased exhaustion markers in CD8 + T cells.
Here, we showed for the first time that viral load was associated with higher PD-1 expression and decreased HLA-DR expression in CD14 + monocytes. Downregulation of HLA-DR in monocytes is a hallmark of innate immune dysfunction during acute SARS-CoV-2 and associated with immunosuppression against opportunistic infections during hospital admission [30], [31]. Christensen and colleagues [32] observed decreased CD14 + HLA-DR + monocytes and higher PD-1 ligand (PD-L1) associated with decreased respiratory function in COVID-19 patients admitted in intensive care unit, indicating less antigen-presenting activity in more severe COVID-19. Furthermore, CD14 + HLADRlo/neg monocytes are a recognized monocytic myeloid-derived suppressor cells, a heterogeneous group of innate immune cells that possess strong immunosuppressive activities, and was also previously reported in severe COVID-19 patients [33]. Viral infection evokes cellular senescence as a primary stress response in infected cells. In fact, SARS-CoV-2 infection induces a senescence-associated secretory profile (SASP), compromising pro-inflammatory cytokines, pro-coagulant markers and disturbances in monocyte/macrophage activity [34]. Moreover, the upregulation of PD-1 and its ligand (i.e., PD-L1) on monocytes may play a role in several pathological conditions, including septic shock, cancers and hepatic infection [35], [36], [37]. Thus, monocyte exhaustion and downregulation in antigen presenting activity may be directly impacted by viral load during acute SARS-CoV-2 infection.
The upregulation of PD-1 expression in monocytes of COVID-19 patients was previously described, and was accompanied by alterations in metabolic response, such as reduced oxidative phosphorylation, reduced extracellular acidification rate and altered mitochondrial ultrastructure [11]. Here, we confirmed these results by changes in mitochondrial membrane polarization. In fact, changes in mitochondrial membrane potential generated by proton pumps (Complexes I, III and IV) in inner mitochondrial membrane alters the process of energy storage during oxidative phosphorylation [38]. Furthermore, we found increased mitochondrial ROS generation by monocytes of severe COVID-19 patients. Previous studies described that macrophages produce higher amounts of mitochondrial ROS for the killing of intracellular pathogens [39]. On the other hand, SARS-CoV-2 infection triggers mitochondrial ROS production which stabilize hypoxia-inducible factor-alpha1 (HIF-1α) and promotes glycolysis, these metabolic changes directly inhibit T cell response and aggravates the hypercytokinemia condition during severe COVID-19 [10], [40]. Compromised mitochondria respiration and increased ROS production are features of exhausted monocytes presenting low HLA-DR expression in septic conditions [13], [41], indicating that phenotypic changes in monocytes are accompanied by metabolic disturbances. The accumulation of dysfunctional mitochondria and increased ROS generation could lead to defective immune response, not only against SARS-CoV-2 but also increasing susceptibility to secondary infections, and the interaction between PD-1 signaling and mitochondrial dysfunction leading to increased ROS generation and tissue damage [42]. In this sense, a crossover between mitochondrial ROS production and increased viral replication may occur in severe COVID-19 patients with higher viral load, perpetuating the inflammatory response and monocyte dysfunction.
5. Conclusion
In conclusion, we showed that high SARS-CoV-2 viral load alters the systemic inflammatory profile, the bioactive lipid mediators and the redox state of severe COVID-19 patients at hospital admission. Elevated viral load was associated with alterations in activation and inhibitory immune checkpoints and mitochondrial ROS production by CD14 + monocytes. Furthermore, increased mitochondrial ROS and higher PD-1 expression in CD14 + monocytes of COVID-19 patients with high viral load may denote the premature exhaustion profile of innate immune cells through dysfunctional metabolic response induced by the infection. These data may explain why the higher viral load may lead to the severity of clinical outcomes during acute infection through alterations in immune response and redox state of the host.
Declaration of Competing Interest
The authors declare that they have no known competing financial interests or personal relationships that could have appeared to influence the work reported in this paper.
Acknowledgments
We are grateful to the Brazilian agencies Coordenação de Aperfeiçoamento de Pessoal de Nível Superior (CAPES) – Finance Code 001. This study was supported in part by the Hospital Moinhos de Vento through the Program for Supporting the Institutional Development of the Public Health System (PROADISUS), supported by the Ministry of Health of Brazil, FIOCRUZ Ribeirão Preto - TED 163/ 2019 - Processo: N˚ 25380.102201/2019-62/ Projeto Fiotec: PRES-009-FIO-20 and Universidade Federal de Ciências da Saúde de Porto Alegre (Programa de Fomento a Pesquisa). GPD is supported by postdoctoral fellowship from CAPES. PRTR, AP, MCM, FSL, MACF are grateful to CNPq for the PQ productivity scholarship.
Data accessibility statement: The data will be available when requested.
References
Citations & impact
Impact metrics
Citations of article over time
Alternative metrics

Discover the attention surrounding your research
https://www.altmetric.com/details/124675080
Smart citations by scite.ai
Explore citation contexts and check if this article has been
supported or disputed.
https://scite.ai/reports/10.1016/j.intimp.2022.108697
Article citations
Mitochondria in COVID-19: from cellular and molecular perspective.
Front Physiol, 15:1406635, 21 Jun 2024
Cited by: 0 articles | PMID: 38974521
Review
Optimizing cardiopulmonary rehabilitation duration for long COVID patients: an exercise physiology monitoring approach.
Geroscience, 46(5):4163-4183, 21 May 2024
Cited by: 0 articles | PMID: 38771423 | PMCID: PMC11336035
Precision nutrition to reset virus-induced human metabolic reprogramming and dysregulation (HMRD) in long-COVID.
NPJ Sci Food, 8(1):19, 30 Mar 2024
Cited by: 2 articles | PMID: 38555403 | PMCID: PMC10981760
Review Free full text in Europe PMC
Inhalation of ACE2-expressing lung exosomes provides prophylactic protection against SARS-CoV-2.
Nat Commun, 15(1):2236, 12 Mar 2024
Cited by: 0 articles | PMID: 38472181 | PMCID: PMC10933281
Altered mitochondrial respiration in peripheral blood mononuclear cells of post-acute sequelae of SARS-CoV-2 infection.
Mitochondrion, 75:101849, 09 Feb 2024
Cited by: 2 articles | PMID: 38341012 | PMCID: PMC11283875
Go to all (19) article citations
Similar Articles
To arrive at the top five similar articles we use a word-weighted algorithm to compare words from the Title and Abstract of each citation.
A Longitudinal Study of Immune Cells in Severe COVID-19 Patients.
Front Immunol, 11:580250, 23 Oct 2020
Cited by: 40 articles | PMID: 33178207 | PMCID: PMC7597438
CD14+CD16++ "nonclassical" monocytes are associated with endothelial dysfunction in patients with coronary artery disease.
Thromb Haemost, 117(5):971-980, 23 Feb 2017
Cited by: 42 articles | PMID: 28229168
Endotoxin tolerance and low activation of TLR-4/NF-κB axis in monocytes of COVID-19 patients.
J Mol Med (Berl), 101(1-2):183-195, 15 Feb 2023
Cited by: 5 articles | PMID: 36790534 | PMCID: PMC9930695
An unbalanced PD-L1/CD86 ratio in CD14(++)CD16(+) monocytes is correlated with HCV viremia during chronic HCV infection.
Cell Mol Immunol, 11(3):294-304, 17 Feb 2014
Cited by: 15 articles | PMID: 24531620 | PMCID: PMC4085489