Abstract
Free full text

The LEAP Process: Streamlining the Development of Long-Acting Products and Formulations for Infectious Diseases
Abstract
Developing long-acting products and formulations for infectious diseases is a nontrivial undertaking that is frequently classified as high risk and low reward by the pharmaceutical industry. The Long-Acting/Extended Release Antiretroviral Research Resource Program (LEAP) was founded in 2015 with the support of the National Institutes of Health to encourage, promote, and accelerate the development of such products. Assessment methodology for any new proposal brought to this group is part of a framework—the LEAP Process—that includes a landscape analysis of what is currently available in the public domain. This is followed by in silico modeling and simulation offered as a service to the relevant scientific community. A variety of preclinical and clinical outcome metrics are applied to each new agent as part of a continuous feedback loop to improve product characteristics. This allows us to catalog knowledge gaps and barriers that can be addressed by engaged stakeholders. Results are communicated in scientific articles, reviews, and position papers. This undertaking serves to de-risk discovery, development, and implementation by bridging the gaps between academic, regulatory, and industrial investigators, and by engaging those in the community who will be the eventual users of these medicines. The LEAP Process has supported formulations now approved for human immunodeficiency virus, as well as products in clinical and preclinical development for tuberculosis and hepatitis viruses B and C.
Long-acting (LA) drugs and formulations have revolutionized treatment and prevention strategies for a variety of health conditions. This includes drugs for schizophrenia, osteoporosis, hormonal contraception, and prostate cancer that can be administered as infrequently as once every 5 years [1, 2]. The attractions of LA devices and formulations include greatly enhanced patient convenience, adherence, reduced potential for stigmatization, and in many cases better efficacy and tolerability [1]. Regardless, the current availability of widely used LA products is limited to a relatively small number of conditions. Difficulties in discovering and bringing to market molecules that are appropriate for this form of delivery may create reluctance on the part of the pharmaceutical and biotechnology industries to pursue such products, but the area continues to gain traction in academic and industry environments.
The human immunodeficiency virus (HIV) epidemic created interesting possibilities for long-acting drugs used in both the treatment and prevention setting [1]. A combination of long-acting intramuscular cabotegravir and rilpivirine was approved by the US Food and Drug Administration as a once-monthly complete HIV treatment regimen in early 2021, and every-8-week cabotegravir intramuscular was approved for HIV prevention in 2022. Several other LA products for HIV are currently in clinical development. Although the initial success and popularity of these products provides proof of feasibility, this comes after significant reluctance on the part of some in the industry to pursue this method of drug delivery for this infection.
In 2015—recognizing the great value of the LA approach for HIV but also the great difficulties associated with discovery and development in this field—the National Institutes of Health (NIH) provided funding to support a Long-Acting/Extended Release Antiretroviral Research Resource Program (LEAP), an international program headquartered at Johns Hopkins University [3]. LEAP provides broadly based scientific support to accelerate the discovery and development of a class of drugs and formulations of high priority to the NIH but seen as potentially risky by the pharmaceutical industry. This program is supported by an R24 Research Resource mechanism. As such, LEAP is a first-of-its-kind research support program that helps transform the LA antiretroviral field by providing access to a broad set of scientific resources that includes academic and industrial investigators, regulatory experts, and representatives of communities affected by this epidemic. LEAP is composed of a central leadership and administrative support team, an Executive Committee of 15 academic and community experts, and a Pharmaceutical Advisory Board. Although originally formed to support antiretroviral drug development exclusively, in 2020 LEAP was granted authority to expand its mission to include tuberculosis and viral hepatitis, co-infections of great importance in the HIV epidemic and common diseases where availability of such anti-infective products could be revolutionary.
THE LEAP PROCESS
LEAP supports the drug discovery and development process through strategic engagement of academic and industrial investigators with 1 or more candidate LA therapeutics. Molecules, formulations, and/or devices are subjected to systematic evaluation using an iterative process developed by the Program (Figure 1). The LEAP Process begins with a landscape analysis conducted by experts affiliated with the Program that includes a systematic review of the literature to identify overlapping or competing products. Drug candidates are prioritized based on their clinical potential and perceived public health need. If significant shortcomings are identified early, then alternative strategies may be suggested (Figure 1).
Modeling and Simulation
An important technique for candidate evaluation and prioritization involves in silico modeling and simulation of product characteristics, including predicted human pharmacokinetics. The LEAP Modeling and Simulation Core Service, based in the Centre of Excellence for Long-acting Therapeutics at the University of Liverpool, is responsible for leading modeling and simulation efforts. Its domain includes both preclinical and clinical data. Preliminary modelling aims to characterize the feasibility of producing an LA formulation using either existing or novel drug delivery platforms, and the Core also addresses knowledge gaps relating to deployment of long-acting products in understudied populations (eg, pediatrics).
Feasibility and Outcomes
Knowledge gaps are identified and potential solutions are suggested to the affiliated investigators. We work with our partners in the pharmaceutical industry to assure that the proposed product could be produced to meet regulatory approval and marketing requirements. Ultimately, any supported product should be accessible to those in greatest need, including pediatric and adolescent populations. Assessment of both preclinical and clinical outcomes during development provides critical feedback to inform and improve subsequent iterations of the formulations in question, but also future related products (Figure 1).
Meetings
Face-to-face, hybrid, and virtual meetings are a critical component of the LEAP framework. Rigorous evaluation and assessment by the LEAP Process begins with meetings of investigators and other stakeholders (Figure 1). In addition, the LEAP Program sponsors an annual Workshop and Investigators Meeting that attracts up to 200 participants who review recent breakthroughs in LA/extended release [ER] drug and device development, participate in small-group breakout sessions to discuss important problems and knowledge gaps, and propose next steps. Plenary sessions and breakout group summaries are video recorded and posted on the LEAP website (https://www.longactinghiv.org/resource-categories/-conference-and-workshop-proceedings).
Ad hoc meetings of investigators and stakeholders may also be needed to advance the development process. An important example is a two-day Workshop on “Long Acting/Extended Release Antiretroviral Formulations for Children, Adolescents, and Pregnant Women: Knowledge Gaps and Approaches for Development,” held in November, 2017, in which 120 invited experts discussed barriers to progress in bringing specific LA/ER formulations to these populations of need. The major recommendations from this Workshop were published [4], and a Food and Drug Administration position paper cited the findings from this Workshop as being of “outstanding interest” [5].
Working Groups
Where needed, working groups are formed that consist of academic, regulatory, and pharmaceutical industry representatives with specific subject matter expertise. This mechanism has been used for approaches to the development of LA formulations for tuberculosis, hepatitis B virus, and hepatitis C virus. Community representatives are also included to provide product end user perspective. Working groups meet regularly by teleconference, usually monthly, and may also meet face to face at international conferences when convenient.
Intellectual Property
Addressing barriers to sharing of critical information among diverse parties requires attention to confidentiality and intellectual property issues. The general approach has been to assume that only nonconfidential information will be shared, and to identify situations where that is not the case as an exception to the usual process. For example, the LEAP Working Group on Tuberculosis negotiated a 6-party nondisclosure agreement that included the US government (NIH), 3 universities (Johns Hopkins University, the University of Nebraska, and the University of Liverpool), and 2 pharmaceutical companies (Janssen and Merck), to allow confidential discussion of data related to development of the first LA clinical candidate formulation for tuberculosis, LA-bedaquiline [6]. The Modelling and Simulation Core (see the following section) negotiates confidentiality agreements with pharmaceutical clients on an as-needed basis.
Communications
Public access to scientific data, deliberations, and developmental outcomes are critical to the success of the LEAP Process. The Program maintains a website (www.longactinghiv.org) that includes updates on relevant conferences and workshops, funding opportunities, publications, and other resources. Best practices for LEAP programs and projects mandate publication of results. Since 2015, the LEAP Process has contributed to 16 publications in peer-reviewed journals and 12 scientific abstracts.
THE IMPORTANT ROLE OF MODELING AND SIMULATION
The pharmacokinetics of anti-infective drugs represent 1 of the main determinants of efficacy and toxicity. Sufficient and sustained concentrations of anti-infectives in different tissues and organs are necessary to provide effective inhibition of pathogen replication. As for most small molecules, the pharmacokinetics of anti-infective drugs is often complex, and multiple barriers related to drug distribution can hinder the identification of suitable new drug candidates. Differences in pharmacokinetics often also underpin risk:benefit considerations for clinical management, including but not limited to drug–drug interactions, deployment in special populations, and ethnic differences in exposure.
Predictive computer-based methods can support the integration of available pharmacological knowledge and pharmacokinetic data to inform the rational development and management of anti-infective therapies but limitations relating to simulations need to be fully understood and accepted. The LEAP Process uses in silico modeling and simulation at all stages to improve the efficiency of lead candidate selection, streamline preclinical and clinical development, and create a continuous learning model that improves how the process is applied in the future (Figure 2). The core service emphasizes physiologically based pharmacokinetics (PBPK) models, which use a bottom-up systems approach to better understand how drug candidates are likely to be handled in a variety of in vivo situations [7].
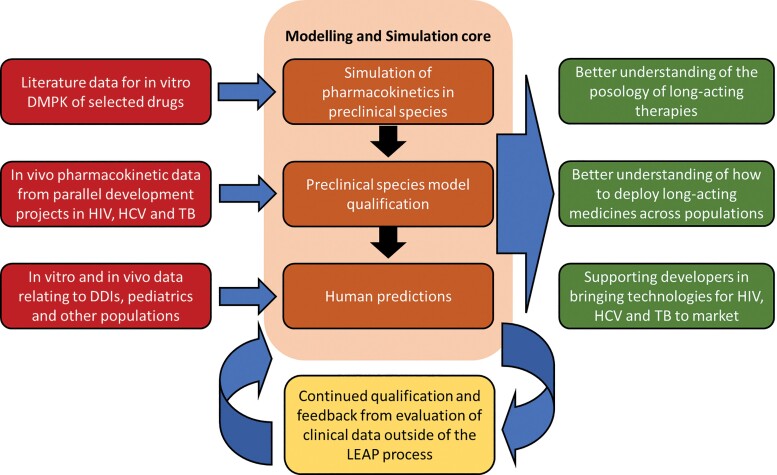
Integral role of the Long-Acting/Extended Release Antiretroviral Research Resource Program (LEAP) Modeling and Simulation Core in the LEAP Process. Abbreviations: DMPK, drug metabolism and pharmacokinetics; HIV, human immunodeficiency virus; HCV, hepatitis C virus; TB, tuberculosis; DDIs, drug-drug interactions.
The main limitations of mechanistic pharmacokinetic modelling include a broad variety of factors potentially influencing drug distribution and elimination. Having a detailed understanding of the underpinning mechanisms regulating absorption, distribution, metabolism, and elimination is essential to support a reliable quantitative framework. A suboptimal understanding and description of key pathways can lead to the misrepresentation of mathematical models of physiologic processes, and therefore poor pharmacokinetic predictions. Of particular relevance to LA drug delivery, major knowledge gaps remain regarding the mechanisms underpinning drug release, and marked species differences in drug absorption and exposure are evident, which complicates preclinical development.
PHYSIOLOGICALLY BASED PHARMACOKINETIC MODELING
Physiologically based pharmacokinetic modeling (also referred to as mechanistic pharmacokinetic modeling) is used across the spectrum from preclinical development to postmarketing optimization of drug dosing (posology). Quantitative descriptions of key processes such as absorption, distribution, metabolism, and elimination can be incorporated into mechanistic and PBPK models, which in turn present a framework to predict drug distribution and address complex pharmacological questions. PBPK modelling involves simulation of important drug processes by integrating previous knowledge on organism- and drug-specific characteristics [8]. PBPK modelling mimics the structure of the biological system being studied, and comprises distinct compartments that correspond to the physiology of tissues and organs that are connected via circulating blood and lymph. Circulation is subdivided into a venous and arterial pool. Typically, each compartment is defined by a blood flow rate and tissue volume; a tissue is described as either permeability rate limited, or perfusion rate limited [9].
The equations used in a PBPK model are derived from the law of mass action; they are used to describe the rate of change of the amount of drug within compartments over time. Four different types of mathematical equations have been used in models: (1) linear ordinary differential, routinely used to describe dynamic pharmacokinetic processes; (2) nonlinear differential, used when nonlinear processes are present within particular tissues (eg, binding and/or concentration-dependent elimination); (3) partial differential, when dispersion models are assumed; and (4) algebraic, used when processes are assumed to be static [10].
Once equations have been developed, the model requires input parameters. These can be split into 2 categories: physiologic and drug-specific. Physiologic input parameters are needed to characterize the physiological processes and anatomical structure of the species being studied (eg, body weight, height, blood flow, organ weights and volumes, enzyme ontogenies). These parameters are usually informed by data in the published literature. Drug-specific parameters, such as solubility, partitioning (eg, tissue:plasma coefficients and blood:plasma ratios), protein binding, logP, and clearance are used to characterize absorption, distribution, metabolism, and excretion–related processes. Drug-specific parameters can be derived from in silico predictive models based on the structure of the compound, in vivo methods, or in vitro experiments [10].
Whole-body PBPK models offer a mechanistic framework to measure drug concentrations in plasma and specific tissues over time, and can be combined with PD models to quantify the pharmacological effect of drugs. Examples of basic PD models may involve the quantitative prediction of pathogen replication in infections using basic proliferation models [11]. This modelling strategy can be successfully applied to simulate various complex clinical scenarios and therefore provide a rational framework for future research. This can also identify patients at higher risk of treatment failure or drug toxicity and suggest dose stratification [9]. The mechanistic modelling strategy is based on a detailed description of characterize absorption, distribution, metabolism, and excretion processes (eg, bioavailability, first pass metabolism, tissue distribution, clearance) underpinning PK, providing a quantitative estimate of drug concentrations in key organs and tissues such as lungs for tuberculosis [12] and liver for hepatitis C virus infection [13].
Several patient-specific characteristics such as age, weight, pregnancy, hepatic, and/or renal impairment can cause significant changes in drug absorption, distribution, metabolism, and elimination. Limited knowledge on how these individual parameters affect the PK of drugs mean fewer therapeutics options are available, and also sometimes necessitates off-label drug use. PBPK models have been extensively developed to assess special populations by integrating knowledge on the physiological and anatomical changes that occur, and provide information on optimized dosing regimens [9]. For most special population PBPK models, the structure is adapted from an already validated healthy or physiologically normal adult PBPK model. Appropriate changes in anatomy and physiology are considered using mathematical equations and/or additional compartments. PBPK models developed by the LEAP Process have predicted the PK properties of approved LA formulations of intramuscular cabotegravir and rilpivirine in children and adolescents [14], which should be qualified soon as part of several ongoing clinical studies.
Other examples exist relating to orally administered drugs for which successful qualification has already occurred. For example, the pharmacokinetics of efavirenz (EFV) in pregnancy was predicted via PBPK modelling: Chetty et al evaluated a lower dose of EFV (400 mg) in pregnant women to determine whether this dose would be therapeutically equivalent to a 600-mg dose in pregnancy, as had been clinically observed in the nonpregnant population. The model was validated using available clinical pharmacokinetic data for a 600-mg dose of EFV in pregnancy. Their results suggested a 2-fold increase in EFV clearance in the third trimester of pregnancy, consistent with clinical observations, indicating that the reduced EFV dose may not provide therapeutic drug concentrations in some extensive metabolizers [15].
PBPK modelling has been successfully integrated into the regulatory process for small molecule drugs in a number of scenarios. For example, PBPK is used to support first-in-human dose prediction and in specific situations has replaced clinical trials to inform labelling changes for specific drug–drug interactions [9]. Regulatory authorities may require that a PBPK model be suitably qualified by orthogonal analyses (eg, observations in animals) before inclusion within a regulatory submission.
APPLICATION TO SELECTION OF LONG-ACTING DRUGS AND FORMULATIONS
Multiple approaches have been described in the scientific literature to enhance drug distribution and elimination, and an increasing number of novel drug delivery technologies are the subject of intensive research. Advanced materials can support the sustained release of drugs over a prolonged time and allow the long-acting administration of key anti-infective drugs. Modified formulations may enhance drug penetration into key organs and tissues and support more effective inhibition of pathogen replication. PBPK modelling has utility in identifying the delivery strategies that may best translate into beneficial clinical performance. Frequency of administration and the combination of loading or maintenance doses can be simulated to better understand potential options for dosing. Moreover, PBPK models can be applied to describe the geometrical structure and interaction between formulations, tissues, and cells, supporting an informed design of advanced materials and formulations.
Drugs with different physicochemical properties have different compatibility with different delivery platforms, such as injectable technologies, implants, and/or microarray (microneedle) patches. The pharmacokinetic and physicochemical characteristics of the drug have a major influence on the success of long-acting medicine compatibility. PBPK models can simulate multiple scenarios to identify those with the best chance of producing therapeutic drug concentrations [7]. Validated PBPK models developed as part of the LEAP Process have identified anti-infective formulations most compatible with multiple platforms and disease applications [16, 17]. As part of the LEAP Process, data from a large number of parallel research projects are used to qualify predictions arising from the Modelling and Simulation Core Service, but residual uncertainty should be accepted before ultimate demonstration in clinical trials.
OUTCOMES AFTER 5 YEARS
The LEAP Process has contributed to progress in the field in a number of ways. This includes: (1) organizing a scientific collaboration that led to the first LA/ER formulation of an approved antimalarial drug, atovaquone [18]; (2) demonstrating the utility of in silico modelling to predict an important clinical drug–drug interaction of an LA/ER antiretroviral (ARV), cabotegravir, with rifampin, which has been incorporated into regulatory product labelling [19]; (3) supporting the design and publication of the first survey on acceptability of LA/ER ARV injectables and implants in adolescents and young adults [20]; (4) publishing the first comprehensive reviews of implant technology for HIV drug delivery [2, 21]; and (5) publishing a commentary on the need for LA/ER formulations having activity against hepatitis B virus [22]. Documenting LEAP Process outcomes also required establishing the first online resource dedicated to LA/ER ARV development (www.longactinghiv.org); this website receives more than 30 000 documented visits each year.
The LEAP Modelling and Simulation Core has been a major driver of innovation in this field. Important highlights are summarized in Table 1. Accomplishments included a modelling strategy for the simulation of nanoparticles that can reach the systemic circulation and, therefore, maximize the penetration of drugs in key tissues and organs [23]. We have also modelled ARV PK after administration of a hypothetical subcutaneous implantable device with zero-order release kinetics with a systemic intracellular depot [24], and integrated quantitative structure-activity relationship models into PBPK to accelerate the identification of novel candidates (see Table 1). The application of this information to preclinical formulation development and early clinical phase I study results could greatly improve the efficiency of identifying the most promising drug candidates and bringing them into clinical trials.
Table 1.
Selected Accomplishments of the LEAP Modelling and Simulation Core Service
Drug(s) | Disease | Key Findings | Reference |
---|---|---|---|
Cabotegravir, rilpivirine | HIV | • Optimal dose prediction of LAI cabotegravir and rilpivirine in children/adolescents aged 3–18 y. • Loading dose and maintenance dose prediction of cabotegravir ranged from 200 to 600 mg and from 100 to 250 mg, respectively, and for rilpivirine it ranged from 250 to 550 mg and from 150 to 500 mg, respectively, across various weight groups of children ranging from 15 to 70 kg. | [14] |
Cabotegravir, rilpivirine | HIV | • Optimal dose and release rate has been determined for administration of cabotegravir and rilpivirine via a microarray patch. • Based on the simulations, patch sizes less than 60 cm2 should be viable for LA delivery. • These simulations underpinned a successful grant application to the UK engineering and physical sciences research council (EPSRC) and microarray development at Queens University Belfast. • The modelling approach has been further applied for the design of multipurpose prevention technology supporting the coadministration of ARVs and contraceptives. | [17] |
Tenofovir alafenamide | HIV | • The feasibility of a TAF subcutaneous implant providing plasma concentrations for up to 6 mo was assessed. • Plasma, intracellular, and tissue tenofovir triphosphate concentrations were simulated to capture the preventive potential. Release rates above 1.4 mg/d were identified as optimal and are being used to inform implant development at RTI. | [24] |
Rifampicin, cabotegravir, rilpivirine | TB/HIV | • Dramatic reduction in exposure of cabotegravir and rilpivirine was predicted when administered with rifampicin. • Half-lives predicted to remain unchanged resulting in several weeks of suboptimal exposure. • Preliminary recommendation that co-administration should be avoided. | [19] |
Bedaquiline, delamanid, isoniazid, rifapentine | TB | • Optimal release rates to achieve monthly therapeutic exposure were successfully identified for all drugs studied. • Key recommendations about the compatibility of existing TB therapies as potential LAI candidates were developed. | [16] |
Glecaprevir, pibrentasvir | HCV | • To date, the compatibiltiy and optimal release rate for glecaprivir has been identified and work is ongoing to develop models for pibrentasvir and both drugs in combination. | Ongoing work, unpublished |
Abbreviations: ARV, ; HCV, hepatitis C virus; LA, long acting; LAI, long-acting injectable; LEAP, Long-Acting/Extended Release Antiretroviral Research Resource Program; RTI, Research Triangle Institute; TAF, tenofovir alafenamide; TB, tuberculosis.
The Modelling and Simulation Core was commissioned by the NIH-supported International Maternal Pediatric and Adolescent Clinical Trials Network to develop dosing recommendations for LA-cabotegravir use in neonates. This ongoing phase 1 study was based on work we published for dosing of LA-cabotegravir and LA-rilpivirine in infants and children [14].
THE FUTURE
The LEAP Process is iterative—part of a feedback loop for continuous learning and process improvement. Future directions will include initiatives on a number of fronts. To centralize access to the status of product development, we are collaborating with the Medicines Patent Pool and Unitaid on a publically accessible LA product landscape, The Long-Acting Technologies Patents and Licences Database, that will include information on product name, pharmacologic characteristics, stage of development, status of patents and intellectual property, as well as related publications (accessible at https://lapal.medicinespatentpool.org).
To develop next-generation predictive strategies, the Modelling and Simulation Core will continue to pursue the validation of model accuracy with a growing library of preclinical and clinical data. In accordance with best practices, models are considered as appropriately qualified if predictions are within 0.8- to 1.2-fold difference from observed clinical and/or preclinical data, but residual uncertainty should always be acknowledged. Additionally, the selected computational strategy will allow the estimation of the effect of relevant patient characteristics on drug distribution (eg, cirrhosis and relative change in blood flow and CYP expression; pregnancy and changes in protein binding and therefore volume of distribution) supporting a comprehensive evaluation of suitability of LA/ER dosing strategies in various patient populations [25, 26]. The PBPK modelling will be further integrated into PK/PD approaches for disease-specific consideration for additional therapeutic areas that include tuberculosis and viral hepatitis [27, 28]. This will include identification of appropriate target concentrations and PK/PD assumptions for these important disease areas.
Finally, we see this effort as being vital in a public health approach, where profits alone are not sufficient to drive the development of transformative but high-risk products [29]. It is our hope that public access to the methods and results of the LEAP Process will further that aim.
Contributor Information
Charles Flexner, Divisions of Clinical Pharmacology and Infectious Diseases, Department of Medicine, Johns Hopkins University School of Medicine, Johns Hopkins University, Baltimore, Maryland, USA. Department of Pharmacology and Molecular Sciences, Johns Hopkins University School of Medicine, Johns Hopkins University, Baltimore, Maryland, USA. Department of International Health, Bloomberg School of Public Health, Johns Hopkins University, Baltimore, Maryland, USA.
Marco Siccardi, Department of Molecular and Clinical Pharmacology, University of Liverpool, Liverpool, United Kingdom.
Fazila Bunglawala, Department of Molecular and Clinical Pharmacology, University of Liverpool, Liverpool, United Kingdom.
Andrew Owen, Department of Molecular and Clinical Pharmacology, University of Liverpool, Liverpool, United Kingdom.
Notes
Acknowledgments. The authors thank Jane McKenzie-White and Matthew Williams for assistance in the preparation of this manuscript.
Financial support. C. F. received related support during the preparation of this manuscript from NIH grant NIAID R24 AI118397, Long-Acting/Extended Release Antiretroviral Resource Program (LEAP), www.longactinghiv.org, awarded to Johns Hopkins University. A. O. acknowledges funding from Unitaid for project LONGEVITY, Wellcome Trust (222489/Z/21/Z), EPSRC (EP/R024804/1; EP/S012265/1), and NIH (R01AI134091; R24AI118397).
Supplement sponsorship. This article appears as part of the supplement “Long-Acting and Extended-Release Formulations for the Treatment and Prevention of Infectious Diseases,” sponsored by the Long-Acting/Extended Release Antiretroviral Research Resource Program (LEAP).
References
Articles from Clinical Infectious Diseases: An Official Publication of the Infectious Diseases Society of America are provided here courtesy of Oxford University Press
Full text links
Read article at publisher's site: https://doi.org/10.1093/cid/ciac750
Read article for free, from open access legal sources, via Unpaywall:
https://academic.oup.com/cid/article-pdf/75/Supplement_4/S502/50491176/ciac750.pdf
Citations & impact
Impact metrics
Alternative metrics

Discover the attention surrounding your research
https://www.altmetric.com/details/138747095
Smart citations by scite.ai
Explore citation contexts and check if this article has been
supported or disputed.
https://scite.ai/reports/10.1093/cid/ciac750
Article citations
Projected health and economic effects of a pan-tuberculosis treatment regimen: a modelling study.
Lancet Glob Health, 12(10):e1629-e1637, 16 Aug 2024
Cited by: 0 articles | PMID: 39159654 | PMCID: PMC11413512
A Holistic Review of the Preclinical Landscape for Long-Acting Anti-infective Drugs Using HIV as a Paradigm.
Clin Infect Dis, 75(suppl 4):S490-S497, 01 Nov 2022
Cited by: 1 article | PMID: 36410386 | PMCID: PMC10200324
Review Free full text in Europe PMC
Similar Articles
To arrive at the top five similar articles we use a word-weighted algorithm to compare words from the Title and Abstract of each citation.
[Development of antituberculous drugs: current status and future prospects].
Kekkaku, 81(12):753-774, 01 Dec 2006
Cited by: 27 articles | PMID: 17240921
Review
Tuberculosis
The International Bank for Reconstruction and Development / The World Bank, Washington (DC), 14 Sep 2018
Cited by: 0 articles | PMID: 30212088
ReviewBooks & documents Free full text in Europe PMC
Creating demand for long-acting formulations for the treatment and prevention of HIV, tuberculosis, and viral hepatitis.
Curr Opin HIV AIDS, 14(1):13-20, 01 Jan 2019
Cited by: 13 articles | PMID: 30394948
Review
Folic acid supplementation and malaria susceptibility and severity among people taking antifolate antimalarial drugs in endemic areas.
Cochrane Database Syst Rev, 2(2022), 01 Feb 2022
Cited by: 12 articles | PMID: 36321557 | PMCID: PMC8805585
Review Free full text in Europe PMC
Funding
Funders who supported this work.
EPSRC (2)
Grant ID: EP/S012265/1
Grant ID: EP/R024804/1
Engineering and Physical Sciences Research Council (2)
Grant ID: EP/S012265/1
Grant ID: EP/R024804/1
Johns Hopkins University
Long-Acting/Extended Release Antiretroviral Resource Program
NIAID NIH HHS (2)
Grant ID: R01AI134091
Grant ID: R24 AI118397
NIH (3)
Grant ID: R01AI134091
Grant ID: NIAID R24 AI118397
Grant ID: R24AI118397
NIH HHS (1)
Grant ID: NIAID R24 AI118397
Wellcome Trust (1)
Grant ID: 222489/Z/21/Z