Abstract
Free full text

Re‐evaluation of certain aspects of the EFSA Scientific Opinion of April 2010 on risk assessment of parasites in fishery products, based on new scientific data. Part 1: ToRs1–3
Abstract
Surveillance data published since 2010, although limited, showed that there is no evidence of zoonotic parasite infection in market quality Atlantic salmon, marine rainbow trout, gilthead seabream, turbot, meagre, Atlantic halibut, common carp and European catfish. No studies were found for greater amberjack, brown trout, African catfish, European eel and pikeperch. Anisakis pegreffii, A. simplex (s. s.) and Cryptocotyle lingua were found in European seabass, Atlantic bluefin tuna and/or cod, and Pseudamphistomum truncatum and Paracoenogonimus ovatus in tench, produced in open offshore cages or flow‐through ponds or tanks. It is almost certain that fish produced in closed recirculating aquaculture systems (RAS) or flow‐through facilities with filtered water intake and exclusively fed heat‐treated feed are free of zoonotic parasites. Since the last EFSA opinion, the UV‐press and artificial digestion methods have been developed into ISO standards to detect parasites in fish, while new UV‐scanning, optical, molecular and OMICs technologies and methodologies have been developed for the detection, visualisation, isolation and/or identification of zoonotic parasites in fish. Freezing and heating continue to be the most efficient methods to kill parasites in fishery products. High‐pressure processing may be suitable for some specific products. Pulsed electric field is a promising technology although further development is needed. Ultrasound treatments were not effective. Traditional dry salting of anchovies successfully inactivated Anisakis. Studies on other traditional processes – air‐drying and double salting (brine salting plus dry salting) – suggest that anisakids are successfully inactivated, but more data covering these and other parasites in more fish species and products is required to determine if these processes are always effective. Marinade combinations with anchovies have not effectively inactivated anisakids. Natural products, essential oils and plant extracts, may kill parasites but safety and organoleptic data are lacking. Advanced processing techniques for intelligent gutting and trimming are being developed to remove parasites from fish.
SUMMARY
In 2010, the EFSA BIOHAZ Panel published a scientific opinion on food safety related to parasites in fishery products, focusing on possible allergic reactions and the evaluation of alternative methods to freezing for killing viable parasites. Fish‐borne human diseases were primarily caused by infection with viable cestode, trematode and nematode parasites, although allergic reactions were also possible when the parasite was Anisakis simplex (s. l.). Freezing (e.g. core temperature at −15°C for at least 96 h, at −20°C for at least 24 h or at −35°C for at least 15 h) and heat treatments (e.g. core temperature of at least 60°C for at least 1 min) were the most effective for killing fish‐borne parasites including their larvae. There was insufficient information to determine whether or not alternative treatments such as high hydrostatic pressure, irradiation, drying and low voltage currents, were effective for killing Anisakidae larvae, but it was concluded that many traditional marinating and cold smoking methods were not sufficient to kill these larvae.
The European Commission asked EFSA to update certain aspects of this BIOHAZ 2010 scientific opinion on risk assessment of parasites in fishery products based on any new scientific evidence that may have become available since then. In particular, EFSA was requested to review and assess:
ToR1: The occurrence of parasites of public health importance in fishery products derived from the most relevant farmed fish species in the EU (in particular, but not limited to, Atlantic salmon, seabass, farmed seabream and turbot).
ToR2: Diagnostic methods for the detection of parasites of public health importance in fishery products from such farmed fish species.
ToR3: Technical developments and new scientific data available in relation to killing viable parasites of public health importance in fishery products, in particular treatments other than freezing, and
ToR4: Whether any particular species of wild caught fish originating from specific fishing grounds could be regarded as not representing a health hazard with regard to the presence of parasites of public health importance.
This opinion covers ToRs 1–3.
Aquaculture in the EU currently produces ~1.1 million tonnes of aquatic organisms worth €4.2 billion annually. The annual finfish production in the non‐EU countries Norway and Iceland (EFTA), UK and Faroe Islands slightly exceeds 2.0 million tonnes, of which Norway alone produces more than 1.66 million tonnes, mostly consisting of Atlantic salmon. The mean per capita annual consumption of fishery products in the EU is ~
24 kg with one quarter of this (~
1.25 million tonnes) being supplied by aquaculture. Tuna (all species combined, mostly wild), Atlantic salmon (mostly farmed), cod (mostly wild) and Alaska pollock (wild) were the four most consumed fishes in the EU.
Based on production and consumption data, the farmed finfish species considered most relevant and thus considered in this opinion include: Atlantic salmon, Salmo salar; rainbow trout, Oncorhynchus mykiss; gilthead seabream, Sparus aurata; European seabass, Dicentrarchus labrax; Atlantic bluefin tuna, Thunnus thynnus; turbot, Scophthalmus maximus; meagre, Argyrosomus regius; Atlantic halibut, Hippoglossus hippoglossus; Atlantic cod, Gadus morhua; and greater amberjack, Seriola dumerilii, all of which are produced in marine aquaculture, as well as rainbow trout, Oncorhynchus mykiss; brown trout, Salmo trutta; common carp, Cyprinus carpio; European eel, Anguilla anguilla; European catfish, Silurus glanis, African catfish, Clarias gariepinus; tench, Tinca tinca; and pikeperch, Sander lucioperca, from freshwater systems.
The most important zoonotic fish‐borne parasites of public health importance occurring in the wild and to which farmed fish produced in EU/EFTA aquaculture could be exposed include, in the marine environment, the nematodes A. simplex (s. s.), A. pegreffii, Phocanema decipiens (s. l.) and Contracaecum osculatum (s. l.) as well as the trematode Cryptocotyle lingua; and in freshwater ecosystems, the trematodes Opisthorchis felineus, Metorchis spp., Pseudamphistomum truncatum, Paracoenogonimus ovatus and the cestode Dibothriocephalus spp.
Fish from seawater land‐based recirculating aquaculture systems (RAS), or indoor or in roofed farming facilities with filtered and/or treated water intake and exclusively fed heat‐treated pelleted feed, are unlikely to be exposed to zoonotic parasites. In contrast, fish farmed in open marine offshore cages or open flow‐through freshwater ponds or tanks may be exposed to zoonotic parasites. Since 2010, the number of studies testing fish for parasites were limited including in Atlantic salmon (4), marine rainbow trout (5), gilthead seabream (9), European seabass (10), Atlantic bluefin tuna (2), turbot (3), meagre (1), Atlantic halibut (1), Atlantic cod (1), freshwater rainbow trout (1), European catfish (1), tench (1) and common carp (1). No studies were available for greater amberjack, brown trout, African catfish, European eel and pikeperch. The studies available were primarily testing for anisakids and the majority failed to detect parasites in the fish. The exceptions were European seabass, where two of the 10 studies reported two A. pegreffii and two A. simplex (s. l.) larvae in three fish (of which 1 larva was detected in the fillet); Atlantic bluefin tuna, where A. pegreffii and A. simplex (s. s.) were detected with prevalence values ranging from 17.1% to 32.8%; and Atlantic cod infected with C. lingua and A. simplex (s. l.), with prevalence of 55%–79% for the first, and 1% for the latter. Potentially zoonotic freshwater trematodes (P. ovatus and P. truncatum) were detected in tench only. No cestodes (Dibothriocephalus spp.) were found in any study of farmed fish in Europe.
These parasites can be detected directly from the fishery products via visual inspection including candling, artificial digestion (ISO 23036‐2), UV‐press (ISO 23036‐1) and further identified to species level by morphological (morphometrics) and molecular approaches (PCR, qPCR). Additionally, parasite‐specific molecules present within the fishery product matrix can be detected using molecular methods such as immunoassays, quantitative PCR (qPCR) and proteomics. The molecular identification of isolated parasites from fishery products has been significantly improved by the application of PCR‐amplification/sequencing studies targeting both nuclear and mitochondrial sequences in the parasites. When applied in combination with a microscopic identification technique, these molecular methods should be regarded as the most reliable identification methods.
Since the last EFSA BIOHAZ Panel (2010) scientific opinion, new technologies and methodologies have been developed for the detection, visualisation and isolation of zoonotic parasites in fish products and for the specific identification of the isolated parasites. These include new UV‐scanning devices for the detection of Anisakis in fishery products and novel optical (hyperspectral) sensing methodologies. However, further development and validation are required before wide scale application. The application of artificial intelligence and machine learning algorithms in image and video processing are also being tested to support the high‐throughput detection, prediction of occurrence and identification of parasites in fishery products.
Omics (genomics, metagenomics, transcriptomics and proteomics) generated data are also a recent and useful resource for the selection of further molecular/genetic markers to be used for the identification and characterisation of zoonotic parasites.
There are several treatments for inactivating parasites in fishery products. Since 2010, additional information and data are available for freezing, heating (conventional and microwave), high‐pressure processing (HPP), pulsed electric fields (PEF), drying, ultrasounds, salting, marinating and the use of natural products. The susceptibility of Anisakis larvae to isothermal lethal conditions has been mathematically described by the Weibull model. HPP employing specific pressure–time combinations that maintain the sensory characteristics can be applied for some products, and the potential of PEF has been explored although further development is needed. Ultrasound treatments have not shown to be effective. Traditional dry salting processes of anchovies successfully inactivated Anisakis. Studies on other traditional preservation and processing methods such as air‐drying of Arctic migrating cod (‘stockfish’), double salting (salting in brine plus dry salting) of anchovies and cod (‘baccalà’) also suggest that anisakids are successfully inactivated. However, more data covering these and other parasites in more fish species and products are required to determine if these processes are always effective. Marinade combinations with anchovies have not proven successful. There are also several studies investigating the potential use of natural products, such as essential oils and plant extracts which may kill parasites but data on safety (toxicity) and organoleptic properties are lacking. Most studies target nematodes of the family Anisakidae, with less information on the trematodes and cestodes.
Advanced processing techniques for intelligent gutting and trimming operations are becoming available and their implementation could have a significant impact on the removal of parasites. Other strategies for the removal of parasites by chemical procedures are still in the proof‐of‐concept phase.
1. INTRODUCTION
1.1. Background and Terms of Reference as provided by the requestor
In 2010 EFSA published a scientific opinion on risk assessment of parasites in fishery products. 1 EFSA was requested to analyse three aspects:
Assessment of food safety concerns due to possible allergic reactions from parasites in fishery products;
Alternative treatments for killing viable parasites and comparison with freezing method;
Criteria for when fishing grounds (wild‐farmed) fishery products do not present a health hazard (Atlantic salmon in particular). EFSA conclusions were considered for modifying part D of Annex III, Section VIII, Chapter III to Regulation (EC) No 853/2004 2 (Commission Regulation (EU) No 1276/2011).
In fact, part D of Annex III, Section VIII, Chapter III to Regulation (EC) No 853/2004 establishes that:
Food business operators placing on the market the following fishery products derived from finfish or cephalopods:
fishery products intended to be consumed raw; or
marinated, salted and any other treated fishery products, if the treatment is insufficient to kill the viable parasites;
must ensure that the raw material or finished product undergo a freezing treatment to kill viable parasites that may be a risk to the health of the consumer.
The freezing treatment is not carried out for fishery products:
that have undergone or are intended to undergo before consumption a heat treatment that kills the viable parasite. In the case of parasites other than trematodes the product is heated to a core temperature of 60°C or more for at least 1 min;
that have been preserved as frozen fishery products for a sufficiently long period to kill the viable parasites;
from wild catches, provided that: (i) there are epidemiological data available indicating that the fishing grounds of origin do not present a health hazard with regard to the presence of parasites; and (ii) the competent authority so authorises;
derived from fish farming, cultured from embryos and have been fed exclusively on a diet that cannot contain viable parasites that present a health hazard, and one of the following requirements is complied with:
have been exclusively reared in an environment that is free from viable parasites; or
the food business operator verifies through procedures, approved by the competent authority, that the fishery products do not represent a health hazard with regard to the presence of viable parasites.
Before placing on the market fishery products which have not undergone the freezing treatment or which are not intended to undergo before consumption a treatment that kills viable parasites that present a health hazard, a food business operator must ensure that the fishery products originate from a fishing ground or fish farming which complies with the specific conditions referred to in one of those points. This provision may be met by information in the commercial document or by any other information accompanying the fishery products.
The ParaFishControl is an EU H2020‐funded project that aims at increasing the sustainability and competitiveness of the European aquaculture industry by improving our understanding of fish‐parasite interactions and by developing innovative solutions and tools for the prevention, control and mitigation of the most harmful parasitic species affecting the main European farmed fish species. The project started in 2015 and finished in 2020 and had a consortium of 29 partners (public and private) from 13 countries. Research related to that project demonstrated in farmed seabass, farmed seabream, turbot, and sea caged rainbow trout that no zoonotic parasites were found, concluding that the risk related to zoonotic Anisakidae is negligible. The authors suggested that there is groundwork for amending the current legislation.
Other studies demonstrated that farmed fish were found to be less infected in comparison with wild fish (2%) but not Anisakis free. Farmed fish is in general reported to be considerably less infected and therefore representing a very limited food safety risk, but guaranteeing nematode free fish remains impossible.
In addition, the EFSA BIOHAZ Panel (2010) Opinion concluded that ‘no sea fishing grounds can be considered free of A. simplex larvae’, and that ‘all wild caught seawater and freshwater fish must be considered at risk of containing viable parasites of human health hazard if these products are to be eaten raw or almost raw’. Furthermore, the BIOHAZ Panel recommended the collection of systematic data on the complete life cycle, geographical and seasonal distribution, prevalence, intensity, and anatomical location of parasites of public health importance in wild caught fishery products.
The European Union One Health 2020 Zoonoses report elaborated by EFSA and ECDC reports that in 2020, Anisakis caused two outbreaks, both reported by Spain, involving six individuals. No outbreaks were reported in 2019. The causative agent was not identified at the species level.
TERMS OF REFERENCE
EFSA is asked to update certain aspects of its Scientific Opinion of April 2010 on risk assessment of parasites in fishery products based on any new scientific evidence that may have become available since then. In particular, EFSA is requested to review and assess:
The occurrence of parasites of public health importance in fishery products derived from the most relevant farmed fish species in the EU (in particular, but not limited to, Atlantic salmon, seabass, farmed seabream and turbot).
Diagnostic methods for the detection of parasites of public health importance in fishery products from such farmed fish species.
Technical developments and new scientific data available in relation to killing viable parasites of public health importance in fishery products, in particular treatments other than freezing.
Whether any particular species of wild caught fish originating from specific fishing grounds could be regarded as not representing a health hazard with regards to the presence of parasites of public health importance.
The deadline for this scientific opinion, addressing ToRs1‐3, is by 31 March 2024. For a 2nd opinion, addressing ToR4, 31 December 2024.
1.2. Interpretation of the Terms of Reference
The following has been clarified with the requestor:
Whereas the legal definition, according to (EC) No 853/2004, for ‘Fishery products’ means all marine water or freshwater animals (except for live bivalve molluscs, live echinoderms, live tunicates and live marine gastropods, and all mammals, reptiles and frogs) whether wild or farmed and including all edible forms, parts and products of such animals, for the current opinion only finfish species will be covered.
ToR1 covers aquaculture within the European Union and the European Free Trade Association (EU/EFTA). 3 Data from the Faroe Islands and United Kingdom (UK) will also be considered in the assessment due to their importance as European exporters of farmed finfish into the EU. Fish farmed outside these European areas/countries are excluded.
ToR2 and ToR3 cover parasite detection and inactivation methods, respectively, used for finfish (both wild caught and farmed fish).
ToR4 will be presented in a separated opinion (Part 2). It will cover all fishing grounds for caught fish that are consumed in the EU/EFTA market.
While the previous EFSA scientific opinion from 2010 (EFSA BIOHAZ Panel, 2010) will be considered, the WG will focus on new information and data generated since then. Accordingly, the information/literature/data to be revised will cover the period from 2010 (inclusive) to date.
Allergies will not be covered by the current assessment.
Additional clarification done by the working group members:
In the context of this opinion, the parasites considered of public health importance will be those parasites that are known to be zoonotic or are potentially zoonotic (updating those considered for EFSA BIOHAZ Panel, 2010).
Only parasites that infect fish are considered. Parasites that do not infect fish but are found in contaminated waters (e.g. Cryptosporidium, Giardia, Toxoplasma) and may be present in their gastrointestinal tract and subsequently cross‐contaminate fish during processing are excluded.
Relevant finfish species will be defined considering data from the EU/EFTA aquaculture production and EU/EFTA consumption, and thus their economic and dietary importance.
With regard to the fishery products, only the edible parts of the finfish will be considered.
In ToR1, occurrence refers to the frequencies in which parasites are found to be present in each of the indicated most relevant finfish species. Epidemiological data on prevalence (number of infected fish/number of analysed fish ×100, in percentage), mean abundance (total number of parasites of a particular species recorded in a set of analysed fish/number of analysed fish) and mean intensity (total number of parasites of a particular species recorded in a set of analysed fish/number of infected fishes) (according to Bush et al., 1997) as well as data on presence/absence (in some studies, this may be all that is reported), will be considered.
In ToR2, both the visual and molecular‐based detection/identification methods will be considered.
In ToR3, treatments for the inactivation and/or removal of viable parasites of public health importance in fishery products will be considered. Treatments may be of a physical or chemical nature or may be a combination of treatments.
Each ToR was translated into an assessment question (AQ) and if applicable, into sub‐assessment questions (SQ1) (Annex A). These read as follows:
AQ1: What is the occurrence of parasites of public health importance in fishery products derived from the most relevant farmed finfish species in the EU/EFTA?
SQ1.1: Which are the most relevant farmed fish species produced in the EU/EFTA, in addition to Atlantic salmon, seabass, seabream and turbot that may be infected with parasites of public health importance?
SQ1.2: Which are the parasites of public health importance that could infect the most relevant farmed finfish species in the EU/EFTA (from SQ1.1)?
SQ1.3: Considering SQ1.1 and SQ1.2, what is the occurrence of parasites of public health importance in fishery products derived from the most relevant farmed finfish species in the EU/EFTA (from SQ1.1)?
AQ2: What testing methods are currently available and may be available in the near future to test the fish species for the parasites identified in the answer to ToR1?
AQ3. What technical developments and new scientific data for inactivation and/or removal of viable parasites (identified in the answer to ToR1) in fishery products, in particular treatments other than freezing, have been described since the EFSA BIOHAZ Panel (2010) scientific opinion?
1.3. Additional information
1.3.1. EFSA BIOHAZ 2010 scientific opinion
In 2010, EFSA published a scientific opinion on food safety related to parasites in fishery products (EFSA BIOHAZ Panel, 2010). Possible allergic reactions to parasites in fishery products were assessed and alternative treatments for killing viable parasites were evaluated as compared to the freezing method. The main identified fishery product‐borne parasitic diseases for humans were caused by cestodes, trematodes and nematodes. The associated diseases were mainly caused by infection with the viable parasite. Allergic reactions to parasites in fishery products were also possible, but based on the limited data available, these were only caused by Anisakis simplex. During the first infection with this parasite, the patient is sensitised, and subsequent allergic reactions only require exposure to the antigen (the live parasite may not be present) and may be severe. This allergic reaction and gastro‐allergic anisakiasis is relatively common in some regions of Spain, Italy and Croatia but is rarely reported in other parts of Europe.
Although most studies on the efficacy of physical and chemical treatments focused on A. simplex, it was considered that the properties of other multicellular parasites were likely to be similar, apart from the trematode developmental stage metacercariae, which are more heat resistant. Freezing and heat treatments were the most effective processes for killing fish‐borne parasites including their larvae, when undertaken under specific conditions. These include, for example, freezing (core temperature) at −15°C for at least 96 h, at −20°C for at least 24 h or at −35°C for at least 15 h. Heat treatment should achieve a core temperature of at least 60°C for at least 1 min. There was insufficient information to determine whether or not alternative treatments, including high hydrostatic pressure (also known as high‐pressure processing, HPP, used throughout the current document), irradiation, drying and low voltage currents, were effective for killing Anisakidae larvae as an alternative to freezing. However, it was clear that many traditional marinating and cold smoking methods were not sufficient to kill these larvae.
The criteria used to determine whether fishery products from specific fishing grounds were likely to present a health hazard considered the species, prevalence, abundance and geographical distributions of the parasites and their hosts, combined with results from monitoring. However, much of this data was lacking in 2010. Based on the limited information available then, it was concluded that there were no fishing grounds that were free of parasites and thus all wild caught seawater and freshwater fish were potentially infected and presented a risk of illness for consumers, especially if consumed raw.
To undertake a similar assessment for fishery products from aquaculture, data on the prevalence, abundance, species and geographical distributions of the parasites and their hosts were required, as well as information on the fish species and susceptibility to parasites, origin of the stock, production system, type of feed and feeding methods, time span for growth and processing method. Based on the information available in 2010, it was concluded that farmed Atlantic salmon reared in floating cages or onshore tanks and fed on pelleted feedstuffs were unlikely to be infected with anisakids and the risk of human infection with their larvae was considered to be negligible. Apart from farmed Atlantic salmon and anisakids, there were insufficient monitoring data for other farmed fish, and thus, it was not possible to identify other farmed fish species that did not present a health hazard with respect to the presence of parasites in related products.
1.3.2. Overview of parasites of public health importance in finfish from European waters (wild and aquaculture)
As reported in EFSA BIOHAZ Panel (2010), the zoonotic parasites of public health relevance in fishery products include species belonging to nematodes, trematodes and cestodes. Information on their life cycle and the distribution of the parasites in the fish body/muscles was included in that scientific opinion. An update of the information currently available on the parasites infecting fish sampled in European waters is included below.
1.3.2.1. Nematoda
The most relevant zoonotic nematodes include parasites belonging to the Anisakidae family, encompassing some species of the genera Anisakis, Phocanema 4 (recently resurrected genus to encompass six species formerly placed in genus Pseudoterranova) (see Bao et al., 2023) and Contracaecum. In the latter genus, only species of the C. osculatum (s. l.) complex maturing in pinnipeds are considered zoonotic.
These anisakid species share some general life cycle traits in that they all use planktonic or semi‐planktonic crustaceans as intermediate hosts and fish and/or squid (Anisakis) as paratenic/transport hosts carrying the infective larval stage (L3). Marine mammals, i.e. cetaceans (Anisakis) or pinnipeds, act as definitive hosts where the nematodes reach maturity and from which the eggs are released.
Concerning human zoonosis, the term anisakidosis is related to the disease caused generally by anisakids, while the term anisakiasis refers to the disease caused by Anisakis spp. Generally speaking, anisakidosis and anisakiasis are underestimated because they are misdiagnosed and there is no compulsory notification at EU level. An overview of human anisakidosis was also recently provided by Shamsi and Barton (2023).
Anisakis
: The two species A. simplex (s. s.) and A. pegreffii are the most common zoonotic nematodes associated with the consumption of raw or mildly thermally processed seafood. The L3 larval stages are mostly located in the visceral body cavity and outside the internal organs, but they are also found in the musculature of commercially important fish species (reviewed in Mattiucci et al., 2018; Shamsi & Barton, 2023) that are infected intra vitam and/or post‐mortem. The latter phenomenon is temperature dependant and occurs in the species A. pegreffii infecting anchovy (Engraulis encrasicholus) above 2°C (Cipriani et al., 2016). Storage temperature and time influence the post‐mortem motility of A. simplex (s. s.) larvae in herring (Clupea harengus) and blue whiting (Micromesistius poutassou) (Cipriani et al., 2024). Keeping the temperature at ≤2°C prevents larval post‐mortem migration into the flesh during fish storage, handling and transportation (Cipriani et al., 2024). Several studies have reported that the ventral (as compared to the dorsal) flesh is the most infected part of the fish (reviewed in Mattiucci et al., 2018; Levsen et al., 2018). Humans may become infected with Anisakis L3 larvae when they consume raw or undercooked fish, but these larvae do not develop to the adult stage. However, in a few cases, fourth‐stage larvae were isolated from human patients (Suzuki et al., 2021). When the larvae are viable and infective, the L3 of Anisakis penetrates the mucosal layers of gastric and/or the intestinal tract within a few hours of being ingested causing tissues damage. This acute gastrointestinal form of Anisakis infection represents an invasive disease (i.e. gastric anisakiasis (GA) or intestinal anisakiasis (IA)). The ulceration is accompanied by an eosinophilic inflammatory response with subsequent granuloma formation surrounding the penetrated larva. Clinical symptoms include severe stomach or abdominal pain, nausea, vomiting and intestinal obstruction, and mimic other common gastrointestinal diseases, such as acute appendicitis, peptic ulcer, etc. Basic diagnosis requires an anamnestic survey of the symptomatic patient and endoscopy, if possible, supported by serological diagnosis. Early removal and identification of the worm is essential to avoid the chronic infection developing into gastrointestinal eosinophilic granulomatosis.
The symptoms may also be complicated by a mild to strong allergic response (gastroallergic anisakiasis, GAA). It is clearly documented that ingestion of viable L3 larvae is required for the induction of allergic manifestations (Mattiucci et al., 2013). However, it is still unknown if an allergic reaction can occur because of exposure to allergens from dead parasites, e.g. present in canned or other heavily processed fishery products. Some allergens are relatively resistant to enzyme digestion or heat treatment and can trigger IgE reactions even when L3 larvae are killed by freezing and/or cooking of the fish (Carballeda‐Sangiao et al., 2014). Since allergens related to zoonotic parasites will not be specifically covered by the current assessment, this aspect will not be further discussed in this opinion.
The majority (72%) of human cases of illness caused by above‐mentioned species of Anisakis are GA, while 26% are IA cases, with 2% being extra‐gastrointestinal or ectopic anisakiasis (for a review, Mattiucci et al., 2018). A. simplex (s. s.) has been identified by molecular methods causing invasive gastric and IA (Arai et al., 2014; EFSA BIOHAZ Panel, 2010; Roca‐Geronès et al., 2020). Although associated with allergic anisakiasis, in some European countries, the aetiological agent has not been identified at species level (Daschner et al., 2021; EFSA BIOHAZ Panel, 2010; Mattiucci et al., 2018). Anisakis pegreffii causes GA, IA, GAA and ectopic anisakiasis, being identified in cases of invasive anisakiasis, detected by observation and molecular identification of the viable larvae during endoscopy (Arai et al., 2014; Lim et al., 2015; Mattiucci et al., 2011, 2013; Mattiucci, Colantoni, et al., 2017; Mattiucci, Paoletti, et al., 2017), colonoscopy (D'Amelio et al., 2023; Mattiucci, Paoletti, et al., 2017), or in surgically removed eosinophilic granulomas (Mattiucci et al., 2011; Mattiucci, Paoletti, et al., 2017; Mladineo et al., 2016). A. pegreffii infections have also been identified in Italy (D'Amelio et al., 2023; EFSA BIOHAZ Panel, 2010; Mattiucci et al., 2011, 2013; Mattiucci, Colantoni, et al., 2017), Croatia (Mladineo et al., 2016), South Korea (Lim et al., 2015) and Japan (Arai et al., 2014). A. simplex (s. s.) has been linked to invasive GA and IA in Japan (Arai et al., 2014; Auer et al., 2007; EFSA BIOHAZ Panel, 2010). The first case of human anisakiasis due to A. simplex (s. s.) occurring in Poland has recently been described (Kołodziejczyk et al., 2020). In Portugal, the first case was reported in 2017, but the larvae were only identified as Anisakis sp. (Carmo et al., 2017). Finally, records of human anisakiasis have been documented in recent years from Iran (Najjari et al., 2022), although it was not possible to identify the Anisakis species, despite the application of the real‐time PCR (qPCR) methodology. Similarly, the first case of human ectopic anisakiasis due to Anisakis sp. was recently described in Greece, by histological and molecular diagnosis (Dinas et al., 2024). It is still unclear if A. pegreffii and A. simplex (s. s.) differ in their pathogenic characteristics in human anisakiasis (Suzuki et al., 2010). The finding of Anisakis sp. larvae in patients suffering from gastric and/or colon carcinoma has also been reported (García Pérez et al., 2015), although the demonstration of potential tumour‐provoking capacity exerted by Anisakis spp. larvae has not yet been demonstrated.
All cases of human anisakiasis that were confirmed by molecular methods have been caused by infections with A. pegreffii or A. simplex (s. s.). However, one cannot exclude that other Anisakis species such as A. typica (s. l.) may act as aetiological agents of human anisakiasis in areas where these parasites commonly occur in food fish. For example, A. typica (s. l.) has been found in the muscle of commercially important fish species in the Indian ocean (Cipriani et al., 2022).
Phocanema : The Phocanema decipiens (s. l.) complex (see Bao et al., 2023; Paggi et al., 1991) consists of at least six sibling species: Ph. decipiens (s. s.), Ph. azarasi, Ph. cattani, Ph. krabbei, Ph. bulbosum and Ph. decipiens sp. E. As adults, they live in pinnipeds, with distinct gene pools and different geographical distribution (Mattiucci & Nascetti, 2008). So far, some of these species are reported as zoonotic. The first human case caused by these nematodes was described by Lee et al. (1985) in Korea, but the aetiological agent was not identified to species level by molecular markers. In Europe, in the recent years, cases caused by Ph. decipiens (s. s.) were identified by molecular markers in Italy (Cavallero et al., 2016), and in France (Brunet et al., 2017) most likely related to imported fish consumed raw or only lightly processed. A further infection due to Ph. decipiens (s. s.) was detected in the nasal cavity of a patient from Denmark with rhinitis symptoms (Nordholm et al., 2020). Live larvae of Ph. decipiens (s. l.) were also found in the pharynx, vomit or faeces in patients from Iceland in the years 2004 and 2005, after the consumption of fresh fish such as wolffish and cod (Skirnisson, 2022). A human case caused by Ph. azarasi was reported from Japan (Arizono et al., 2011). Several cases caused by Ph. decipiens (s. s.) from South Korea were reviewed by Song et al. (2022), while cases linked to the species Ph. cattani were described in Argentina (Menghi et al., 2020; Weitzel et al., 2015).
Contracaecum: Contracaecum osculatum (s. s.) identified both by morphology and molecular markers was found to infect pigs and elicit gastric eosinophilic granulomas suggesting the zoonotic potential of this anisakid (Strøm et al., 2015). Two cases of human infection, likely due to C. osculatum (s. l.), were reported from Japan (Nagasawa, 2012); however, no molecular identification was performed. Additionally, a human case of Contracaecum sp. infection acquired from eating locally caught fish was described in Australia (Shamsi & Butcher, 2011). All of these cases in Japan and Australia elicited milder symptoms compared to anisakiasis, including vomiting, gastric or intestinal pain, and in some cases, spontaneous expelling of larva in the stool (reviewed in Mattiucci, Cipriani, et al., 2017). The zoonotic role of Contracaecum species maturing to the adult stage in fish‐eating birds, e.g. C. rudolphii (s. l.), has not been demonstrated to date.
1.3.2.2. Trematoda
Zoonotic trematodes are present in the families Opisthorchiidae, Cyathocotylidae and Heterophyidae. Clonorchis sinensis and taxa belonging to the genera Opisthorchis, Metorchis and Pseudamphistomum (family Opisthorchidae) may cause cholangitis, choledocholithiasis, pancreatitis and cholangiocarcinoma (EFSA BIOHAZ Panel, 2010; Kondrashin et al., 2023). Their prevalence in wild fish ranges from less than 1% to greater than 90%, depending on the region of the world. It is noteworthy that human cases caused by species within these three genera occur sporadically in various parts of Italy (Pozio et al., 2013, and more recent cases) and Russia (Kondrashin et al., 2023). The metacercariae of Paracoenogonimus ovatus (family Cyathocotylidae) commonly occur in the flesh of several wild freshwater fish species including various cyprinids and pike (Esox lucius) in central and south‐eastern Europe. The zoonotic ability of P. ovatus has been demonstrated experimentally in infection trials using laboratory rats which showed intestinal lesions that were causatively linked to the infection (Goncharov & Soroka, 2016).
The metacercariae of the heterophyid species Cryptocotyle lingua, inducing black spot disease in fish, commonly occur in many finfish species from the temperate and subarctic seas of the NE Atlantic (Duflot et al., 2023). C. lingua develops to maturity in fish‐eating birds but also in some terrestrial mammalian species, while periwinkles (Littorina spp.) act as first the intermediate host from which the fish‐infective cercaria stage emerge. Human infections with C. lingua have been reported from Greenland and Alaska (Babbott et al., 1961; Rausch et al., 1967). Marine cage‐reared fish are at risk of infection with C. lingua metacercariae if the cages are sufficiently close to the shoreline. Some C. lingua cercariae may penetrate the skin of the fish host and encyst in the superficial layers of the fillets, thus posing a zoonotic risk if infected fillets are consumed raw or only lightly processed.
1.3.2.3. Cestoda
Cestodes of the genus Dibothriocephalus (syn. Diphyllobothrium) include at least 13 species that infect humans. These cestodes are widely distributed in fish, mammal and bird hosts (EFSA BIOHAZ Panel, 2010; Gustinelli et al., 2016) and are generally associated with intermediate and definitive hosts living in temperate or cold‐water habitats. In recent years, several reports have suggested an expansion of the geographic area of distribution of this genus, due to host switching events (Kuchta et al., 2019). The broad fish tapeworm Dibothriocephalus latus has been considered the principal species infecting humans in Europe (Kuchta et al., 2015). Human diphyllobothriosis due to D. latus has reemerged in the subalpine region of Italy (Lago Maggiore and Lago di Como), Lake Geneva and from France while it shows a decrease in other historical endemic areas, such as the Baltic countries (Kuchta et al., 2015). Raw fillets of perch (Perca fluviatilis) represent the major source of infection. Sporadic cases of human diphyllobothriosis have also been reported from some countries of central and western Europe, which have been considered free of the parasite. These cases seem to be due to the consumption of imported fish. This is the case of D. pacificum and D. nikonkaiensise from Pacific region (reviewed in Kuchta et al., 2015). The occurrence of the broad fish tapeworm, D. latus, in, e.g. wild European perch (Perca fluviatilis) is well documented (e.g. Gustinelli et al., 2016; Králová‐Hromadová et al., 2021), and even non‐endemic areas, such as Spain, report infections in fish (Esteban et al., 2014). The older literature suggested widespread infections of wild fish in the Danube River. However, recent studies on European perch from the river did not detect this parasite, and it was suggested that the infection was no longer as widespread as previously considered (Radačovská et al., 2019).
In recent years, the occurrence of larval cestodes (Trypanorhyncha) of the genera Molicola and Gymnorhynchus has been reported in the edible parts (muscle) of fish of commercial importance (i.e. scabbard fish, Lepidopus caudatus) (Palomba, Marchiori, et al., 2023). These larval parasites are maturing to the adult stage in elasmobranchs in European waters. Although these larval parasites are harmless to humans, a heavy infection could negatively impact the perceived quality of seafood in particular due to the visible larval stages of trypanorhynch species in the edible parts of infected fish. Indeed, their occurrence has been also documented in RASFF notifications (see Figure 1). There is also speculation that Trypanorhyncha larvae, i.e. Gymnorhynchus gigas and Molicola horridus, infecting the fish host musculature, could release proteins that might trigger allergic reactions in humans (Gòmez‐Morales et al., 2008). However, these proteins have not yet been identified in marine metacestodes.
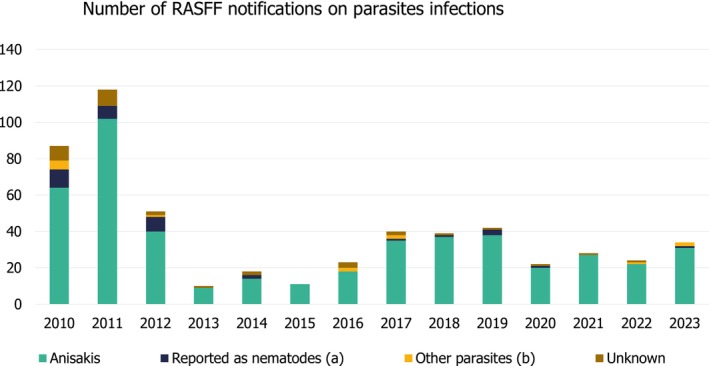
Number of RASFF notifications of parasite infections in fish and fishery products from 2010 to end of 2023. (a) Includes parasites reported as nematodes without further specifying genus and species (i.e. Pseudoterranova). (b) Includes parasites other than nematodes (i.e., Trypanorhyncha, Opisthorchis, Acanthocephala, etc.). Unknown: notifications from unknown parasitic infections. Source: Graphic built by EFSA using data provided by RASFF (Annex B, last update on January 30 2024, data included until December 2023).
1.3.2.4. Acanthocephala
Acanthocephalans, using marine mammals, particularly whales, as the definitive hosts, use various fish species as paratenic hosts, in which the infective larval stage resides. Genera Bolbosoma and Corynosoma include species which are zoonotic. Two species within the genus Bolbosoma (B. capitatum and C. nipponicum) have caused human infections involving severe clinical symptoms. The cases, all in Japan, were all associated with prior ingestion of fish products (e.g. sashimi) (Mathison et al., 2021).
The genus Corynosoma, using primarily seals (pinnipeds) and to some extent whales (cetaceans) as definitive hosts, comprises two species, C. villosum and C. validum, which have been connected to human infections. Ingestion of undercooked fish products was suspected to be the source of infection (Mathison et al., 2021). Since various species of Corynosoma occur in wild populations of e.g. Atlantic cod (Setyawan et al., 2020), the zoonotic potential of European species cannot be excluded. However, no records from aquacultured fish infected with this parasite genus exist.
1.3.2.5. Cnidaria (Myxozoa)
At least two species within the myxosporidian genus Kudoa, i.e. K. thyrsites and K. islandica, are of concern to the European fishery and aquaculture industries since they may induce ‘soft flesh’ which is a rapid and severe post‐mortem enzymatic degradation of the fish body musculature. Both species can infect commercially important wild fish species such as Atlantic mackerel (Scomber scombrus) and wolffish (Anarhichas spp.) in NE Atlantic waters, respectively (Giulietti et al., 2022; Kristmundsson & Freeman, 2014). Kudoa thyrsites has been identified as the causative agent of ‘soft flesh’ in farmed Atlantic salmon in the Pacific, inflicting significant losses for the industry. There have only been two reports of K. thyrsites in farmed Atlantic salmon from Spain and Ireland (Barja & Toranzo, 1993; Palmer, 1994). The parasite has not been identified from other farmed fish species in the EU/EFTA. This may change, however, since climate‐related alterations in the ecosystem may facilitate the spread of K. thyrsites into northern European aquaculture systems.
Two Kudoa species, K. septempunctata and K. hexapunctata, have been identified as the causative agents of food poisoning following consumption of raw farmed or wild fish in Japan and Korea (Kang et al., 2020; Kawai et al., 2012; Sung et al., 2023; Suzuki et al., 2015). Other species may be involved in hitherto unclarified episodes of adverse reactions after raw fish consumption. Hence, in a Spanish study, anti‐Kudoa sp. antibodies were detected in persons showing allergic reactions after fish consumption (Martínez de Velasco et al., 2007). Although neither K. thyrsites nor K. islandica seem to cause food poisoning in humans, a hypersensitisation to the parasites cannot be ruled out.
1.3.2.6. Contamination of fish with other parasites
Occasionally, protozoan parasites that are not naturally infecting fish may contaminate fishery products. For example, the oocyst of Cryptosporidium, Toxoplasma and Blastocystis species, originating from terrestrial vertebrates, have been detected in oysters, mussels and marine coastal fish (Aco‐Alburqueque et al., 2022; Moratal et al., 2022; Santoro et al., 2020; Tedde et al., 2019). Oocysts of these parasites are washed by rainfall from the anthropogenically or livestock‐contaminated terrestrial areas, or sewage water and are accidentally retained by bivalves or fish species that feed by filtering seawater for plankton and microorganisms. Moreover, farmed seabass can be infected with the zoonotic species Cryptosporidium ubiquitum, thus representing a potential risk for consumers (Moratal et al., 2022). However, its typical infection site in fish, i.e. the gastrointestinal tract, is normally not consumed which largely prevents human infections. However, the possible risk of infection during the fish cleaning and food processing cannot be excluded. As stated in the interpretation of ToRs (Section 1.2), these parasites will not be considered in this assessment.
1.3.2.7. Parasite virulence and pathogenic factors
In general, helminths pathogenic factors encompass a rich repertoire of molecules that are secreted/excreted by the parasite and that play a role in the host–parasite interaction, both in natural and accidental (human) hosts. Recent progress using omic methodologies have provided new information on the virulence and pathogenic repertoire of fish zoonotic parasites. It has been demonstrated that the L3 of A. pegreffii secrete these factors, either as free proteins in the secretome (Trumbić et al., 2021), or packaged in form of extracellular vesicles (EVs) (Boysen et al., 2020;Cavallero et al., 2022; Palomba, Rughetti, et al., 2023). These products have a role in the larval invasion mechanism and the immunomodulation of the host's response, in particular the evasion from the immune system. It has been suggested that the pathogenicity of A. pegreffii L3 is likely the result of the direct invasive capability of the larva, its released excretory secretory products (ESPs) (Trumbić et al., 2021), including those transported by EVs (Cavallero et al., 2022; Palomba, Rughetti, et al., 2023), as well as the intricate interplay of the host's immune response (Mehrdana et al., 2017). Among ESPs an array of peptidases and peptidase inhibitors are considered essential for larval moulting, feeding, tissue penetration and migration (Mladineo et al., 2023; Palomba, Rughetti, et al., 2023).
1.3.3. RASFF notifications of zoonotic parasites in fish and fishery products
From 2010 to the end of 2023, there were 544 notifications of infection of the fish/fishery products reported by 21 EU Member States to the Rapid Alert System for Food and Feed (RASFF, 5 see Section 2.1.1, last access on January 2024, Annex B) (Figure 1). With 274 notifications, Italy was the Member State that had the most notifications (50.37% of the total), followed by Spain with 113 notifications (20.77% of the total), France 29 (5.33%) and Greece with 28 (5.15%). The origin of the notified products included Spain (114 cases), Morocco (95 cases), France (92 cases) and Norway (18 cases). In addition, 29 cases originated from New Zealand, of which 21 were notified by Greece in 2011 due to a parasitic infection with Anisakis of frozen squids. Anisakis was the parasite reported in most (85%) of the notifications. In total, 95.59% of the notifications were attributed to ‘Fish and fishery products’, but a noteworthy 4.04% was assigned to ‘Cephalopods and products thereof’ and 0.38% to ‘Bivalve molluscs and products thereof’. The most reported fish species were hake (20.22% of the total 544 notifications), mackerel (18%), monkfish/angler (13.60%), scabbardfish (6.43%) and codfish (5.70%). These five species together account for almost two‐thirds of the total number of the notifications.
It is worth noting that of these only cod is considered an important farmed fish species, based on production and consumption volumes in the EU, in this opinion (see Section 3.1.1). These notifications do not include information on whether the product originated from wild fisheries or aquaculture production, so the infected cod may have been from wild caught fish. More information on the notifications from 2001 to 2020 can be found in Caldeira et al. (2021).
The parasite notifications for imported fishery products since 2016 for the EU reported to GLOBEFISH 6 (see data Section 2.1.2) are shown in Figure 2. In 2022, 11 cases of parasite notifications in imported fish were recorded, including 10 due to Anisakis, mainly detected in scabbard fish and scorpion fish, and one unidentified parasite in red mullet.
1.3.4. Human outbreaks and related human cases caused by anisakid nematodes in the EU
According to the data reported by the Member States to the EFSA zoonoses and food‐borne outbreaks database (see data Section 2.1.3), 11 food‐borne human outbreaks caused by unspecified Anisakis spp. were reported in the EU from 2010 to 2022. One occurred in France in 2010, and the other 10 in Spain in 2014 (1), 2015 (2), 2016 (2), 2018 (3) and 2020 (2). No outbreaks were reported to EFSA in 2021 or 2022 (EFSA and ECDC, 2023). Altogether, there were 45 cases of illnesses, with two cases where patients were hospitalised, and no deaths reported (Figure 3). Four outbreaks were reported with strong evidence regarding the food vehicle, which was ‘fish and fishery products’.
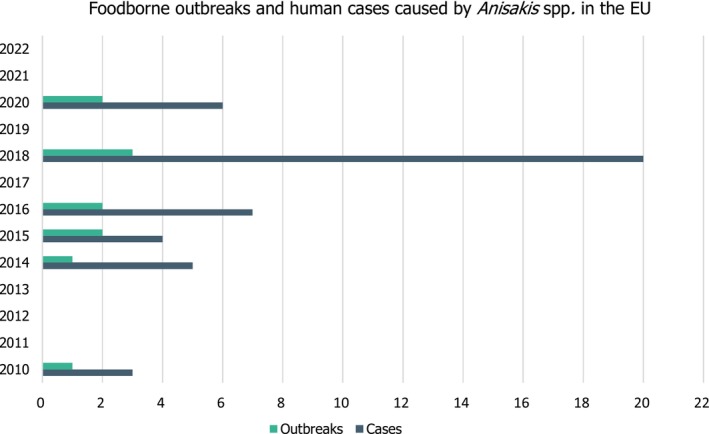
Food‐borne outbreaks and related human cases caused by Anisakis spp. (unspecified) reported to the EFSA zoonoses and food‐borne outbreaks database. One outbreak occurred in France (2010) and the rest occurred in Spain over the years (2014–2020). Source: EFSA zoonoses and foodborne outbreaks database (Annex C, last update January 2024).
Additionally, several (>50) cases of illness caused by A. pegreffii have been reported in Italy (reviewed in Mattiucci et al., 2018; Guardone et al., 2018; D'Amelio et al., 2023) since 1999. In Portugal, there were three recent cases reported in 2017 (2), and in 2018 (1) (for a summary, see Santos et al., 2022). Overall, the occurrence of human anisakiasis is underestimated due to underreporting and issues with diagnosis.
1.3.5. Ecological drivers of the infection with zoonotic parasites in fish
The distribution of the zoonotic helminth parasites is the results of both biotic (hosts involved in their life cycle) and abiotic factors.
Helminth parasites, such as opistorchid trematodes, have a patchy distribution within their geographical range and are associated with specific wetlands. The zoonotic parasite Opistorchis felineus, for example, is present in European freshwater fish and its bithyniids first intermediate snail hosts have been reported to be confined to slowly flowing shallow waters and littoral zones of large bodies of water, such as lakes. The potential for expansion of the range of this parasite, as well as the zoonotic species of Metorchis, is linked to the presence of suitable snail and fish hosts in the same area. These parasites use both wild and domestic carnivore species as definitive hosts (Sithithaworn et al., 2015).
Even the existence of local foci of diphyllobothriosis infection depends on the presence of the first suitable intermediate host (copepods), the long‐term survival of the larval stage (plerocercoids) in wild fish, as well as the large spectrum of nonhuman definitive hosts (reservoirs) (Kuchta et al., 2015).
As for anisakid nematodes, successful transmission requires a wide availability of suitable definitive and intermediate hosts, with a sufficient population size, and stable marine trophic chains that allow the parasites to complete their life cycle. When conditions are favourable, a large parasite population can be maintained in fishing grounds (for a review see Mattiucci et al., 2018; Palomba, Marchiori, et al., 2023).
Among the ecological drivers, the following factors should be also considered: (i) temperature, salinity and oceanographic conditions as these affect the hatching of eggs and the survival and dispersion of the first larval stages of anisakids; (ii) the fishing ground (the origin and migratory routes) of certain fish species can often explain the differences not only in the distribution of Anisakis spp., but also of their infection levels (expressed as prevalence, mean abundance and/or mean intensity, according to Bush et al. (1997), see 1.2) in the same fish species when sampled from different fishing areas; (iii) the fish length/size (several different fish species have shown a strong positive correlation between fish size/length and Anisakis spp. prevalence and/or abundance values); and (iv) the site of infection in the fish and cephalopod hosts. The localisation of anisakids in different hosts tissues may vary for different parasites species. For example, different rates of infection by A. pegreffii and A. simplex (s. s.) of the fish host's musculature (mainly the ventral belly flap) were recorded (Levsen et al., 2018; Mattiucci et al., 2018). The L3 of A. typica (s. l.) has been also observed in fish musculature as intra‐vitam infection in its suitable fish hosts (Cipriani et al., 2022). Conversely, A. physeteris has never been found in the fish musculature. It has also been observed that the differential site of infection with anisakid species appear dependent not only on the parasite species, but also on the fish host species (Cipriani et al., 2024; Levsen et al., 2018; Mattiucci et al., 2018; Mladineo & Poljak, 2014).
Possible impact of climate change on parasite diversity and distribution in European aquaculture systems
In general, the currently observed or expected rise in water temperature, especially in northern seas (MedECC, 2020), may increase parasite distribution, prevalence and transmission. For example, the hatching success of eggs and the survival of free larvae of Anisakis simplex (s. l.) can be achieved from 12°C to 19°C (Gomes et al., 2023; Højgaard, 1998; Mladineo et al., 2023). Such a temperature range may prevail over longer periods during the year and over larger geographical areas in the North‐Atlantic as a result of climate change, thus facilitating the presence of relatively higher numbers of free and infective Anisakis larvae and for longer periods of time. The ongoing or expected northward spread of plankton and fish intermediate or paratenic hosts of A. simplex (s. l.), such as the copepod Calanus finmarchicus and Atlantic mackerel (Stenevik & Sundby, 2007), along with a prolonged migration season of cetaceans that feed on them (Evans & Bjørge, 2013), may also promote the presence of Anisakis larvae in the vicinity of coastal farming facilities in the NE‐Atlantic (Norway, Iceland, Faeroes, Scotland, Ireland).
Recent findings indicate a potential northbound expansion of the geographical range of A. pegreffii into NE Atlantic waters. Previously limited to the northern part of the Atlantic Spanish coast, recent findings of its larval stages in the Atlantic cod (Gay et al., 2018), the Atlantic mackerel (Levsen et al., 2018) and in European hake (Diez et al., 2022) seems to indicate a northward expansion of the parasite. This suggests that the species may complete at least parts of its life cycle in these areas. This hypothesis is supported by in vitro observations of A. pegreffii eggs hatching achieved at 19°C (Mladineo et al., 2023; Moratal et al., 2023) suggesting the ongoing rising sea temperature in NE Atlantic waters could permit the completion of the A. pegreffii life cycle in those regions.
Other anisakid species have recently expanded their range of distribution, most likely as a consequence of changes in sea temperature. For example, A. typica sp. A has shown increased prevalence values in the eastern Mediterranean basin and in the Central Mediterranean (off the Malta coast) in co‐infection with A. pegreffii and A. physeteris (Palomba, Marchiori, et al., 2023). Previous molecular epidemiological studies had failed to detect this parasite in these locations (Mattiucci et al., 2018). Considering the pelagic/mesopelagic life cycle of this parasite species and its capacity to infect fish muscle, we cannot exclude the possibility of the higher probability of infection in fish in Mediterranean waters in the future and the possibility of accidental transmission to farmed fish species in southern European countries (Palomba, Marchiori, et al., 2023).
A recent review of the effect of long‐term changes on the abundance of Anisakis spp. and Phocanema spp. suggested an increase in the abundance of the former over a 53‐year period (1962–2015). This was attributed to a range of factors including long‐term climate change that affected the host susceptibility to infection by compromising the ability of fish to immunologically or behaviourally resist infection, or that facilitates faster growth and shorter generation times in aquatic parasites (Fiorenza et al., 2020).
Other epidemiological drivers which could affect the dissemination of the fish‐borne diseases especially related to anisakids, include the changed habits of fish consumption. People more often consume raw or undercooked foods. At the same time, there is an increasing interest in healthy dietary regimes that has driven increased fish consumption, resulting in more aquaculture products being imported into Europe, including from areas with a high prevalence of zoonotic parasites (Broglia & Kapel, 2011).
2. DATA AND METHODOLOGIES
2.1. Data
Since the EFSA BIOHAZ Panel (2010) scientific opinion on risk assessment of parasites in fishery products, there have been changes in EU membership. For this opinion, when referring to/presenting EU data, data from Croatia were considered from 1 July 2013 onwards, when the country joined the EU, and UK data were considered until 31 January 2020, when the UK left the EU. Consequently, from 1 February 2020, the EU acronym refers to the current 27 Member States, and the UK data are presented separately.
2.1.1. Rapid Alert System for Food and Feed (RASFF)
The EC Rapid Alert System for Food and Feed (RASFF)5 allows for the exchange of information among EU Member State food safety authorities on health risks derived from potential non‐compliant food or animal feed (legal framework, Article 50 of Regulation (EC) n. 178/2002, implementation established in Commission Implementing Regulation (EU) 2019/1715 of 30 September 2019 laying down rules for the functioning of the information management system for official controls and its system components, the IMSOC Regulation). It operates through the iRASFF online platform. Data on the notifications received in EC SANTE RASFF on ‘parasitic infestations’ from 01 January 2010 to 31 December 2023 were provided by colleagues of the RASFF Team (Food Hygiene, Feed and Fraud, EC DG‐SANTE, Unit G.5) to EFSA for the current opinion (Annex B).
2.1.2. FAO: Globefish
GLOBEFISH6 is the FAO Fisheries Division‐funded project responsible for providing up‐to‐date trade and market information on fish and fishery products. Based on the RASFF Portal as source, GLOBEFISH summarises annually the causes of import notifications, such as chemical, microbiological or parasitic, etc.
2.1.3. Data on aquaculture production and fish consumption
2.1.3.1. Data on aquaculture production
The sources used for the data included in Section 3.1.1 and Table B.1 (Annex B) were taken from:
Eurostat 7 is the statistical office of the European Union. Eurostat statistics on fisheries 8 contain data for the EU Member States and the UK (until January 2020), Iceland and Norway on catches of fish products made by vessels in fishing regions; aquaculture production (covers the farming of aquatic organisms such as finfish, molluscs, crustaceans, algae, etc., taking place in both inland and marine areas, and includes also as organic aquaculture 9 ); landings of fishery products in ports; and fishing fleet.
EUMOFA 10 is the European Market Observatory for Fisheries and Aquaculture developed by the European Commission. EUMOFA collects daily data from EU countries, Iceland, Norway, UK and EU institutions on volumes, values and prices of fishery and aquaculture products, from the first sale to retail stage, including imports and exports. Weekly data originate from the European Commission, while monthly data originate from EUROSTAT – COMEXT. They produced yearly the Fish Market reports 11 (EC DG‐MARE, 2023). Both the database data and the cited report were used for the current scientific opinion.
Data on aquaculture for Norway were obtained from the Norwegian Directorate of Fisheries 12 and its report ‘Key figures from Norwegian Aquaculture Industry 2021’ (Fiskeridirektoratet, 2022), which serves as the Ministry of Trade, Industry and Fisheries' advisory and executive body in matters pertaining to fishing and the management of aquaculture.
Data for Iceland were collected from Statistics Iceland, the centre for official statistics in Iceland. 13
Finally, data for the UK were obtained from ‘UK fishing statistics’, House of Commons Library (Uberoi et al., 2022), from the Organisation for Economic Co‐operation and Development (OECD) 14 and from Seafish 15 (public body sponsored by the Department for Environment, Food and Rural Affairs, Defra, working also with the Scottish, Welsh and Northern Irish administrations).
2.1.3.2. Data on fish consumption in the EU
The sources used for the data included in Section 3.1.1 and Table B.1 (Annex B) were taken from:
EUMOFA (see above).
EFSA Comprehensive Food Consumption Database 16 is a source of information on food consumption across the EU. It contains detailed data provided by competent authorities of Member States, the UK and EU pre‐accession countries from the most recent national dietary surveys in those countries, at the level of consumption by the individual consumer. In the database, dietary surveys and food consumption data for each country are divided by category. Data from this database on fish consumption were extracted and considered for the current opinion (filtering at level L1 for ‘Fish, seafood, amphibians, reptiles and invertebrates’, down to L7 exact type of fish/product, Annex D).
2.1.4. The European Union One Health Zoonoses report
The EU system for the monitoring and collection of information on zoonoses is based on Directive 2003/99/EC, which obliges EU Member States to collect relevant and, when applicable, comparable data on zoonoses, zoonotic agents, antimicrobial resistance and food‐borne outbreaks. In accordance with the zoonoses directive, EFSA examines the national reports and data submitted by the EU Member States regarding their zoonoses monitoring activities and publish an EU Summary Report on the trends and sources of zoonoses and zoonotic agents in the EU (EFSA and ECDC, 2023). Data on Anisakis reported by the Member States to the EFSA zoonoses and food‐borne outbreaks database were extracted from 2010 to end of 2023 for the current opinion (Annex C).
2.1.5. Data from EU and national research projects
PARASITE: ‘Parasite risk assessment with integrated tools in EU fish production value chains’ 17 (EC FP7‐KBBE no. 312068, 2013–2016). It provided information on the epidemiology of zoonotic parasites in the most economically important fish species or stocks from major European fishing grounds (Levsen et al., 2018), an overview of methods for the detection of anisakids in fish samples (Levsen et al., 2018), and the identification to the species level by innovative molecular methods (Mattiucci et al., 2016, 2019; Paoletti et al., 2018). It also provided technological tools for the mitigation of the risk posed by anisakids in fishery products, methods to assess viability and infectivity of anisakids under different treatments and conditions and information on treatments for the inactivation of anisakids. This and some other information generated within the project were considered for the current opinion.
ParaFishControl ‘Advanced tools and research strategies for parasite control in European farmed fish’ 18 (EU H2020, 2015–2020). Information generated from an observational survey on the presence of Anisakidae zoonotic parasites in the most economically relevant farmed marine fish species other than Atlantic salmon in European countries (Fioravanti et al., 2021) was considered for the current opinion.
APROMAR 19 (2009–2011), ‘Presence of nematodes of the genus Anisakis evaluation in the Spanish marine aquacultured fish’ (founded by the Spanish Ministry of the Environment and Rural and Marine affairs, and by the EC European Fishery Fund). Information from an epidemiological study on the prevalence and intensity of Anisakis in Spanish aquaculture gilthead seabream, European seabass, turbot and meagre (APROMAR, 2012) was considered for the current opinion.
2.2. Methodologies
2.2.1. Approach to answer the ToRs
The approach to answer the ToRs was defined in advance and is described in the protocol (Annex A). It covers both the problem formulation (i.e. what the assessment aims to address) and which methods will be used for addressing the problem. The problem formulation (‘what’) includes the clarification of the mandate (see further refined in Section 1.2) and consists of the steps (1) translation of the mandate into scientifically answerable AQs, and (2) the selection of the approach for the assessment. The planning of the methods for conducting the assessment (‘how’) consists of specifying the evidence needs and the methods for answering each AQ, including the uncertainty analysis. Protocol development followed the draft framework for protocol development for EFSA's scientific assessments (EFSA Scientific Committee, 2023).
2.2.2. Literature search and expert knowledge
A qualitative assessment was undertaken, based on the available literature and expert knowledge within the WG as detailed in the protocol (Annex A). The search strategy (search strings and databases) is included in Annex E (E1‐E2). Literature searches were extended using ‘footnote chasing’ (White et al., 1992) and supplemented by citation inputs by WG members and information about relevant publications provided by members of the EFSA BIOHAZ Panel. The relevance of the records in providing information was assessed by screening the title, keywords and the abstract and based on the knowledge and expertise of the WG members. This review included international reports and EFSA scientific opinions and reports, scientific review papers, book chapters, peer‐review papers and other documents known by the experts or retrieved through non‐systematic searches. Current European Legislation and ISO standards were also considered.
Additionally, a systematic review conducted within the ‘Pathogens in foods database: extension with Vibrio and parasites’ EFSA's grant agreement (GP/EFSA/BIOHAW/2023/05 20 ) was used to complement the data collected from the searches mentioned above as described in Annex E (E3). Protocol followed and extracted data for the occurrence of anisakids in EU/EFTA aquaculture are available at https://doi.org/10.5281/zenodo.10790873. These data will be incorporated in the ‘Pathogens in Food (PIF) database (see Appendix 3) accessible through a web application (https://pif.esa.ipb.pt/).
2.2.3. Uncertainty analysis
As recommended by the EFSA guidance and related principles and methods on uncertainty analysis in scientific assessments (EFSA Scientific Committee, 2018a, 2018b), an uncertainty analysis was undertaken as described in the protocol (Annex A), the sources of the main uncertainties related to ToR1 were identified by the experts in the WG and their impact on the uncertainty of the answers to the AQ1, were discussed. Consensus expert judgement within the WG, informed by the collected evidence and expert knowledge, was used to assess the certainty of the answers to the AQ1, which was expressed using EFSA's subjective probability scale when appropriate (EFSA Scientific Committee, 2018a).
3. ASSESSMENT
3.1. ToR1: The occurrence of parasites of public health importance in fishery products derived from the most relevant farmed fish species in the EU (in particular, but not limited to, Atlantic salmon, seabass, seabream and turbot)
AQ1: What is the occurrence of parasites of public health importance in fishery products derived from the most relevant farmed finfish species in the EU/EFTA?
This section addresses the occurrence of parasites of public health importance in fishery products derived from the most relevant farmed finfish species in the EU/EFTA. The relevant farmed finfish species (considering data on production and consumption) and the respective farming practices will be described before linking each fish species with specific parasites.
3.1.1. Farmed fish species in the EU/EFTA/Faroe Islands/UK
SQ1.1: Which are the most relevant farmed fish species produced in the EU/EFTA, in addition to Atlantic salmon, seabass, seabream and turbot that may be infected with parasites of public health importance?
ToR1 of this opinion covers aquaculture within the EU, but also EFTA countries, among which Norway and Iceland are the main producers. Production data from the Faroe Islands and United Kingdom (UK) are also relevant as both are major exporters of farmed finfish into the EU (EC DG‐MARE, 2023) and were also considered. Fish farmed outside these European areas/countries are excluded.
3.1.1.1. EU/EFTA/Faroe Islands/UK aquaculture production
According to Eurostat data, between 2010 and 2021, the volume of EU aquaculture production remained relatively stable. Aquaculture farming in the EU (Figure 4) yielded 1.1 million tonnes of aquatic organisms in 2021 (corresponding to one quarter of the output of European fisheries as a whole), worth €4.2 billion.
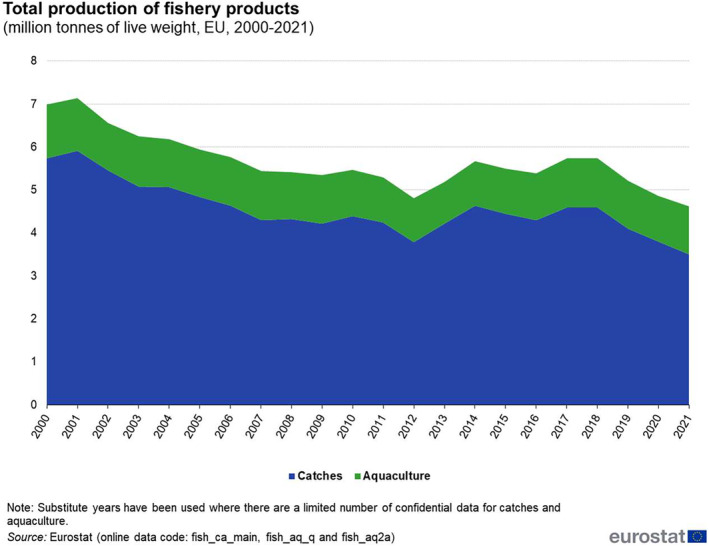
Total production of fishery products in the EU (2000–2021, in million tonnes of live weight). Source: Figure taken from Eurostat. https://ec.europa.eu/eurostat/statistics‐explained/index.php?title=Aquaculture_statistics
Despite the great diversity of farmed aquatic species in the world (~500 taxonomically recognised species of which 313 are finfish species [FAO, 2022]), only a small number of species dominate EU aquaculture production, being ~
49% crustaceans and molluscs, 19% salmonids, 22% marine fish and 10% freshwater fish (ECA, 2023).
The main farmed finfish species are trout (mainly rainbow trout, Oncorhynchus mykiss), gilthead seabream (Sparus aurata), European seabass (Dicentrarchus labrax), carp (mainly common carp, Cyprinus carpio), bluefin tuna (Atlantic bluefin tuna, Thunnus thynnus), salmon (Atlantic salmon, Salmo salar) and turbot (Scophthalmus maximus) (Figures 5, ,6;6; additional data for the different fish species are shown in Appendix A, Table A.1). The main EU finfish producers are Greece, Spain, Italy and France (EC DG‐MARE, 2023).
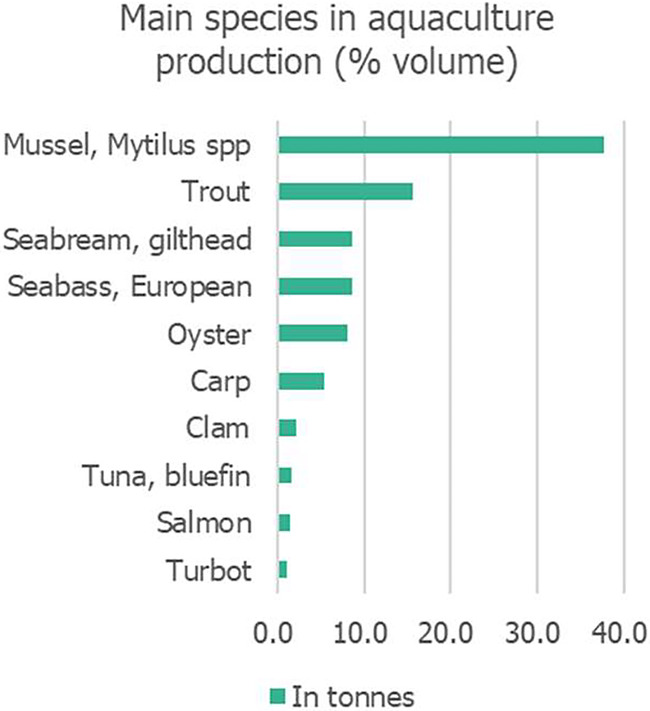
Main species in EU aquaculture production in 2021 (percentage of volume in tonnes). Source: Graphic built by EFSA based on EUROSTAT data (last access February 2024): https://ec.europa.eu/eurostat/statistics‐explained/index.php?title=Aquaculture_statistics#EU_Aquaculture.
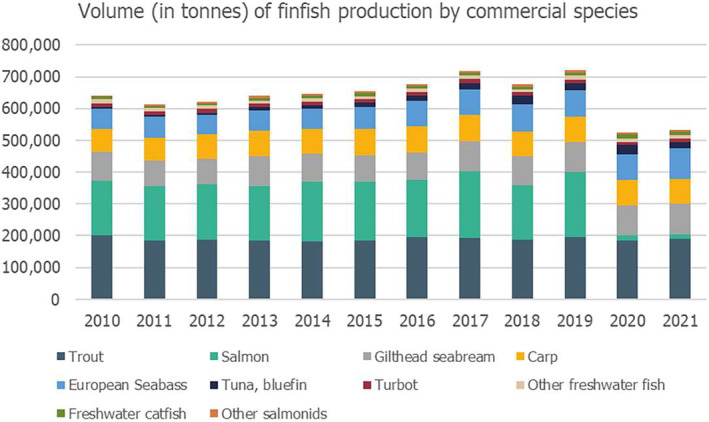
Volume (in tonnes) of aquaculture production by main commercial finfish species, in the EU, for the period 2010–2021. Note: UK data were not included in 2020 and 2021 as the UK had left the EU and this accounts for the decrease in salmon production. Source: Graphic was built by EFSA using data available at EUMOFA (https://eumofa.eu/data#aquacultureTab last access February 2024).
The production data for different fish species, according to the EU Fish market report 2023 (EC DG‐MARE, 2023), are provided in Appendix A, Table A.1.
Norway: Between 2010 and 2021, there was a sharp increase in the volume of Norwegian aquaculture production. The total sales volume of farmed fish in Norway reached 1662 million tonnes in 2021, making Norway the largest fish producer in Europe (the value of aquaculture output in Norway exceeding that of the whole of the EU, with Atlantic salmon alone accounting for 93.9% of the total sales volume followed by rainbow trout with 5.7%).12 Atlantic cod and Atlantic halibut are currently intensively farmed in Norway with a total production volume reaching 4340 tonnes in 2021 (Appendix A, Table A.1).
In Iceland, the total production of farmed fish was 53,000 tonnes in 2021, 21 with Atlantic salmon comprising 90% of all fish farming (Appendix A, Table A.1). The farming of arctic char and rainbow trout was roughly 5000 and 1000 tonnes in 2021, respectively.
The total aquaculture production in Faroe Islands was roughly 95,000 tonnes in 2021, consisting entirely of Atlantic salmon 22 (Appendix A, Table A.1).
British aquaculture: Aquaculture production stands at 230,000 tonnes in 2021, being an important part of the UK seafood industry. 23 This production is dominated by Atlantic salmon (mainly farmed in Scotland [Uberoi et al., 2022]), followed by marine molluscs and rainbow trout, while many other species are farmed in small volume 24 (Appendix A, Table A.1).
3.1.1.2. EU consumption of fishery products
The mean per capita annual consumption of fishery products in the EU is ~24 kg with one quarter of this (~
1.25 million tonnes) being supplied by aquaculture. Tuna (all species combined, mostly wild), Atlantic salmon (mostly farmed), cod (mostly wild) and Alaska pollock (wild) were the four most consumed fishes in the EU in 2021 according to EUMOFA (EC DG‐MARE, 2023) (Table 1, Appendix A, Table A.1). In 2021, Portugal (56.52 kg live weight/capita/year) was the major consumer of fishery and aquaculture products in the EU, followed by Spain (43.0 kg live weight/capita/year) and France, Luxembourg and Italy (32.2, 31.4 and 30.2 kg live weight/capita/year, respectively) (EC DG‐MARE, 2023) (Table 1).
TABLE 1
Apparent consumption of most consumed fishery and aquaculture finfish in the EU (2021).
Products | Per capita consumption (kg, LWE) | Increase or decrease in 2021 as compared to 2020 | % wild | % farmed |
---|---|---|---|---|
Tuna | 2.86 | −6% | 99.4% | 0.6% |
Salmon | 2.60 | 77% | 5.6% | 94.4% |
Cod | 1.75 | 2% | 99.9% | 0.1% |
Alaska pollock | 1.68 | −3% | 100% | 0% |
Hake | 1.02 | −1% | 100% | 0% |
Herring | 1.00 | −9% | 100% | 0% |
Sardine | 0.54 | −3% | 100% | 0% |
Mackerel | 0.53 | −11% | 100% | 0% |
Trout | 0.49 | 0.3% | 1.6% | 98.4% |
Saithe (=Coalfish) | 0.36 | 2% | 100% | 0% |
Other products | 6.29 | 5% | 73.8% | 26.2% |
Total | 23.71 | 2% | 71.3% | 28.7% |
Abbreviation: LWE, live weight equivalent.
Source: Adapted by EFSA from Table 10 of the EU Fish Market report, 2023 Edition (EC DG‐MARE, 2023).
Additional data on specific fish consumption can be found in the EFSA Comprehensive European Food Consumption Database (see data Section 2.1.4 and Annex D) and in EUMOFA section EU fish market country profile (EC DG‐MARE, 2023).
3.1.1.3. Most relevant farmed fish species
Based on the information available on production and consumption shown above and in Annex B (Table B1), the farmed finfish species 25 considered most relevant and that will be considered in the current opinion are the following:
For marine aquaculture: Atlantic salmon Salmo salar Linnaeus, 1758; rainbow trout Oncorhynchus mykiss (Walbaum, 1792); gilthead seabream Sparus aurata Linnaeus, 1758; European seabass Dicentrarchus labrax (Linnaeus, 1758); Atlantic bluefin tuna Thunnus thynnus (Linnaeus, 1758); turbot Scophthalmus maximus (Linnaeus, 1758); meagre Argyrosomus regius (Asso, 1801); Atlantic halibut Hippoglossus hippoglossus (Linnaeus, 1758); Atlantic cod Gadus morhua Linnaeus, 1758; and greater amberjack Seriola dumerili (Risso, 1810).
For freshwater aquaculture: rainbow trout Oncorhynchus mykiss (Walbaum, 1792) and brown trout Salmo trutta Linnaeus, 1758; common carp Cyprinus carpio Linnaeus, 1758; European eel Anguilla anguilla (Linnaeus, 1758); European catfish Silurus glanis (Linnaeus, 1758); African catfish Clarias gariepinus Burchell, 1822; tench Tinca tinca (Linnaeus, 1758); and pikeperch Sander lucioperca (Linnaeus, 1758).
3.1.2. Parasites of public health importance that could infect the most relevant farmed finfish species in the EU/EFTA
SQ1.2: Which are the parasites of public health importance that could infect the most relevant farmed finfish species in the EU/EFTA (from SQ1.1)?
As indicated in Section 1.2, in the context of this opinion, the parasites considered of public health importance are those parasite species that are known to be zoonotic (updating those considered for EFSA BIOHAZ Panel (2010)). The evidently zoonotic parasite species (see Section 1.3.2) which occur in the wild and to which farmed fish produced in EU/EFTA/Faroe Islands/UK aquaculture could be exposed to infection, are shown in Table 2.
TABLE 2
Parasites of public health importance that could infect the most relevant farmed finfish species in the EU/EFTA/Faroe Islands/UK.
Phylum | Family | Genus | Species |
---|---|---|---|
Nematoda | Anisakidae | Anisakis | A. simplex (s. s.) |
A. pegreffii | |||
Phocanema a | Ph. decipiens (s. l.) | ||
Contracaecum | C. osculatum (s. l.) | ||
Platyhelminthes (Trematoda) | Heterophyidae | Cryptocotyle | C. lingua |
Opisthorchiidae | Opisthorchis | O. felineus | |
Metorchis | Metorchis spp. | ||
Pseudamphistomum | P. truncatum | ||
Cyathocotylidae | Paracoenogonimus | P. ovatus | |
Platyhelminthes (Cestoda) | Diphyllobothriidae | Dibothriocephalus a | Dibothriocephalus spp. |
Abbreviations: s. l., sensu lato; s. s., sensu stricto.
3.1.3. Occurrence of parasites of public health importance in fishery products derived from the most relevant farmed fish species in the EU/EFTA
SQ1.3: Considering SQ1.1 and SQ1.2, what is the occurrence of zoonotic parasites of public health importance in fishery products derived from the most relevant farmed finfish species in the EU/EFTA (from SQ1.1)?
The occurrence of parasites considered of public health importance (Section 3.1.2) in the different farmed finfish species, as reported in the reviewed literature, is specifically outlined below (Sections 3.1.3.1–3.1.3.15) and summarised in Table 3. As indicated above, available information from Faroe Islands and UK aquaculture was considered.
TABLE 3
Parasitological surveys of public health important parasites in farmed finfish produced in the EU/EFTA/UK from 2010 to 2023.
Marine fish species | ||||||
---|---|---|---|---|---|---|
Fish species | No of analysed fish | Sampling origin (sea/country/point of sale/product type) | Period covered by the studies | Infection level a | Survey detection methodology | References |
Atlantic salmon | 270 | Retail: smoked slices, 100–200 g, obtained in supermarkets from Spain, France and Italy. Unknown origin of fish | 2016 |
P![]() ![]() | VI(TI)/UV‐press | Pardo González et al. (2020) |
10 | Retail: fillets sampled in Belgium, unknown origin of fish | 5/2018–5/2019 | P![]() ![]() | AD | Mercken et al. (2020) | |
4184 (3525 market quality fish, 395 runts, 264 non‐runt discard fish) | Atlantic/Coastal Norway | 1/2014–7/2015 | P![]() ![]() | UV‐press | Levsen and Maage (2016) | |
100 (50 market quality fish, 50 runts) | Atlantic/Coastal Norway | 07/2011 | P![]() ![]() | VI/AD | Mo et al. (2014) | |
Marine rainbow trout | 200 | Baltic Sea/Denmark | 3/2016–11/2018 | P![]() ![]() | VI/AD (viscera)/UV‐press (fillets) | Fioravanti et al. (2021) |
170 | Baltic Sea/Denmark | 10/2020–12/2020 | P![]() ![]() | VI/AD (right fillets)/UV‐press (left fillets) | Karami et al. (2022) | |
225 | Atlantic/Coastal Scotland/UK | 1/2012–11/2012 | P![]() ![]() | VI(TI) | Brooker et al. (2016) | |
1038 (860 market quality fish, 178 runts) | Atlantic/Coastal Norway | 2015–2016 | P![]() ![]() | UV‐press | Roiha et al. (2021) | |
190 (95 market quality fish, 95 runts) | Baltic Sea/Denmark | 2012–2013 | P![]() ![]() | VI/AD | Skov et al. (2014) | |
Gilthead seabream | 43 | Mediterranean/Spain | 5/2006–6/2006 | P![]() ![]() | VI | Fernandez‐Jover et al. (2010) |
612 | Mediterranean/Spain (Murcia) | 6/2006–9/2009 | P![]() ![]() | VI/AD | Peñalver et al. (2010) | |
145 | Mediterranean (Adriatic)/Croatia | 9/2008–8/2009 | P![]() ![]() | VI | Mladineo et al. (2010) | |
462 | Mediterranean/Greece and Italy | 11/2008–12/2010 | P![]() ![]() | VI(TI) | Ioannou Kapota (2012) | |
551 | Atlantic and Mediterranean/Spain (Murcia, Cataluña, Valencian Community, Canary Islands and Andalusia) | 3/2010–4/2011 | P![]() ![]() | VI/AD/UV‐Press | APROMAR (2012) | |
53 | Mediterranean (Adriatic and Ionian Seas)/Italy (Apulia) | 9/2012–8/2013 | P![]() ![]() | VI/AD | Goffredo et al. (2019) | |
17 | Retail: sampled in Belgium, unknown origin (17 muscle samples including fillets of 9 whole fish) | 5/2018–5/2019 | P![]() ![]() | AD | Mercken et al. (2020) | |
3105 |
Mediterranean/Italy, Spain and Greece Also including retail: Italian and Spanish imports from Turkey, Greece and Croatia | 3/2016–11/2018 | P![]() ![]() | VI/AD (viscera)/UV‐press (fillets) | Fioravanti et al. (2021) | |
186,060 | Mediterranean (Tyrrhenian Sea)/Italy (Follonica) | 2016–2020 | P![]() ![]() | VI | Castiglione et al. (2021) | |
European seabass | 259 | Mediterranean/Spain (Murcia) | 6/2006–9/2009 | P![]() ![]() | VI/AD | Peñalver et al. (2010) |
151 | Mediterranean (Adriatic)/Croatia | 9/2008–8/2009 | P![]() ![]() | VI | Mladineo et al. (2010) | |
31 | Mediterranean/Spain | 5/2006–6/2006 | P![]() ![]() | VI | Fernandez‐Jover et al. (2010) | |
310 | Atlantic and Mediterranean/Spain (Murcia, Cataluña, Valencian Community, Canary Islands and Andalusia) | 3/2010–4/2011 | P![]() ![]() | VI/AD/UV‐press | APROMAR (2012) | |
916 | Mediterranean/Greece and Italy | 11/2008–12/2010 | P![]() ![]() | VI(TI) | Ioannou Kapota (2012) | |
83 (Italy) 68 (Greece) |
Mediterranean/Italy (Sicily) Also including retail: fish farmed in Greece, sold in Sicily, Italy | 1/2015–8/2016 |
P
P MA | VI/AD | Cammilleri et al. (2018) | |
75 | Mediterranean (Adriatic and Ionian Seas)/Italy (Apulia) | 9/2012–8/2013 | P![]() ![]() | VI/AD | Goffredo et al. (2019) | |
21 | Retail: sampled in Belgium, unknown origin (21 muscle samples including fillets of 11 whole fish) | 5/2018–5/2019 |
P MA MI | AD | Mercken et al. (2020) | |
3051 |
Mediterranean/Italy, Spain and Greece Also including retail: Italian and Spanish imports from Turkey, Greece and Croatia | 3/2016–11/2018 | P![]() ![]() | VI/AD (viscera)/UV‐press (fillets) | Fioravanti et al. (2021) | |
124,040 | Mediterranean (Tyrrhenian Sea)/Italy (Follonica) | 2016–2020 | P![]() ![]() | VI | Castiglione et al. (2021) | |
Atlantic bluefin tuna | 58 | Adriatic/Croatia | 2003, 2005, 2006 |
P MA MI | VI | Mladineo et al. (2011) |
128 | Adriatic/Croatia | 2004–2006 |
P MA MI | VI | Mladineo et al. (2011) | |
120 | Adriatic/Croatia | 2008 |
P MA MI | VI | Mladineo and Poljak (2014) | |
Turbot | 144 | Atlantic/Spain | 3/2010–4/2011 | P![]() ![]() | VI/AD/UV‐press | APROMAR (2012) |
3 | Retail: whole fish sampled in Belgium, unknown origin | 5/2018–5/2019 | P![]() ![]() | AD | Mercken et al. (2020) | |
1035 | Atlantic/Spain | 3/2016–11/2018 | P![]() ![]() | VI/AD (viscera)/UV‐press (fillets) | Fioravanti et al. (2021) | |
Meagre | 72 | Mediterranean/Spain (Murcia, Valencian Community) | 3/2010–4/2011 | P![]() ![]() | VI/AD/UV‐press | APROMAR (2012) |
Atlantic halibut | 150 | Atlantic/West‐coast of Scotland/UK | 2012 | P![]() ![]() | VI(TI) | Brooker et al. (2016) |
Atlantic cod | 136 (hatchery reared) | Atlantic/Norwegian coast | 2006–2008 |
P
P | VI(TI) (left side fillets only) | Heuch et al. (2011) |
Greater amberjack | NA | NA | NA | NA | NA | No reports for the period available |
Freshwater fish species | ||||||
---|---|---|---|---|---|---|
Fish species | No. of analysed fish | Country | Period covered by the studies | Infection level | Survey detection Methodology | References |
Rainbow trout | 180 | Denmark | 2016–2021 | P![]() ![]() | VI/AD (right fillets)/UV‐press (left fillets) | Karami et al. (2022) |
Brown trout | NA | NA | NA | NA | NA | No reports for the period available |
Common carp | 318 | Hungary | 2016–2017 | P![]() ![]() | VI/AD | Sándor et al. (2020), Cech et al. (2021) |
European catfish | 36 | Poland | 2009 | P![]() ![]() | VI of all organs | Sobecka et al. (2010) |
African catfish | NA | NA | NA | NA | NA | No reports for the period available |
European eel | NA | NA | NA | NA | NA | No reports for the period available |
Tench | NA | Germany | 2022 |
P![]() ![]() | VI (combined with compression) | Mata et al. (2023) |
Pikeperch | NA | NA | NA | NA | NA | No reports for the period available |
Abbreviations: AD, artificial digestion; MA, mean abundance; MI, mean intensity; NA, not available; P, prevalence; TI, transillumination (candling); UV, ultraviolet; VI, plain visual inspection.
3.1.3.1. Atlantic salmon
In EFSA BIOHAZ Panel (2010), the available evidence suggested that for farmed Atlantic salmon reared in floating cages or onshore tanks, and fed compound feedstuffs which cannot contain live parasites, the risk of infection with larval anisakids was negligible unless changes in farming practices occur (EFSA BIOHAZ Panel, 2010). Since 2010, there were four studies that analysed farmed Atlantic salmon or products thereof for the presence of anisakid parasites (Table 3), two of which detected A. simplex (s. s.) larvae in runts in the sea cages (Levsen & Maage, 2016; Mo et al., 2014). The findings were attributed to opportunistic feeding of the runts on infected planktonic or semi‐planktonic crustaceans or smaller wild fish which occasionally cross the side nettings. Due to their poor appearance, runts are readily discarded during harvest and processing and do not reach the market. However, the findings show that A. simplex (s. s.) larvae may enter the salmon production cages along coastal Norway, suggesting that market quality fish are exposed to the parasite.
3.1.3.2. Marine rainbow trout
In EFSA BIOHAZ Panel (2010), it was considered that sufficient monitoring data were not available to conclude about the presence of zoonotic parasites in maricultured rainbow trout. Since then, there were five studies focusing on marine rainbow trout produced in Denmark, Norway or in the UK (Table 3). The studies showed that market quality farmed marine rainbow trout were free of zoonotic parasites (Brooker et al., 2016; Fioravanti et al., 2021; Karami et al., 2022; Roiha et al., 2021; Skov et al., 2014). However, as was found for Norwegian Atlantic salmon, a few runts of maricultured rainbow trout in Norway were shown to be infected with A. simplex (s. s.) larvae (Roiha et al., 2021) (Table 3). Since the culturing conditions of Atlantic salmon and rainbow trout are identical in Norway, the same mechanisms of transmission are most likely involved in the A. simplex (s. s.) infections in the runts of both fish species. As for Atlantic salmon, runts of maricultured rainbow trout are readily discarded during harvest and processing and do not reach the market. However, the findings show that A. simplex (s. s.) larvae may enter the rainbow trout production cages along coastal Norway, suggesting that market quality fish are also exposed to the parasite. In Denmark, rainbow trout mariculture facilities are located in brackish water where the parasite infection pressure is considered lower due to low water salinity, and no infections were reported for this production.
3.1.3.3. Gilthead seabream
In EFSA BIOHAZ Panel (2010), it was indicated that no information was available on infections of farmed gilthead seabream with larval anisakids. Since then, nine studies have investigated farmed gilthead seabream farmed mainly in the Mediterranean Sea, but also from Canary Islands farms, and others with unknown origin, for the presence of anisakids (Table 3). In these studies, no zoonotic parasites were reported for farmed gilthead seabream. Some Contracaecum spp. larvae were found in farmed gilthead seabream from Sardinia (Italy) (Salati et al., 2013). However, the authors indicated that the larvae likely belong to species maturing in piscivorous birds (Salati et al., 2013). Any zoonotic potential of these species has not been demonstrated to date (see Section 1.3.2.1).
3.1.3.4. European seabass
In EFSA BIOHAZ Panel (2010), it was indicated that no information was available on infections of farmed European seabass with larval anisakids. Since then, 10 studies have investigated European seabass farmed mainly in the Mediterranean Sea but also from Canary Islands farms for the presence of Anisakis (Table 3). In eight of the surveys, no zoonotic parasites were found, while one study reported the presence of two A. pegreffii larvae in the body cavity of one farmed European seabass from Greece sold at a fish market in Sicily, Italy (Cammilleri et al., 2018). In another study, Mercken et al. (2020) detected two A. simplex (s. s.) larvae in the viscera and fillet, respectively, of two farmed seabass sampled randomly at a fish market in Belgium. The farming location of the fish was unknown (Table 3).
3.1.3.5. Atlantic bluefin tuna
In EFSA BIOHAZ Panel (2010), it was stated that farmed tuna may be infected with larval anisakids. Since then, two studies have shown that farmed tuna are infected with A. pegreffii and A. simplex (s. s.), mostly beneath the stomach serosa and to a lesser extent, in the pyloric caeca serosa (Mladineo et al., 2011; Mladineo & Poljak, 2014) (Table 3). One of two studies demonstrated that wild‐caught juvenile tuna prior to release into net‐pen cages, had twice as high level of Anisakis spp. infections compared to fish that were caged for more than 1½ years (Mladineo et al., 2011). The prevalence ranged from 17.1% to 32.8%.
3.1.3.6. Turbot
In EFSA BIOHAZ Panel (2010), it was stated that there was no information available on infections of farmed turbot with larval anisakids. Since then, two studies investigated the possible presence of anisakid parasites in farmed turbot along the Atlantic coast of Spain in land‐based farming systems (Table 3). In these studies, no zoonotic parasites were reported for farmed turbot. Additionally, Mercken et al. (2020) did not detect any parasites in three farmed turbots (farming location unknown) sampled randomly at a fish market in Belgium (Table 3).
3.1.3.7. Meagre
This fish species was not considered in EFSA BIOHAZ Panel (2010). The presence of anisakids was tested in meagre in a study undertaken in Spain (N=
72), but no parasites were detected (APROMAR, 2012) (Table 3). However, sufficient monitoring data are lacking for farmed meagre.
3.1.3.8. Atlantic halibut
This fish species was not considered in EFSA BIOHAZ Panel (2010). A single study reported negative result for 75 farmed halibut in Scotland which were inspected visually by the naked eye and candling of fillet slices (Brooker et al., 2016) (Table 3). Thus, only limited data are available for Scotland and monitoring data are lacking for Norway.
3.1.3.9. Atlantic cod
In EFSA BIOHAZ Panel (2010), it was stated that there was no information available on the presence of parasites in farmed Atlantic cod. Since then, only a single parasitological study on farmed cod has been carried out, including 136 hatchery‐reared fish from western and central coastal Norway (Heuch et al., 2011) (Table 3). The study revealed the occurrence of C. lingua and A. simplex (s. l.). In the same study, the presence of C. lingua was reported with a prevalence ranging from 55% to 100% (Heuch et al., 2011). Additional data come from a routine veterinary inspection of farmed cod from a coastal sea‐cage locality in northern Norway, 26 where a single Anisakis larva (not specifically identified) was found on the liver of a cod runt. Additionally, the presence of ‘black spots’ (probably melanised cysts of C. lingua metacercariae) in/on the skin of some of the inspected cod were observed.
3.1.3.10. Greater amberjack
This fish species was not considered in EFSA BIOHAZ Panel (2010). No monitoring data is available for farmed greater amberjack.
3.1.3.11. Rainbow trout and brown trout
Since EFSA BIOHAZ Panel (2010), there was one study focusing on freshwater farmed rainbow trout in Denmark (Karami et al., 2022), and no zoonotic parasites were detected. Freshwater rainbow trout farms are usually connected to natural water bodies (streams, lakes); thus, the fish may be exposed to snail‐borne zoonotic parasites, such as trematodes. Since this study exclusively investigated freshwater‐reared rainbow trout in Denmark, monitoring data are lacking from other freshwater rainbow trout producing countries in Europe.
There are no records or reports of any findings of potentially zoonotic parasites in farmed brown trout in the scientific literature reviewed for the current opinion.
3.1.3.12. Common carp and catfish
Common carp is mostly reared in earth pond systems connected to natural freshwater bodies in several central and eastern European countries. Several studies have shown that wild cyprinids in natural freshwaters can carry various snail‐borne parasites, such as Opisthorchis spp. and Metorchis spp. It could therefore be expected that cultured carp is exposed to infection. However, a parasitological survey of cultured common carp in Hungary did not detect these parasites, and the metacercariae found were non‐zoonotic (Cech et al., 2021; Sándor et al., 2020). Monitoring data from other major carp producing countries including Poland, Czechia and Germany are lacking.
European catfish and African catfish were not considered in EFSA BIOHAZ Panel (2010). Small‐scale parasitological studies of farmed European catfish in Poland did not reveal any zoonotic parasites in the fish (Sobecka et al., 2010). In Europe, African catfish are exclusively produced in facilities with recirculating aquaculture systems (RAS), suggesting that the fish are not exposed to zoonotic parasites. However, monitoring data are not available for African catfish.
3.1.3.13. European eel
Cultured eel is usually produced by stocking wild‐caught glass‐eels in RAS. It is generally considered that glass‐eels are susceptible to zoonotic anisakid nematodes. However, there are no studies that target zoonotic parasites in glass‐eels.
3.1.3.14. Tench
This fish species was not considered in the previous EFSA BIOHAZ Panel (2010). A recent study (Mata et al., 2023) revealed the presence of metacercariae of two potentially zoonotic trematode species, i.e. Pseudamphistomum truncatum (Opistorchiidae) and Paracoenogonimus ovatus (Cyathocotylidae) in the body musculature of both wild and pond cultured tench in eastern Germany. Metacercariae of P. truncatum were also isolated in wild tench from lakes of North Italy (Caffara et al., 2020). The prevalence of the metacercariae in farmed tench ranged from 9% to 100%. Since consumption of raw or semi‐raw marinated fillets of tench appears to be common, especially in parts of central and southern Europe (Pozio et al., 2013), there is a risk of human infection with these trematodes. However, monitoring data are lacking from other tench producing countries or areas in Europe.
3.1.3.15. Pikeperch
This fish species was not considered in EFSA BIOHAZ Panel (2010). Pikeperch reared in open farming systems with water inlets from rivers, streams and lakes may be exposed to infective parasite stages such as cercariae released from snail intermediate hosts. In Europe, pikeperch are largely produced in RAS, suggesting that the fish are not exposed to zoonotic parasites. However, there are no studies specifically targeting zoonotic parasites in farmed pikeperch.
Additional information extracted collected for the ‘Pathogens in foods database’ (see Section 2.2.2, and Annex E, section E3) can be found in https://doi.org/10.5281/zenodo.10790873.
Uncertainties
As is evident from the relatively few studies mentioned above, there is a lack of surveillance data for zoonotic parasites in the target fish species. More specifically:
a) there are no studies for specific fish species (e.g. greater amberjack, brown trout, African catfish, European eel, tench and pikeperch) and a limited number of studies for other species;
b) the studies that are available do not cover all of the different producing countries, e.g. rainbow trout are farmed in a wide number of EU Member States (in 2021, in 23 EU Member States, mostly in France, Italy and Denmark), and from those Member States only published data were available for Denmark (together with data from Norway and UK [Scotland]); Portugal, France and the Netherlands have significant turbot fish farming activities but no published data on parasites in turbot for these countries; Atlantic halibut are farmed in Norway and in UK (Scotland) but there is only published data available for Scotland, etc., as well as other examples mentioned above;
c) in some studies, the sample size of collected fish is low for a proper statistical analysis and not representative of the large numbers of fish produced;
d) the majority of studies of maricultured fish focuses exclusively on anisakids;
e) the majority of studies has been performed on a single occasion and the seasonal or yearly data are missing;
f) moreover, the methodology is often not detailed or low‐resolution methods are used. Even when the parasite detection methods used are sufficiently sensitive, the lack of standardisation inhibits comparison of the data in the different studies.
Due to the lack of representative data, it is not possible to make informative estimates of the prevalence or the abundance of those parasites considered of public health importance for all fish species, farming systems or production area in the EU/EFTA.
3.1.4. Farming practices which influence the likelihood of parasite infection of fish
Although uninfected and disinfected fish eggs and larvae are used for aquaculture, fish may be exposed to parasites through the occasional access of intermediate and paratenic hosts in open flow‐through freshwater systems or seawater cages. Feeding with baitfish, such as in Atlantic bluefin tuna and to some extent in the greater amberjack aquaculture, increases the possibility of fish exposure to anisakids. This risk is almost certainly eliminated when the fish are produced in closed recirculating aquaculture systems (RAS) or flow‐through facilities where the fish are reared in indoor or roofed facilities with filtered and/or treated water intake and the fish is exclusively fed heat‐treated feed. The offshore location of salmon farms may also result in increased exposure of the fish to anisakids as they are situated close to marine mammals' migration routes and habitats, especially cetaceans, that act as main definitive hosts of this parasite.
In some regions of the Mediterranean, larger seabass intermittently fed with unprocessed fish offal may be exposed to anisakids.
Capture‐based aquaculture that relies on the capture of juvenile wild fish for subsequent on‐growing and fattening in captivity, as used for the Atlantic bluefin tuna in Europe, also potentially facilitates exposure of the fish to parasites.
Further information on farming practices that constitute a risk were described in EFSA BIOHAZ Panel (2010) and Crotta et al. (2016).
Use of cleaner fish: There are no reports of anisakid nematodes, or any other zoonotic parasites potentially transferable to farmed salmonids, in commercially produced cleaner fish. Although hatchery‐reared cleaner fish are increasingly produced, substantial numbers of wild cleaner fish, especially wrasses, are still employed (Erkinharju et al., 2021). For example, close to 13.7 million individual wrasses were live caught along coastal Norway in 2022, and subsequently released into Norwegian salmon and rainbow trout sea cage facilities for delousing purposes. 27
3.1.5. Concluding remarks for ToR1
EFSA was requested to review and assess:
ToR1. The occurrence of parasites of public health importance in fishery products derived from the most relevant farmed fish species in the EU (in particular, but not limited to, Atlantic salmon, seabass, farmed seabream and turbot).
AQ1: What is the occurrence of parasites of public health importance in fishery products derived from the most relevant farmed finfish species in the EU/EFTA?
SQ1.1: Which are the most relevant farmed fish species produced in the EU/EFTA, in addition to Atlantic salmon, seabass, seabream and turbot that may be infected with parasites of public health importance?
The most relevant farmed finfish species in the EU/EFTA, based on production and consumption data, are Atlantic salmon, rainbow trout, gilthead seabream, European seabass, Atlantic bluefin tuna, turbot, meagre, Atlantic cod, Atlantic halibut and greater amberjack in marine farming systems; and rainbow and brown trout, common carp, European and African catfish, European eel, tench and pikeperch in freshwater fish farming.
SQ1.2: Which are the parasites of public health importance that could infect the most relevant farmed finfish species in the EU/EFTA (from SQ1.1)?
The parasites of public health importance occurring in the wild and with which farmed fish produced in EU/EFTA aquaculture could be infected, include:
- ○ in the marine environment the nematodes A. simplex (s. s.), A. pegreffii, Phocanema decipiens (s. l.) and Contracaecum osculatum (s. l.) as well as the trematode Cryptocotyle lingua;
- ○ in freshwater systems the trematodes Opisthorchis felineus, Metorchis spp., Pseudamphistomum truncatum, Paracoenogonimus ovatus and the cestode Dibothriocephalus spp.
SQ1.3: Considering SQ1.1 and SQ1.2, what is the occurrence of parasites of public health importance in fishery products derived from the most relevant farmed finfish species in the EU/EFTA (from SQ1.1)?
The experts consider it to be 99%–100% certain (almost certain) that fish produced in recirculating aquaculture systems (RAS), or indoor or roofed facilities with filtered and/or treated water intake are not exposed to parasites provided the fish is exclusively fed heat‐treated feed.
Fish farmed in open marine offshore cages or open flow‐through freshwater ponds or tanks can be exposed to zoonotic parasites.
- • In summary the data on occurrence, since 2010 were as follows:
- ○ Atlantic salmon: Four studies, all testing for anisakids, did not record infection in market quality fish.
- ○ Marine rainbow trout: Five studies since 2010 (Denmark, Norway and UK), all testing for anisakids, did not record infection in market quality fish.
- ○ Gilthead seabream: Nine studies since 2010 (Mediterranean Sea), all testing for anisakids; no zoonotic parasites detected.
- ○ European seabass: 10 studies since 2010 (9 from Mediterranean Sea, 1 based on fish from retail), all testing for anisakids; no parasites detected in 8/10; two A. pegreffii larvae detected in one farmed fish (Greece), two A. simplex (s. s.) larvae detected in two farmed fish of unknown farming origin.
- ○ Atlantic bluefin tuna: Two studies since 2010, testing for anisakids (Adriatic Sea, Croatia); Atlantic bluefin tuna infected with A. pegreffii and A. simplex (s. s.) with prevalence values from 17.1% to 32.8%.
- ○ Turbot: Three studies since 2010 (Atlantic coast of Spain), all testing for anisakids; no parasites detected.
- ○ Meagre: One study since 2010 (Spain), testing for anisakids; no parasites detected.
- ○ Atlantic halibut: One study since 2010 (UK ‐ Scotland), testing for anisakids; no parasites detected.
- ○ Atlantic cod: One study since 2010 (Norway), detected C. lingua (prevalence 55% to 79%) and A. simplex (s. l.) (1%) in hatchery reared fish.
- ○ Greater amberjack: No studies available.
- ○ Freshwater rainbow trout: One study since 2010 (Denmark), all testing for anisakids; no parasites detected.
- ○ Brown trout: No studies available.
- ○ Common carp: One study since 2010 (Hungary); no parasites detected.
- ○ European catfish: Several small‐scale studies since 2010 (Poland); no parasites detected.
- ○ African catfish: No studies available.
- ○ Tench: One study since 2010 (Germany), presence of metacercariae of two potentially zoonotic trematode species, i.e. Pseudamphistomum truncatum (Opistorchiidae) and Paracoenogonimus ovatus (Cyathocotylidae) detected.
- ○ European eel and Pikeperch: No studies available.
- • Due to the lack of representative data, it is not possible to make informative estimates of the prevalence or the abundance of those parasites, that are considered to be of public health importance, for all fish species, farming systems and production area in the EU/EFTA.
3.2. ToR2: Diagnostic methods for the detection of parasites of public health importance in fishery products from such farmed fish species
AQ2: What testing methods are currently available and may be available in the near future to test the fish species for the parasites identified in the answer to ToR1?
Diagnostic methods for the detection of parasites in fishery products can be divided into methods that are employed for visualisation and isolation of the parasite, and methods to identify the parasite. Either methods are used for detection of larval or juvenile stages of nematodes, trematodes and cestodes species, as described in Sections 1.3.2 and 3.1.2 (Table 4).
TABLE 4
Methods to visualise, isolate and identify larval parasites.
Methods included in EFSA BIOHAZ Panel (2010) | Parasites | Advances since 2010 | Advantages | Disadvantages | Main reference(s)/links | ||
---|---|---|---|---|---|---|---|
1. Methods to visualise and isolate | 1.1. Visual and optical inspection including candling | Already available | Nematodes | None | Non‐destructive | Detection of only macroscopically visible parasites; subjective efficiency (level of operator's experience); no quantitative results; variability dependent on fillet thickness, presence of skin, oil content, pigmentation | Mercken et al. (2022) |
1.2. Compression, including UV‐press method and subsequent identification | Already available | Nematodes, other helminths | The method has been standardised for nematodes within Anisakidae (ISO 23036‐1:2021) | Detection even in pre‐frozen fillets; quantifiable; determination of spatial distribution of Anisakidae larvae in the fillets and visceral organs | Destructive; less reliable with very low or very high numbers of larvae | Gómez‐Morales et al. (2018), Levsen et al. (2018), ISO 23036‐1:2021 (ISO, 2021b) | |
1.3. Artificial digestion and subsequent microscopical evaluation | Already available | Nematodes and other helminths (e.g. trematodes, cestodes, acanthocephalans) | The method has been standardised for nematodes within Anisakidae (ISO 23036‐2:2021); use of liquid pepsin for artificial digestion | Relatively low‐cost, for batch sampling (surveys) | Enzymatic degradation of some parasites from frozen samples; better for live than frozen parasitic larvae; less sensitive compared to UV‐press method; time‐consuming | Gómez‐Morales et al. (2018), Levsen et al. (2018), ISO 23036‐2:2021 (ISO, 2021a) | |
Development of orbital digestion for detection of Cryptocotyle | Preserves metacercaria morphology up to 8![]() | Borges et al. (2015), Mehrdana et al. (2015), Duflot et al. (2021) | |||||
1.4. Optical sensing | Not available | Nematodes | Magnetic resonance imaging (MRI) | Detection in live fish; accumulation and movements of A. simplex; genus‐specific | Requires specific hardware, and training; data acquisition is slow (13 min/fish) | Bao et al. (2017) | |
Hyperspectral (HS), ultrasound (US), X‐ray imaging (dual energy ray CT and planar X‐ray) |
Improvements in illumination enabled a significant increase of the signal to noise ration Fast processing (1 fillet/s) with HS; Dual energy ray CT detects nematodes deep in the muscle | HS requires more standardisation (detection rate depends on storage time and sample handling); US produces noisy images, with no contrast between the nematode and muscle; long image acquisition in dual energy ray CT detects; low contrast in planar X‐ray | Sivertsen et al. (2011, 2012), Xu et al. (2023), Heia et al. (2017) | ||||
2. Methods to identify | 2.1. Microscopical evaluation of morphological and morphometric parameters | Already available | All parasites | None | Inexpensive; detection to genus level | Requires training; no identification to the species level; time consuming | Skov et al. (2008, 2009) |
2.2. Immunological methods | Not available | Nematodes | ELISA detection of Anisakis allergens by polyclonal IgG and IgE in fish products (in house validation, in Norway, 2015 for testing of allergenicity in aquaculture fish) | Sensitivity usually high | LOD (limit of detection) varies by fish product; specificity depends on the applied antibody due to frequent cross‐reactions between anisakid genera | Werner et al. (2011), Fæste, Plassen, et al. (2015), Fæste, Plassen, et al. (2015) | |
Chemiluminescent ELISAs: CL sandwich ELISA (S‐ELISA) and CL competitive (C‐ELISA) | LOD 0.5–5 ng/mL in highly processed seafood (where qPCR failed) | Requires specific equipment and training | Kochanowski et al. (2020) | ||||
2.3. Molecular identification by PCR | EURL‐Parasites PCR/RFLP ITS rDNA for Anisakidae larvae identification |
Nematodes Anisakidae | Sensitivity and specificity high, without the need to sequencing the locus |
Requires specific equipment and training It does not correctly identify hybrid genotypes between sibling species of anisakids | EURL‐Parasites https://www.iss.it/documents/5430402/5722370/MI‐04‐rev.‐3.pdf/67b30531‐6d79‐9b10‐87c3‐4abae2a9764c?t=1620381675883 | ||
EURL‐Parasites Multiplex PCR for Anisakidae larvae identification. Based on ITS rDNA gene | Requires specific equipment and training | https://www.iss.it/documents/5430402/5722370/MI‐10‐rev‐1.pdf/7e614838‐7235‐6271‐9dbc‐e0cc68db9228?t=1620381678759 | |||||
Multiplex qPCR of the mtDNA cox2 gene | Detects, at species level, in both singleplex and multiplex, 0.0006 ng/μL of DNA of A. pegreffii, A. simplex (s. s.), Ph decipiens (s. s.), Ph. krabbei, Hysterothylcium aduncum. Validated on parasites isolated from fish and DNA of the parasites in biopsy samples of human cases of anisakiasis | It requires specific probes. The method has not been so far performed on fish products outside the framework of EU‐PAPASITE project | Paoletti et al. (2018), Mattiucci, Paoletti, et al. (2017), Mattiucci et al. (2018), Parasites consortium (2016) | ||||
TaqMan assay for A. simplex ITS and mtDNA cox2 locus | Detects as little as 0.1 pg of A. simplex (s. l.) DNA | Requires specific probe. Lacks specificity | Lopez and Pardo (2010), Mossali et al. (2010), Herrero et al. (2011) | ||||
Validation of PATHfinder Anisakis/Phocanema DNA detection assay with TaqMan (ITS locus) | Anisakis spp., Phocanema spp. | It requires specific probe | Cavallero et al. (2017) | ||||
SYBR Green qPCR assay for the mtDNAcox2 | Detects (LOD![]() ![]() | Lack of specificity in anisakids detection. Not yet investigated over large samples | Godinez‐Gonzales et al. (2017) | ||||
Sequence analysis of the nDNA gene EF1 | Diagnostic SNPs allow the identification of A. pegreffii and A. simplex (s. s.) | Not yet investigated for other anisakid species | Mattiucci et al. (2016) | ||||
Sequences analysis of the mtDNA cox2 | Allows the identification of all the anisakid species so far recognised | It does not detect hybrids between sibling species of anisakids because it is a mitochondrial gene | Mattiucci et al. (2018) | ||||
Sequence analysis and ARMS_PCR‐DNA assay of the nuclear gene nas10 | Diagnostic SNPs allow the rapid identification of the sibling species of the A. simplex (s. l.) complex (i.e. A. pegreffii, A. simplex (s. s.) and A. berlandi) and hybrid genotypes in a multilocus approach. Validated on several hundreds of specimens | Not yet investigated for other anisakid species | Palomba, Paoletti, et al. (2020) | ||||
Microsatellite DNA loci (SSRs DNA loci) | Diagnostic alleles allow the rapid identification of the sibling species of the A. simplex (s. l.) complex (i.e., A. pegreffii, A. simplex (s. s.) and A. berlandi); validated in hundred specimens of the three Anisakis species (Bello et al., 2020; Mattiucci et al., 2019). Distinction of hybrid specimens between A. simplex (s. s.) and A. pegreffii as well as between A. pegreffii and A. berlandi. Allow intraspecific differentiation among populations of these Anisakis spp. | Not yet investigated for other anisakid species | Mladineo et al. (2017), Mattiucci et al. (2019), Bello et al. (2020, 2021) | ||||
LAMP (Loop‐Mediated Isothermal Amplification) assay: Anisakis | Sensitivity better than qPCR (detects 102 less of DNA concentration); works at 65°C (lower compared to conventional PCR); less time than qPCR, usable in industry | It is not able to identify the parasites to the species level |
Cammilleri et al., 2020 | ||||
Recombinase polymerase amplification (RPA) SYBR Green I (RPA‐SG): Detection of A. simplex, A. pegreffii, A. typica by ITS locus | Faster than qPCR, similar sensitivity | Not yet validated over a large number of specimens | Chen et al. (2022) | ||||
Trematodes Opistorchiidae and Heterophyidae | Multiplex and nested PCR for detection of Centrocestus formosanus; European Opisthorchiid and Heterophyid in fish or fish products, by using 18S, rDNA ITS, mtDNA cox1 and mtDNA cytb | Possibility to detect multiple species isolated from fish tissues | Not yet validated over a large number of specimens | Caffara et al. (2020), Jaruboonyakorn et al. (2022) | |||
PCR RFLP of the 28S rDNA | Genotyping of C. formosanus without the need to sequence the 28S rDNA locus | Not yet validated over a large number of specimens | Thaenkham et al. (2011) | ||||
EURL‐Parasites ITS2 PCR for Opisthorchiidae: Opisthorchis felineus, O. viverrini, C. sinensis | Detects eggs of Opistorchiidae in faecal samples of human and animal origins | Not yet tested in infected seafood samples | https://www.iss.it/documents/20126/9277401/MI‐08+%28rev+4%29.pdf/68ea08e7‐a1c4‐7fa4‐31e0‐f1f630f784ed?t=1708591329854 | ||||
O. felineus PCR mtDNA cox1 locus | Species‐specific validation of 40 blind specimens, in compliance to ISO 17025 certification | Not yet validated in infected seafood samples | Caffara et al. (2017) |
Abbreviations: ARM, amplification‐refractory mutation system; CT, computed tomography; EF, elongation factor; ELISA, enzyme‐linked immunosorbent assay; EURL‐Parasites (EURLP), European Reference Laboratory for Parasites; HS, hyperspectral; ITS, internal transcribed spacers; LAMP, loop‐mediated isothermal amplification; LOD, limit of detection; MRI, magnetic resonance imaging; mtDNA, mitochondrial DNA; PCR, polymerase chain reaction; qPCR, real‐time (quantitative) PCR; rDNA, ribosomal DNA; RFLP, restriction fragment length polymorphism; RPA, recombinase polymerase amplification; SSR, simple sequence repeats; US, ultrasound; UV‐Press, ultraviolet press.
3.2.1. Current diagnostic methods
Methods currently in use are essentially those described in EFSA BIOHAZ Panel (2010) with some having undergone further optimisation (Table 4).
3.2.1.1. Methods to visualise and isolate parasites
The current methods to visualise and isolate parasites include visual inspection, artificial digestion and UV‐press method.
Visual inspection of fishery products includes candling, sometimes referred as transillumination (a method whereby visible ascaridoid larvae are detected in fish slices on a light table) (Mercken et al., 2022). This method has low sensitivity, e.g. detecting only 7%–10% of anisakid L3, however, it is the only non‐destructive method for parasite detection. The methodology and equipment were described in EFSA BIOHAZ Panel (2010). Candling is mostly useful for the detection of larger nematode larvae. A recently reported sensitivity for ascaridoids in fish fillets was 31% and the negative predictive value (NPV) was 87%. However, the sensitivity increases with the increase of larvae per 100 g of infected muscle. Phocanema spp. larvae are recovered at a higher percentage as they are darker and larger (Mercken et al., 2022). There has been no change in this method since 2010.
Artificial digestion mimics the digestion process in a carnivorous animal and involves the use of a pepsin and hydrochloric acid solution to free parasitic larvae from muscle or other tissues (EFSA BIOHAZ Panel, 2010). The filtrate is subsequently examined by microscopy, recovering virtually all parasites, although it is time‐consuming and thus used for specific surveys rather than mass screening. Identification of isolated nematode larvae to the genus level can be achieved by light microscopy, but species identification requires the application of molecular methods. Artificial digestion is very useful for products containing live parasite larvae (Mehrdana et al., 2015), but less sensitive if larvae are dead (e.g. in frozen products, Sánchez‐Alonso, Rodríguez, Tejada, et al., 2021) because live parasite larvae have a range of molecules, that make them resistant to the action of pepsin and hydrochloric acid.
The main improvement since 2010 of the artificial digestion for anisakids detection was the use of the liquid pepsin (Llarena‐Reino et al., 2013) and standardisation of the method, becoming the ISO 23036‐2:2021 standard (ISO, 2021a). The recovery of anisakids is 91.7%, with sensitivity and specificity of 93%–100%, and accuracy of 97% (Guardone et al., 2016). The method is also used for the extraction of trematodes (metacercariae) and cestodes (plerocercoids) from large quantities of fish (Borges et al., 2015; EFSA BIOHAZ Panel, 2010). Recently, four artificial digestion protocols have been compared for the detection of Cryptocotyle metacercariae in fish fillets, suggesting that the orbital digestion was the least destructive for metacercariae, allowing their better quantification and a reliable morphological identification up to 8days post‐mortem (Duflot et al., 2021). Due to considerable size differences between parasite types (trematode metacercaria ~ of <
1
mm, cestodes between 1 and 30
mm and anisakid larvae between 1 and 45
mm), appropriate filter sizes should be selected. This method is better than visual inspection, and it is particularly useful for metacercariae for which conventional detection methods (microscopic examination of compressed flesh samples) are time‐consuming and lack sensitivity.
UV‐press method (i.e. compression of the edible portion followed by freezing and UV exposure) and subsequent microscopical evaluation is well adopted and more widely applied for nematode larvae detection since 2010 (Levsen et al., 2018). Various types of equipment may be used for the process (EFSA BIOHAZ Panel, 2010), which shows higher reproducibility than artificial digestion, but with no differences in accuracy and specificity (Gómez‐Morales et al., 2018). Since 2010, the method has been standardised for the detection of anisakids, becoming the ISO 23036‐1:2021 standard (ISO, 2021b).
The spectral signature of the nematode larvae depends on the host muscle and its biochemical components, but it is usually detected in the range of 370 to 600 nm. Darker nematodes, such as Phocanema spp. emit another fluorescent peak at around 630–650 nm, likely associated with met‐haemoglobin (Mhb) (Klapper et al., 2021).
A prototype tool capitalising on the UV‐press method is the UV‐scanner (Parasites project final report 28 ), now commercially available. It consists of a black box with UV light where the fillet is inserted, and a scanning device with a monitor that visualises the fillet under the UV. However, to date, this scanning technique has not been fully validated for all types of zoonotic parasites in different fishery products.
For both methods, the UV‐pressing and the artificial digestion, it is possible to quantify the number of larvae with a high confidence level, but both are destructive and less reliable with very low or very high numbers of larvae.
3.2.1.2. Methods to identify parasites
The methods to identify parasites include microscopic evaluation, immunological methods and molecular identification (Table 4).
Microscopical evaluation of morphological and morphometric parameters has been described in the previous EFSA opinion (EFSA BIOHAZ Panel, 2010) and have not changed since then. It involves mounting of the live and/or dead stained and/or unstained parasites on microscope slides and light microscopical evaluation of the morphological and morphometric parameters. It is not species‐specific but facilitates the identification of the isolated parasites to the genus level, when morphological features are visible.
Immunological methods targeting parasite antigens in seafood extracts comprises an ELISA method using polyclonal antibodies (Fæste, Levsen, et al., 2015; Fæste, Plassen, et al., 2015; Werner et al., 2011). The sensitivity has been confirmed and the specificity documented by an enzyme‐linked immunoassay (ELISA) applying IgE from an allergic patient. The method is destructive, necessitates staff training but requires relatively non‐expensive equipment. The sensitivity is high, even for samples that were false‐negative when tested by commercial qPCR (see below), but the limit of detection (LOD) varies depending on the fish species of the raw material (Kochanowski et al., 2020). Due to cross‐allergenicity among anisakids, the choice of detecting antibody is crucial for accurate species identification (Mehrdana et al., 2021).
Molecular identification. After the isolation of the worms and DNA extraction, identification can be done by polymerase chain reaction (PCR)‐amplification of the DNA targets of interest. If standard procedures are followed and the parasites are stored in ethanol and/or frozen, the quality and quantity of extracted DNA will not be affected (Sales et al., 2020). Consequently, a target fragment of purified DNA is amplified using PCR. The most common target fragment for amplification in parasites is nuclear and/or mitochondrial genome sequences. All parasite species may be identified using this method after selecting the appropriate primer set. Although this technology was available in 2010, the number of potential DNA target gene loci and types of PCR has increased significantly, facilitating increased diagnostic precision.
Application for the identification of different types of parasites is described below.
The methodology for genetic identification of nematodes relies on the sequencing of conventional nuclear gene loci, such as the ribosomal DNA that includes the 18S, internal transcribed spacers ITS‐1, 5.8S, ITS‐2 and 28S. Primers for amplification of ITS target locus for anisakids have been reviewed in Mattiucci et al. (2018) and Karami et al. (2022). New nuclear loci targets, which successfully differentiate sibling species within the A. simplex (s. l.) complex include the elongation factor (EF) alpha‐1 (Mattiucci et al., 2016), the metallopeptidase nas10 (Palomba, Paoletti, et al., 2020) and DNA microsatellites (SSRs loci) (Bello et al., 2020, 2021; Mattiucci et al., 2019; Mladineo et al., 2017). The latter were not developed to identify all the anisakid species. In contrast, the mitochondrial gene locus mtDNA cox2 (see Mattiucci & Nascetti, 2008) allows the molecular identification of all the anisakid species of genera Anisakis, Phocanema and Contracaecum. To quantify DNA of anisakids by a real‐time PCR (qPCR), a TaqMan assay for the ITS locus is used (Lopez & Pardo, 2010; Mossali et al., 2010). For identification of zoonotic species of the genus Anisakis and Phocanema spp., a multiplex qPCR detection assay was developed and commercialised, and subsequently validated by Cavallero et al. (2017). Another multiplex qPCR that uses mtDNA cox2 locus was developed for the identification of the zoonotic species infecting fish in European waters, i.e. Anisakis pegreffii, A. simplex (s. s.), Ph. decipiens (s. s.), Ph. krabbei to be discriminated from non‐zoonotic species, i.e. Hysterothylacium aduncum (Paoletti et al., 2018).
A loop‐mediated isothermal amplification (LAMP) assay, developed for use in industry, discriminates larvae of Anisakis from Phocanema and Hysterothylacium genera. It has some advantages as it is faster than conventional PCR or qPCR and does not require reagents and temperature control devices as required by the former (Cammilleri et al., 2020). However, this LAMP assay is not able to identify the isolated parasites to the species level. Finally, recombinase polymerase amplification‐SYBR Green (RPA‐SG) uses ITS locus to differentiate between A. simplex, A. pegreffii, A. typica, but achieves sensitivity of 102 copies per reaction (qPCR) in 20min at 37°C (Chen et al., 2022). A SYBR‐Green uses mtDNA cox2 was developed to detect A. simplex (s. l) DNA in commercial fish‐derived food (Godinez‐Gonzales et al., 2017).
For trematodes, PCR‐based techniques to detect the development stages of the liver flukes in snail and fish infections have been described. For example, PCR methods targeting nuclear and mitochondrial genes are available for the detection of O. viverrini metacercariae in fish meat and C. sinensis metacercariae in fish (EFSA BIOHAZ Panel, 2010). Primers to be used for the identification of a range of opisthorchiid and heterophyid species are described in Duan et al. (2021). The European opisthorchiid species isolated from fish fillets O. felineus can be identified by PCR amplification of mtDNA cox1 locus (Caffara et al., 2017). Subsequently, a multiplex PCR was developed to identify European opisthorchiids and heterophyids isolated from fish such as O. felineus, P. truncatum, Metorchis spp., Metagonimus spp. and Apophallus spp. using 18S, ITS rDNA, mtDNA cox1 (Caffara et al., 2020). Multiplex PCR (rDNA 18S locus and mtDNA cytb locus) has also been developed for the identification of C. formosanus in fish (Jaruboonyakorn et al., 2022). Molecular tools for the identification of opisthorchioidea in general have been reviewed by Duflot et al. (2021).
For cestodes, as for other parasites, the intermediate stage plerocercoids detected by microscopic examination of compressed fish flesh or organ samples can be identified at species level by molecular methods. Conventional PCR with subsequent sequencing, and qPCR are mostly used. PCR primers for amplification of target sequences of cestodes were provided by Waeschenbach and Littlewood (2017) and Setyawan et al. (2019).
The methods with their advantages and disadvantages are summarised in Table 4. The molecular identification of isolated parasites from fishery products has been significantly improved by the application of PCR and sequencing methods targeting both nuclear and mitochondrial sequences in the parasites. These molecular approaches applied together with the use of microscopic identification techniques should be regarded as the most reliable identification methods. By using these techniques, it is now possible to obtain a high degree of specific identification of isolated parasites.
3.2.2. Diagnostic methods that may be available in the near future
Diagnostic methods that are currently used in research but which may find greater application in the European Union Reference Laboratory for Parasites (EURLP), National Reference Laboratories and the industry in the future are shown in Tables 4, ,55.
TABLE 5
OMICs technologies.
Methods included in EFSA BIOHAZ Panel, 2010 | Advances since 2010 | Advantages | Disadvantages | Parasites | Main reference(s)/links | |
---|---|---|---|---|---|---|
1. Genomics | Sanger sequencing of A. simplex, A. simplex (s. s.) | Updated whole genome sequencing (WGS) of A. simplex (s. l.) and A. pegreffii | Better genome annotation and completeness due to assembly of short and long reads | Requires training for bioinformatic analyses; requires availability of closely related reference genomes for better annotation | All parasites | NCBI Bioprojects PRJNA16672 (registered in 2006); PRJEB6697 (in 2014); PRJEB496 (in 2018); PRJNA878701 (in 2024) (Mladineo, 2022) |
2. Metagenomics | N/A | Metabarcoding of 18S locus, combined with CRISPR‐Cas9 technology to deplete unspecific reads (e.g. host, microbiota) | Detects gastro‐intestinal parasitome: standardised by mock community test; patented | Requires training for bioinformatic analyses | Helminths, protozoans and microsporidia | Goldberg and Owens (2024) |
3. Proteomics | N/A | MS, CID (collision‐induced dissociation), LC–MS/MS introduced after 2015 to detected Anisakis allergens in fishery products (research purposes only) | High sensitivity; potential to use machine learning algorithms to enhance the identification process (in particular when biosample contains both the host and parasite) | Requires hardware, training and further standardisation (i.e. validation by molecular identification); challenging for closely related species; uniform protocol for sample preservation and for protein extraction, creation of a universal database for a wide range of parasites | Nematodes, other helminths | Fæste et al. (2016), Sy et al. (2022) |
Tandem Mass Tag (TMT)‐based quantitative proteomics | Identification of specific proteome signature of L3 versus L4 of A. simplex | Same as above | Stryiński et al. (2019) | |||
MALDI‐TOF MS: profiling of Anisakis proteome to construct the first library | High sensitivity | Same as above | Marzano et al. (2020) | |||
LC‐ESI‐MS/MS: quantitative proteomics with iTRAQ labelling, shotgun proteomics approach to evaluate protein species obtained from the above liquid chromatography‐mass spectrometry (LC‐MS/MS) using LTQ XL™ Orbitrap XL coupled to a nanoHPLC system | Useful for the proteomic profiling of: (1) A. simplex, A. pegreffii and their hybrids, (2) microvesicles released by A. pegreffii L3 in vitro cultured | Same as above; testing in natural infections in fish hosts | Arcos et al. (2020), Palomba, Rughetti, et al. (2023) | |||
4. Transcriptomics | N/A | RNA‐Sequencing (RNAseq) of anisakids (A. pegreffii, A. simplex, A. simplex × A. pegreffii hybrids, C. osculatum), D. dendriticus. | Infers parasites' molecular identity and functional signature | Requires training for bioinformatic analyses; availability of closely related references genomes | All parasites | Baird et al. (2016), Llorens et al. (2018), Cavallero et al. (2018, 2020), Nam et al. (2020), Trumbić et al. (2021), Palomba et al. (2019, 2022); Palomba, Cipriani, et al. (2020); Palomba, Libro, et al. (2023), Sidorova et al. (2023) |
Abbreviations: CID, collision‐induced dissociation; CRISPR‐Cas9, clustered regularly interspaced palindromic repeats ‐Cas9 technology; LC‐ESI‐MS, liquid chromatography‐electrospray ionisation‐mass spectrometry; LC‐MS, liquid chromatography‐mass spectrometry; MALDI‐TOF MS, matrix assisted laser desorption ionisation‐time of flight mass spectrometry; MS, mass spectrometry; NA, non available; NCBI, National Center for Biotechnology Information; NGS, next generation sequencing; TMT, tandem mass tag.
3.2.2.1. Future methods to visualise and detect parasites
These are physical methods, designated as optical sensing technologies that rely on high‐technology imaging (Table 4), such as magnetic resonance imaging (MRI), hyperspectral imaging, ultrasound and X‐ray imaging (Table 5). They are non‐destructive and have been tested under industry conditions, but have been mostly used for nematodes. MRI can be used in live fish, detecting the accumulation within a tissue/body cavity and movements of A. simplex. It is specific to genus level, but the image acquisition time is relatively long (13 min/fillet) (Bao et al., 2017). Hyperspectral imaging has a faster processing time (1 s/fillet), and improvements in illumination have enabled a significant increase of the signal to noise ratio. However, it requires more standardisation as the detection rate depends on storage time and sample handling (Sivertsen et al., 2011, 2012; Xu et al., 2023). Dual energy computed tomography (CT) detects nematodes deep in the muscle, but the disadvantage is that it takes a relatively long time. Ultrasound has proven to be less applicable, as it produces noisy images, with no contrast between the nematode and muscle (Heia et al., 2017). All of the listed techniques require equipment and trained staff, and the specificity is at best to the genus level (Bao et al., 2017; Heia et al., 2017). Hyperspectral imaging for example, detects 70.8% of the dark and 60.3% of pale nematodes (Sivertsen et al., 2012). Future development of equipment and optimisation of the methodology may make these technologies more applicable for the industry.
3.2.2.2. OMICs for the detection and identification of parasites and their molecules
These methods include genomics, metagenomics, proteomics and transcriptomics (the methods with their advantages and disadvantages are shown in Table 5). They are sensitive, reliable and robust but the interpretation of the results requires reference databases. However, they are not routinely used for species identification, because they require investment in equipment and well‐trained staff for both data generation and analysis. There are publicly available data sets against which particular targets can be annotated and referenced (e.g. GenBank from the National Center for Biotechnology Information (NCBI), WormBase, European Nucleotide Archive (ENA)). OMICs methods are usually not validated across large number of samples or species, but with optimisation, they can be applied to most parasites. Their advantage is that while they enable parasite identification, they also facilitate identification of parasites' molecules, such as allergens, antigens or other bioactive compounds. These will be useful for the future development of reliable diagnostic/identification tools.
Genomics: Genome data are not primarily used to identify the species as simpler and fast molecular tools have been developed to achieve this (see above), but it provides a useful resource for the development of further molecular/genetic markers (such as SNPs; ddRAdseq analysis) to be used for the identification and genetic characterisation of target zoonotic parasites as well as for potential drug targets. The first available draft genome of A. simplex (s. l.) was published in 2006 (NCBI BioProject PRJNA16672), and subsequently updated in 2014 and 2018 (PRJEB6697 and PRJEB496, respectively). The genome of A. pegreffii has been also recently sequenced (Mladineo, 2022) (PRJNA878701, registered in 2024).
Metagenomics: Although no metagenomic studies have been performed targeting the whole genome of a community of zoonotic parasites in fish, a deep sequencing of an 18S rRNA gene locus has been developed for three groups of parasites. This approach capitalising on metabarcoding and CRISPR‐Cas9 technology has been developed and validated to detect helminths, protozoan and microsporidia parasites in a host gastro‐intestinal tract (parasitome) (Goldberg & Owens, 2024, 29 ). The method has been standardised using a mock community test reagent and the interfering host signal has been reduced using CRISPR‐Cas9 digestion with host‐specific guide RNAs. The patented method can be used for the detection of multiple parasite taxa from fishery products in the future.
Transcriptomics: The RNA‐sequencing has been mostly used to catalogue gene expression in zoonotic parasites in different conditions and from different parasite tissues and life stages, particularly to identify targets involved in the pathogenicity of A. pegreffii and A. simplex (s. s.) (Baird et al., 2016; Cavallero et al., 2018, 2020, 2022; Llorens et al., 2018; Nam et al., 2020; Palomba et al., 2019, 2022; Palomba, Cipriani, et al., 2020; Trumbić et al., 2021). These data could be used for parasite identification. De novo transcriptomics was performed in other anisakids of the genus Contracaecum, i.e. C. osculatum sp. D (Palomba, Libro, et al., 2023). In cestodes, transcriptomics has been performed to date in plerocercoid and the adult stage of Dibothriocephalus dendriticus (Sidorova et al., 2023).
Proteomics: Proteomic profiling of anisakids preceded the application of transcriptomics, capitalising on techniques well advanced for use in human pathogens. The first profiling of A. simplex (s. s.) and A. pegreffii was done using the MALDI‐TOF/TOF MS approach (Arcos et al., 2014). This was followed by mapping of allergens in the Anisakis proteome using the nano LC‐ESI‐MS/MS technique (Fæste et al., 2014), quantification of A. simplex proteins in food matrix by LC‐MS/MS (Fæste et al., 2016), fast monitoring of parasites in fish products using parallel reaction monitoring (PRM) MS (Carrera et al., 2016) and quantification of proteomes of A. simplex s.s., A. pegreffii and their hybrids using iTRAQ labelling and subsequent nano LC‐ESI‐MS/MS (Arcos et al., 2020). Shotgun sequence identification of L3 and L4 proteins based on isobaric mass labelling (Tandem Mass Tag, TMT) (Stryiński et al., 2019, 2022) was then undertaken, followed by the construction of a spectral library of Anisakis spp. using MALDI‐TOF MS supported by LC‐ESI‐MS/MS (Marzano et al., 2020). Shotgun analysis, with LC‐MS/MS coupled to a nanoHPLC and bioinformatic analysis allowed to profiling protein content of extracellular vesicles (EVs) of L3 A. pegreffii (Palomba, Rughetti, et al., 2023). Lastly, targeted proteomics was employed to identify shared allergens between A. simplex and Ph. decipiens (Saelens et al., 2022). To date, MALDI‐TOF MS is not routinely used to identify zoonotic parasites infecting fish, because other methods are more user‐friendly and there is a lack of comparative reference data for all zoonotic parasites.
3.2.2.3. Outlook on future developments in visualisation, isolation and identification methods for zoonotic parasites in fishery products
Advances in high‐throughput sequencing methods, combined with the computational biology and bioinformatics, have allowed new discoveries in both basic and applied research of anisakid parasites, mainly in the two zoonotic parasites A. simplex (s. s.) and A. pegreffii. This resulted in the discovery of parasites' gene transcripts and proteins attributed to the particular parasitic developmental stage, as well as host’ markers involved in the interaction with natural and accidental hosts (Bušelić et al., 2018; Nam et al., 2020; Palomba, Cipriani, et al., 2020; Palomba, Rughetti, et al., 2023; Trumbić et al., 2021). Research is required to obtain new insights into the host–parasite interaction, in particular the characterisation of molecules that have a differential immunogenic and pathogenic role in accidental human infection, such as antigens and allergens, to support the future development of reliable diagnostic tools.
Artificial intelligence (AI) and machine learning methods (e.g. deep learning) have been successfully used in various fields of parasitology including parasitic disease diagnostics as well as parasite and vector species identification (Dantas‐Torres, 2023). AI‐based algorithms in image or video processing are useful tools for parasite species identification and infection level assessment in parasitic disease examinations (Li et al., 2023; Wąsikowska et al., 2018; Wąsikowska & Linowska, 2021).
3.2.3. Concluding remarks for ToR2 AQ2
AQ2. What testing methods are currently available and may be available in the near future to test the fish species for the parasites identified in the answer to ToR1?
The methods that were described in the previous EFSA BIOHAZ opinion (2010) are still in use for the detection of zoonotic parasites in fishery products, and include visual inspection including candling, artificial digestion and the UV‐press method. The UV‐press and artificial digestion methods have been standardised by the ISO (ISO 23036‐1:2021 and ISO 23036‐2:2021 standards, respectively).
Since the last EFSA opinion (EFSA BIOHAZ Panel, 2010), new technologies and methodologies have been developed for the detection, visualisation and isolation of zoonotic parasites in fish products and for the specific identification of the isolated parasites.
New UV‐scanning devices have been developed and are in use for the detection of Anisakis in fishery products. However, to date these scanning techniques have not been fully validated for all types of zoonotic parasites in fishery products. Novel optical (hyperspectral) sensing methodologies have also been developed and these have found limited application for testing fish under commercial conditions. Future development of equipment and methodology may make these technologies more widely applicable.
The molecular identification of isolated parasites from fishery products has been significantly improved by the application of PCR‐amplification and sequencing methods targeting both nuclear and mitochondrial gene loci in the parasites. These genetic/molecular approaches (applied together with the use of microscopic identification techniques) are currently regarded as the most reliable identification method.
OMICs (genomics, metagenomics, transcriptomics and proteomics) generated data are a useful resource for the selection of further molecular/genetic markers to be used for the identification and characterisation of zoonotic parasites.
The application of artificial intelligence and machine learning algorithms in image and video processing are also being tested for the high throughput detection and identification of parasites in fish.
3.3. ToR3: Technical developments and new scientific data available in relation to killing and/or removal of viable parasites of public health importance in fishery products, in particular treatments other than freezing
AQ3. What technical developments and new scientific data for inactivation and/or removal of viable parasites (identified in the answer to ToR1) in fishery products, in particular treatments other than freezing, have been described since the EFSA BIOHAZ 2010 scientific opinion?
The EFSA BIOHAZ Panel (2010) discussed several physical and chemical treatments for inactivating parasites in fishery products. Since then, more data have been gathered in relation to those treatments already covered in that opinion (see Section 3.3.1) and some new technologies and traditional preservation methods have been used to explore the possibility of adopting alternatives to freezing. The end point used in most inactivation studies is the loss of larvae viability (which, for anisakids, was defined by EFSA as the ability of individual larvae to survive various chemical and physical treatments or processing procedures). New procedures to assess larval inactivation, including the use of devices with the potential of high throughput screening, have been explored. Moreover, methods for removing parasites from the fish have also been investigated.
Since 2010, treatments for inactivation of parasites have been studied for nematodes of the family Anisakidae (mostly for Anisakis spp.), but less for other nematodes, cestodes and trematodes.
These have been covered in several reviews (e.g. Della Morte et al., 2023; Franssen et al., 2019; Fu et al., 2023; Kuchta et al., 2015; Liu et al., 2022; Martínez et al., 2023; Pozio et al., 2013; Tavares‐Dias, 2018; Valero et al., 2015).
In this section, a summary of the EFSA BIOHAZ Panel (2010) scientific opinion is firstly presented (Section 3.3.1). The current state of the art in terms of the methodology and approaches for assessing parasite inactivation is subsequently described (Section 3.3.2). Thereafter, new data, gathered since the previous opinion on the application of physical treatments (other than freezing) (Section 3.3.3), chemical treatments (Section 3.3.4) and general considerations for physical and chemical treatments (Section 3.3.5) are presented and finally, strategies for physical or chemical removal of parasites from fishery products are described (Section 3.3.6).
3.3.1. Summary of methods for the killing of viable parasites of public health importance in fishery products as discussed in the EFSA BIOHAZ Panel (2010) scientific opinion
A summary of the methods for killing viable parasites of public health importance in fishery products as discussed in the EFSA 2010 scientific opinion is presented below (EFSA BIOHAZ Panel, 2010).
Freezing is effective in killing parasites in fish. The lower the freezing temperature and the longer the storage time at this temperature, the lower the probability of survival of parasites. For example, at −10°C anisakids can survive for days, but at −35°C, the survival period is reduced to a few hours. Other factors reported to affect the mortality of anisakids is the time to reach the final freezing temperature and the fat content of the fish. For cestode larvae, Diphyllobothrium plerocercoid is inactivated in a household freezer when the fish is frozen for at least 1 day at −18°C. Clonorchis and Opisthorchis metacercariae are inactivated when fish is kept at −10°C for 5days. Depending on the freezing conditions, it may take days for all parts of the fish to reach a lethal temperature and it is important to stress that the target temperature must be reached at the core of the sample (i.e., warmest location) and that one‐ and two‐star domestic freezers (i.e. operating at −6°C and
−
12°C, respectively) are not suitable for freezing fish in order to kill nematode parasites.
Reaching >60°C (core temperature) for 1 min was considered the minimum heat treatment required to kill all anisakids larvae in fishery products by conventional heating. The time to reach this core temperature is affected by the product thickness and composition. For microwave heating, it was reported that the fillet should reach at least 74°C for at least 15 s. Heating above 56°C inactivated Diphyllobothrium plerocercoids, and for inactivation of free O. viverrini metacercariae, time–temperature combinations of 5 h at 50°C and 30 min at 70°C were found to be sufficient. Based on these data, the EFSA BIOHAZ Panel (2010) concluded that for A. simplex larvae, equivalent lethal effects to −20°C for 24 h in all parts of the product could be attained at time–temperatures of −15°C for at least 96 h or
−
35°C for at least 15 h, or heating at ≥
60°C for at least 1 min in the thermal centre of the fish product. However, the report stressed that these treatments may not inactivate allergens.
High‐pressure processing (HPP) 30 can kill A. simplex larvae, but for some applications, the long treatment times required to kill all larvae, e.g. 200 MPa for 10 min or 140 MPa for 60 min, were considered impractical for the food industry (EFSA BIOHAZ Panel, 2010). In some fish species, effective combinations for killing A. simplex larvae (i.e. 414 MPa for 30–60 s; 276 MPa for 90–180 s; and 207 MPa for 180 s) caused changes in the muscle colour and appearance which may limit the application of this technology. Nevertheless, it was suggested that 300 MPa for 5 min could be effective in the treatment of mackerel and other fatty fish species.
No information was found for the effect of drying. Regarding irradiation, A. simplex is highly resistant to the doses that were acceptable for fishery products (i.e. doses up to 3 kGy), whereas for metacercariae of O. viverrini and C. sinensis, low‐dose irradiation of freshwater fish was sufficient to inactivate them or prevent infectivity (EFSA BIOHAZ Panel, 2010). It had been claimed that low voltage current inactivates A. simplex larvae in fish but scientific studies to validate this were not available. A. simplex are not able to survive hot smoking since products are exposed to temperatures well above 60°C for 3–8 h. However, cold smoking, in which temperatures are kept below 38°C for a few hours up to several days, was not sufficient to inactivate A. simplex larvae (EFSA BIOHAZ Panel, 2010).
Salting can, to some extent, inactivate anisakids providing that the salt concentration in brines reaches up to 8%–9% for at least 6weeks, whereas for dry salting, 20
days are sufficient to kill the larvae in herring. Salting at 13.5% NaCl for 24 h inactivates Opisthorchis metacercariae in fish. Marinating conditions of some traditional products are not sufficient to kill A. simplex larvae, and depending on the salt concentrations, they can survive from 35 to 119
days. EFSA BIOHAZ Panel (2010) concluded that the risk posed by A. simplex could be reduced with vinegar and salt, but in some marinated products, these ingredients failed to reduce the parasites in a sufficiently short time, and it is therefore necessary to freeze the products prior to marinating. The EFSA BIOHAZ Panel (2010) also reported other chemical procedures effective in killing Anisakis, these included the use of shogaol and gingerol extracted from Zingiber officinale, components of Perilla leaves or monoterpenic derivatives from essential oils, with alpha‐pinene being the most active. The BIOHAZ Panel suggested that, since the efficacy of chemical treatments depended on factors such as size, fat content and active ingredients, the procedures would have to be optimised for each culinary preparation, if not combined with freezing.
The conclusions of the EFSA BIOHAZ Panel (2010) opinion were considered for modifying part D of Annex III, Section VIII, Chapter III to Regulation (EC) No 853/2004 (Commission Regulation (EU) No 1276/2011) as stated in Section 1.1.
3.3.2. Methods and approaches to evaluate parasite inactivation
To assess the efficacy of a specific treatment of fishery products, it is crucial to determine the post‐treatment inactivation of the infective parasites. Inactivated larvae are considered to have lost their capacity to attach onto the gastric or intestinal mucosa, and to penetrate into the submucosa causing phlegmon–abscess and granuloma in the accidental host (Romero et al., 2013). The different methods to evaluate the inactivation after treatment are summarised below and shown in Table 6.
TABLE 6
Methods for evaluating parasite inactivation.
Inactivation method | Target parasites a | Characteristics/comments | References (examples) |
---|---|---|---|
Bioassay | Clonorchis sinensis metacercariae | Rats; rabbits; Guinea pigs; albino mice | Franssen et al. (2019) and references therein |
Opisthorchis viverrini metacercariae | Hamsters; rabbits; cats | Franssen et al. (2019) and references therein | |
Dibothriocephalus spp. plerocercoid larvae | Golden hamster | Franssen et al. (2019) and references therein | |
Anisakis | Rats | Franssen et al. (2019) and references therein | |
Anisakis | Sprague–Dawley rats | Zuloaga et al. (2013) | |
Contracaecum osculatum | Pigs | Strøm et al. (2015) | |
Anisakis | Wistar rats | Romero et al. (2013), Morsy et al. (2017) | |
Opisthorchis viverrini metacercariae | Male golden Syrian hamsters | Onsurathum et al. (2016) | |
Viability – visual inspection | Anisakis | Measurements of spontaneous and stimulated movements: immediately, and after incubation with 0.85% NaCl for 10 min at 37°C and after 4 h and 24 h at room temperature | Sánchez‐Alonso et al. (2020) |
Anisakis | Immediately, after incubation in a thermoblock at 37°C for 15 min | Łopieńska‐Biernat et al. (2020) | |
Anisakis | Measurements of spontaneous and stimulated movements: stored after treatment at 37°C for 10 min for recovery. Those showing no movement, gently touched ~![]() | Lee et al. (2016) | |
Anisakis | For those showing no spontaneous movement, 3‐min videos were recorded immediately after treatment and at intervals during a 2‐h period | Vidaček et al. (2011) | |
Anisakis | Measurements of spontaneous and stimulated movements: Viability scores: 3 – mobile; 2 – reduction of mobility; 1 – mobility only after stimulation, and 0 – death | Trabelsi et al. (2019) | |
Anisakis | Observations at 24‐ and 48‐h post‐treatment | Onitsuka et al. (2022) | |
Anisakis | Candling table under stereomicroscope: displacement from the inoculation point (in/out of the fillet, coiled/stretched, motility) | Pascual et al. (2010) | |
Separation of larvae & Viability – visual inspection | Anisakis | Artificial digestion to extract larvae from tissues and measurement of spontaneous and stimulated movements. The number of total and viable larvae may be underestimated if performed after treatments such as freezing | Sánchez‐Alonso, Rodríguez, Tejada, et al. (2021) |
Visual inspection – UV light | Anisakis | Fluorescence under ultraviolet light source (366 nm) held 15 cm above the larvae. Emission of fluorescence was recorded immediately and at 1 and 2 h after each treatment. The intensity of the fluorescence rated in arbitrary units as maximum, medium, slight or no fluorescence. Not always inactivated larvae emit fluorescence | Vidaček et al. (2011) |
Viability instrumental device | Anisakis | Instrumental device to measure viability: by near‐infrared imaging, elastic curvature energies and geometric shape parameters are determined | Kroeger et al. (2018) |
Parasites | Whole organism phenotypic analysis with devices with medium‐ to high‐throughput screening potential | Herath et al. (2022) and references therein | |
Caenorhabditis elegans, applicable to other species | Device medium‐ to high‐throughput screening potential: Infrared Light Locomotion Tracking (ILT) system | Simonetta and Golombek (2007) | |
H. contortus, applicable to other species | Device medium‐ to high‐throughput screening potential: ILT system; conditions adapted to shorten the time required for data acquisition from i.e. 3 h to 15 min | Taki et al. (2021) | |
Anisakis | Instrumental device to measure mobility: ILT system, measurements for up to 100 min | Ogata and Tagishi (2021) | |
Anisakis | Instrumental device to measure mobility: ILT system; conditions adapted to measure recovery (measurements for up to 46 h) | Guan, Usieto, Cobacho Arcos, et al. (2023) | |
Agar penetration test | Anisakis | Migration into agar counted after incubation: 0.5%–1.5% agar, roughened surface, herring blood on top of the agar, incubation at 37°C for up to 3![]() | Ruitenberg (1970) |
Anisakis | Migration into agar at different concentrations, counted after incubation: Agar at 0.5%, 0.75% and 1.0% with 0.4% NaCl. 10 mL of each of solutions poured into 20 mL screw‐cap glass bottles. Larvae placed on the surface and the penetration rates measured after 1 h | Suzuki et al. (2010) | |
Anisakis | Migration into agar counted after incubation: L3 immersed in 10 mL of artificial gastric fluid (pH 1.8), placed on top of a solid agar (3 cm depth, 0.75% agar and 0.9% NaCl), incubated at 37°C, 5% CO2. Migration counted after 24 h | Arizono et al. (2012), Sánchez‐Alonso et al. (2018) | |
Anisakis | Migration into agar counted after incubation: 4 mL of agar solution (1% agar in RPMI‐1640 medium, with 20% fetal bovine serum), 100 μL of supernatant, RPMI‐1640 (RPMI‐1640, 20% FBS, 1% commercial pepsin, pH 4.0), was placed into each well. Incubation at 37°C in 5% CO2 and migration counted after 1, 12 and 24 h of incubation | López et al. (2019) | |
Agar test – mobility and motility |
Anisakis Phocanema | Measurement of larval motility (i.e. in situ movement of a larva): video recording for up to 30 min. Larvae figuratively divided into three parts; scores (0–3) to monitor body motility in 1 min in sections of larvae | Guan et al. (2021) |
Anisakis Phocanema | Measurement of larval mobility (i.e. the total distance that a larva migrated within the medium): Video recording for up to 30 min. Migration distance measured | Guan et al. (2021) | |
Resistance to incubation in artificial gastric juice | Anisakis | Mortality of larvae in artificial gastric fluid: mortality of larvae measured upon incubation in 20 mL of artificial gastric fluid (0.1% pepsin, 0.1% porcine stomach mucin, 0.12% NaCl, 0.02% KCl, pH 1.8) at 37°C 5% CO2. The medium was exchanged every 24 h | Arizono et al. (2012), Sánchez‐Alonso, Carballeda‐Sangiao, Rodríguez, et al. (2021) |
Respiration analysis | Anisakis | Oxygen consumption rates in whole specimens with a single chamber Clark electrode: basal (in M9 buffer), maximum (M9 buffer with FCCP‐(carbonyl cyanide 4‐trifluoromethoxy) phenylhydrazone addition) and residual (with sodium azide) respiration capacities measured | Sánchez‐Alonso et al. (2019) |
Other methods | Anisakis | Stress protein levels; dyes (e.g. methylene blue) | Franssen et al. (2019) and references therein |
Abbreviations: FCCP, carbonyl cyanide 4‐trifluoromethoxy; ILT, infrared light locomotion; KCl, Potassium chloride; RPMI‐1640, cell culture medium, i.e. Roswell Park Memorial Institute medium; UV, ultraviolet.
3.3.2.1. Bioassays
Bioassays are considered as the gold standard (Franssen et al., 2019) and experimental laboratory animals have been used to study the pathological reactions related to Anisakis, Phocanema and C. osculatum infections. Recent examples include studies of Anisakis larvae (Morsy et al., 2017; Romero et al., 2013; Zuloaga et al., 2013) or third‐stage C. osculatum larvae (Strøm et al., 2015). Bioassays have also been used for the study of C. sinensis metacercariae and Dibothriocephalus spp. (syn. Diphyllobothrium) plerocercoid larvae (Franssen et al., 2019) and O. viverrini metacercariae (Onsurathum et al., 2016). However, to reduce the use of experimental animals, in vitro assays have been adopted to replace the in vivo methods (Franssen et al., 2019). Indicators of behaviour, physiology, metabolism or physical damage of the parasite can be good alternatives for assessing inactivation.
3.3.2.2. Loss of larval mobility – Visual determination
The survival of anisakid larvae to different treatments is usually measured by their viability. A viable larvae is physically intact and motile measured by its ability to move spontaneously or after stimulation with tweezers and a needle (EFSA BIOHAZ Panel, 2010). This has been assessed under natural or UV light (Vidaček et al., 2011), at 37°C or at room temperature (Łopieńska‐Biernat et al., 2020; Sánchez‐Alonso et al., 2020), immediately or 24 or 48 h after a treatment (Onitsuka et al., 2022; Sánchez‐Alonso et al., 2020). Some authors specify the number of assessments (i.e. times showing no movement after stimulation) for a larva to be considered as dead (Lee et al., 2016), or use a video camera to record movements for a subsequent visual observation (Vidaček et al., 2011), while others have adopted viability scores (e.g. Trabelsi et al., 2019). With the exception of a few examples, where motility has been monitored directly, i.e. by candling (Pascual et al., 2010), the assays are usually performed on isolated larvae, either after manual extraction from the tissues, or by means of the artificial digestion method (Section 3.2.1.1). The latter may underestimate the number of mobile larvae and this limitation needs to be taken into account when designing inactivation protocols (Sánchez‐Alonso, Rodríguez, Tejada, et al., 2021).
3.3.2.3. Loss of larval mobility – Instrumental methods
Slow movements exerted by the larvae may not be detected by the observers (Kroeger et al., 2018) and visual inspection can be tiring and time consuming, since larva may remain motionless for long periods (Guan, Usieto, Cobacho Arcos, et al., 2023).
One technological solution that may be used instead of visual observations was developed for anisakid nematodes by Kroeger et al. (2018). Using near‐infrared imaging, the device measures elastic curvature energies and geometric shape parameters determined from contours, and these are used as a measure of viability. Authors reported that the device differentiated individual living and dead larvae isolated from marinated, deep‐frozen and salted products.
The need for the discovery of anthelminthic drugs for treating several parasitic infections has led to the development of medium‐ to high‐throughput phenotypic screening platforms which can assess not only worm motility but also a series of physiological, metabolic and morphological indicators (for review, see e.g. Herath et al., 2022). In particular, a ‘worm tracking’ commercial system which measures the interference of an infrared light beam by worm movement has been developed (Simonetta & Golombek, 2007), adapted for faster data acquisition (Taki et al., 2021) and applied to study Anisakis larvae (Ogata and Tagishi, 2021; Guan, Usieto, Cobacho Arcos, et al., 2023). Although these methods need to be tested on a larger number of samples including different anisakids or zoonotic parasites species, they may be used for high‐throughput screening in the future.
3.3.2.4. Loss of larval function other than mobility
The agar penetration test can be applied to substitute in vivo assays as there are good correlation of this in vitro method with bioassays (Ruitenberg, 1970). A similar observation was made in a study examining the differences in the penetrative ability of two Anisakis species (Arizono et al., 2012; Suzuki et al., 2010), which were independently corroborated with in vivo studies (Romero et al., 2013). Over the years, some modifications of the method have been adopted (Arizono et al., 2012; López et al., 2019; Sánchez‐Alonso et al., 2018; Suzuki et al., 2010). Other authors measured mobility, as the total distance that the larva migrated inside the agar medium, and motility, as the in situ movements of the larvae into the agar (Guan et al., 2021). In addition, indicators such as the mortality of the larvae upon incubation in an artificial gastric fluid (Arizono et al., 2012; Sánchez‐Alonso, Carballeda‐Sangiao, Rodríguez, et al., 2021), respiratory analysis of intact larvae (Sánchez‐Alonso et al., 2019), the use of dyes, the determination of physiological stress indicators, morphological features and integrity by microscopic examination (Franssen et al., 2019) could all be adopted as complementary measurements, but this would require research on their relationship with parasite infectivity.
At present, the relationship between the survival of the larvae, after a given treatment, and their infection capacity in humans remains unclear (EFSA BIOHAZ Panel, 2010), and as stated in the previous opinion, a precautionary principle is adopted (i.e. all larvae should be dead) which gives a higher margin of safety. However, the new approaches for the measurement of parasite inactivation discussed in this section could lead to the development and application of new indicators of treatment efficacy. High‐throughput methods that assess mobility (or the loss thereof), e.g. will enable operators to rapidly test high numbers of parasites thus facilitating research and the development of monitoring activities that could be applied in industry.
New studies on the factors affecting human host tissues penetration (in the case of infective A. pegreffii L3 in the gastrointestinal tract) will be an important step in understanding the pathogenesis of the disease (Palomba, Rughetti, et al., 2023) and may also provide the scientific basis for new strategies against anisakiasis, including the development of new indicators, e.g. by advanced molecular approaches.
3.3.2.5. Experimental approaches for evaluating parasite inactivation
Table 7 lists the reported physical and chemical treatments for parasite inactivation since the 2010 EFSA BIOHAZ opinion. The application of such treatments has been mostly performed in isolated larvae, in homogenates, in spiked muscle and in products with natural parasitation. Most of the studies were performed under laboratory conditions, with a relatively low to medium total larval sample size (typically from 5 to 35 larvae per assayed condition per experiment). To establish the conditions for complete lethality for a given technology or process with high confidence, inactivation studies with a high number of larvae (in the order of thousands) would be required. Another important consideration is the extrapolation of the results from relatively simple systems to real products (i.e. going from isolated larvae, to spiked products to real foods with naturally parasitised matrices).
TABLE 7
Reported treatments to inactivate parasites.
Treatment | Parasite | Matrix | Treatment conditions a | Parameters for successful treatments b | Comments | References |
---|---|---|---|---|---|---|
| ||||||
Conventional heating | Anisakis simplex (s. l.) | 10 mL of distilled water at room temperature spiked with larvae (N![]() ![]() | Conventional heating using test tubes in a water bath set at 40, 50, 60, 70, 80 and 90°C. Holding times at target temperatures: 30 s to 60 min |
≥ 60°C, 15 min in one batch 60°C, 3 min in the other batch | Time to reach 60°C was around 3 min and the initial time was set when the required temperature was reached | Vidaček et al. (2010) |
A. simplex (s. l.) | Hake (Merluccius merluccius) pieces of fillets spiked with larvae (N![]() ![]() ![]() ![]() | Conventional heating using a water bath set at 93°C. 5 or 3 min holding time in the water bath once sandwiches reached 60°C or 70°C, respectively | With 5‐min and 3‐min holding time after reaching 60°C and 70°C, respectively, all tested larvae were dead | Time to reach 60 and 70°C was 6.5 and 11 min, respectively. No lower temperatures or holding times were assayed | Vidaček et al. (2011) | |
Anisakis type I | 15 mL of prewarmed physiological saline solution with larvae (total N![]() ![]() | Conventional heating of the glass tubes in a water bath set at 45°C, 47°C, 48°C and 50°C |
45°C, ~ 85 min 47°C, ~ 40 min 48°C, ~ 7 min 50°C, ~ | Giarratana et al. (2012) | ||
Depending on the batch: A. simplex (s. s.) (100%–70%); A. pegreffii (0%–24%); heterozygote genotype (0%–6%) | Hake sandwiches (Merluccius merluccius) spiked with larvae (N![]() ![]() |
Conventional heating using various conditions: (a) water bath set at 92°C up to target temperatures, and cooled down in ice water; (b) oven set at 200°C until 50°C was reached in the core, and cooled down in ice water; (c) oven set at 200°C and removal of sandwiches at 7, 10, 15 or 20 min and sandwiches allowed to cool at ambient temperature; (d) water bath set at 60°C or 70°C, and once 58 and 68°C were reached, samples were removed immediately or after 2.5 and 5 min |
By decision tree analysis, ≥ ≥ | None of ≥![]() ![]() | Sánchez‐Alonso, Carballeda‐Sangiao, González‐Muñoz, et al. (2021) | |
Depending on the batch: A. simplex (s. s.), from 100% to 92.5%; A. pegreffii (0%–2.6%); heterozygote genotype (0%–7.5%) | Larvae individually immersed in 30 μL of 0.85% NaCl in 96 microplate wells, per time and temperature condition (N![]() ![]() | Conventional heating at temperatures ranging from 44°C to 61°C in a thermal cycler under ‐quasi‐isothermal conditions, from 0s up to 45 min holding times. Experiments that yielded no survivors were performed in triplicate, at each temperature, to have 288 replicates (96![]() ![]() |
Experimental values: 61°C, 1 s; 59°C, 3 s; 57°C, 10 s; 55°C, 30 s; 52°C, 130 s; 50°C, 300 s; 48°C, 2500 s; 46°C, 8500 s | Mathematical model (i.e. Weibull) explains survival ratio as a function of exposure time; the obtained δT values were used to describe their temperature dependence through a polynomial equation. Good agreement between observed and predicted survival ratios | Guan, Usieto, Sánchez‐Alonso, et al. (2023) | |
Cryptocotyle lingua metacercariae |
Metacercariae were placed between two cod (Gadus morhua) fillet slices (cod sandwich) and in natural parasitised cod. In spiked sandwiches: 10 metacercarial cysts/sandwich, triplicates, 38 cases, 1140 metacercariae analysed. Approx. 5 g of infected cod tissue (skin including 4–6 | Incubation on Petri dishes at different temperatures ranging from 50°C to 100°C from 1 min to 3 h. Petri dishes were supplied with 500 μL of deionised water and a lid in order to avoid desiccation. |
Fish sandwiches: 100°C, 10 min; 90°C, 15 min; 80°C, 15 min; 70°C, 15 min; 60°C, 30 min; 50°C, 2 h. Naturally parasitised fish: 60°C, 30 min; 70°C, 30 min. | Borges et al. (2015) | ||
Microwave | Anisakis sp. | Chilled A. simplex larvae (2.5 g of larvae from heavily parasitised hake (Merluccius merluccius) attached to ovary and viscera tissue, in 4 mL water | Household 900 W microwave oven operating at maximum (100%) power for 30 s. Final temperature of the samples ranged between 67.8°C and 69.2°C | 900 W (100% of total power), 30 s, 67.8–69.2°C as final temperature of the samples | Vidaček et al. (2009) c | |
Anisakis sp. | Fish sandwiches spiked with larvae (N![]() ![]() | Household microwave oven operating on 75% of power (600 W). Samples not covered nor rotated. Temperature was registered after 1 min in microwave, samples were removed and maintained at room temperature (RT) for 15 min | 600 W (75% of total power), 75.6°C after 1 min in microwave followed by 15 min at RT | 10% L3 survived when the final temperature of 64°C (i.e. bigger sandwiches) was reached (after 1 min) | Lanfranchi and Sardella (2010) | |
Aniskakis sp. | Hake (Merluccius merluccius) sandwiches spiked with larvae (N![]() ![]() | Household microwave oven operating at maximum (100%) power for 3 min. Samples covered with microwave‐resistant film | 900 W (100% of total power), 3 min, 66.9°C as final temperature of the samples, then vacuum packed and stored at 5°C for 24 h | Tejada et al. (2006) c | ||
A. simplex (s. l.) | 30 mL of distilled water spiked with larvae (N![]() ![]() | Laboratory microwave digestion oven. The oven was programmed to reach the required temperature in 1 min. The conditions used were 50 and 60°C (at 800 W) for 1, 2, 3, 4, 5, 6, 7, 8, 9 and 10 min and at 70°C (at 1000 W) for 1, 2, 3, 4 and 5 min. Rotated, optimal microwave distribution |
800 W, 60°C, ≥ 1000 W, 70°C, ≥ | Vidaček et al. (2011) | ||
A. simplex (s. l.) | Hake (Merluccius merluccius) sandwiches spiked with larvae (N![]() ![]() | Microwave digestion oven. The oven was programmed to reach the required temperature in 1 min. The conditions used were 800 W, 60°C, 5 min and 1000 W, 70°C, 3 min holding time. Rotated, optimal microwave distribution |
800 W, 60°C (reached after 1 min), 5 min holding time; 1000 W, 70°C (reached after 1 min), 3 min holding time | Vidaček et al. (2011) | ||
High‐pressure processing | A. simplex (s. l.) | 5 mL of distilled water spiked with larvae (N![]() ![]() |
100, 200, 300, 350 MPa for: (a) 1 min; (b) two cycles of 1 min separated by 5 min [i.e. 1 (c) 2 min; (d) two cycles of 2 min separated by 5 min [i.e. 2 | ≥![]() ![]() | Alterations in larvae body and ruptures in the cuticle by scanning electron microscopy (SEM) | Vidacek et al. (2009) c |
A. simplex (s. l.) | Hake (Merluccius merluccius) steaks spiked with larvae (N![]() ![]() | 200 MPa, for (a) 1 min, (b) 2 min, (c) two cycles of 2 min separated by 5 min [i.e. 2![]() ![]() | ≥![]() ![]() | Alterations in larvae body and ruptures in the cuticle by SEM. However, visual changes in appearance only observed at 300 MPa | Vidacek et al. (2009) c | |
A. simplex (s. l.) | White spotted conger (Conger myriaster) spiked with larvae (N![]() ![]() | The pressure vessel was preheated to the desired temperature and pressurised for 1 or 5 min (holding time) at 150 or 200, and for 1 min (holding time) at 250 or 300 MPa. The pressure build‐up velocity was 5 MPa s–1, and the decompression time was <![]() |
200 MPa, 5 min 300 MPa, 1 min | No significant changes in sensory analysis nor in colour measured instrumentally at 200 MPa for 5 min, while low sensory scores were obtained at 300 MPa for 1 min | Lee et al. (2016) | |
Pulsed electric fields | Anisakis (presumably A. pegreffii) | Fillets of horse mackerel (Trachurus japonicus) spiked with larvae in the middle of the fillet (N![]() ![]() |
PEF chamber, capacity 40 μF and 80 μF charging voltage, 15 kV and 4.5 and 9.0 kJ stored energy/pulse, 11 cm distance between parallel plate electrodes (a) Buffer saltwater conductivity (0.2–11.5 mS/cm). Pulsed currents applied to 5.5 kg fillets (about 250 fillets). Charging voltage to the capacitor fixed at 15 kV, capacitance, 40 μF, 1 Hz operating frequency, 500 shots. Immersed in buffered NaCl; initial Tª 5°C, for 10 min. (b) 7 kg fillets, 5 mS/cm, 15 kV, 80 μF, 1 Hz, 100–500 shots. Initial Tª 6°C | Salt water conductivity 5 mS/cm, charging voltage 15 kV, capacitance 80 μF, frequency 1Hz, 500 shots | Sensory assessment confirms that the product retains its quality as sashimi | Onitsuka et al. (2022) |
Anisakis spp. | Saline solution with an electrical conductivity of 1.3![]() ![]() ![]() ![]() | PEF chamber using processing conditions: electric field strength 1–3 kV/cm, specific energy 3–50 kJ/kg, pulse width from 3 to 100 μs. Response surface methodology used to determine optimal treatment |
Viability highly dependent on electric field strength, specific energy and the influence of pulse which was higher at lowest field strengths. 3 kV/cm and 50 kJ/kg provided the best processing conditions Second‐order polynomial equation fitted well the experimental data. Validation experiment rendered good agreement with predicted mortality However, no complete inactivation was reached (i.e. 95%) | Abad et al. (2023) | ||
Anisakis spp. | Hake (Merluccius merluccius) sandwiches spiked with larvae (N![]() ![]() | PEF chamber using processing conditions: electric field strength 1–3 kV/cm and pulse width 30 μs, specific energy 50 kJ/kg |
With 3 kV/cm, 30 μs, 50 kJ/kg, the maximum inactivation was attained No complete inactivation was reached at these conditions in all samples (80%–100%) | Abad et al. (2023) | ||
A. pegreffii |
Fillets of horse mackerel (Trachurus japonicus) (spiked) Chum salmon (Oncorhynchus keta) fillets and slices (spiked) Chum salmon slices (naturally parasitised). In experiments (a) to (e), 10 larvae/fillet, three fillets per condition. In experiments (f) to (h), 10–12 larvae in one position of the fish (estimated total larvae, 219). |
PEF parameters to study: (a) buffer saltwater conductivity, (b) capacitance, (c) charging voltage, (d) input energy per single pulse, (e) distance between parallel plate electrodes. Matrix parameters of study: (f) position of anisakid larvae in fillet, (g) position of larvae in whole fish, (h) natural infection and effect of species. |
Successful conditions for PEF parameters: (a) 5–11 mS/cm at 2.4 μF‐ 30 kV (1.7 J/cc/pulse, total 8.6 J/cc); (b) (e) 20mm, 1.1 J/cc/pulse, 13.8J/cc, 1.6 μF, 30 kV;. Matrix parameters: (g) dorsal, caudal swim blader at 200 shots (137.6 J/cc); (h) block of salmon fillet, 64 pulses, 36.1 J/cc, final temperature 20°C. |
No complete inactivation was achieved in part (c) and (d); the highest immobility (90%) was obtained in (c) at the highest charging voltage (30 kV) at input densities of 0.57 J/cc/pulse, and 23.0 J7cc, 0.8 μF. No complete immobility was found in cut salmon either spiked or naturally parasitised at input energy densities of 36.1 and 42.6 J/cc and final temperature 20°C. | Onitsuka et al. (2024) | |
Other physical methods | ||||||
Drying |
A. simplex (s. s.) (>![]() ![]() | Stockfish, i.e. unsalted, naturally dried cod (natural infection) (Gadus morhua). Total 80 fillets |
Common stockfish production process: (a) 3 months outdoor drying at 1°C; 17 months indoor storage maturation (20 months total time) (b) 3 months outdoor drying, 4.5 months indoor maturation (7.5 months total production time), 10 | The air‐dried stockfish production process with 7.5 months total production time | Larval cuticle damaged showing dehydration effects; larval body partially digested | Bao et al. (2020) |
Ultrasound![]() ![]() | Anisakis | Larvae in tap water (30 larvae in 25 mL); viscera of conger eels (Conger myriaster) naturally infected |
(a) 30 larvae in 25 mL tap water were treated by ultrasound at 37 kHz and 1200 W for 0–30 min; (b) 30 larvae were treated with 25 mL of 500–2000 ppm chlorine solutions for 5 min and then moved into 25 mL tap water and treated by ultrasound at 37 kHz and 1200 W for 0–30 min; (c) Viscera of conger eels naturally infected were treated with 500–2000 ppm chlorine solutions, transferred to tap water and treated by ultrasound at 37 kHz and 1200 W for 5–30 min | (a) 1500 ppm, 2000 ppm chlorine and then, 37 kHz, 1200 W for 30 min | Not successful for viscera of heavily parasitised conger eels | Oh et al. (2014) |
| ||||||
Salting | Anisakis | Larvae (N![]() ![]() | Salting in brine: Salt solution (5, 10, 15, 20% NaCl) for 3 h to 7![]() |
15% NaCl, 7 20% NaCl, 6 | Oh et al. (2014) | |
A. pegreffii | Larvae in different brine solutions. 100 larvae/200 mL solutions in triplicate | Salting in brine: Salt solution (5%, 10%, 15%, 20%, 35% NaCl) stored at 4°C for up to 240 h |
35% NaCl, 3 10%–20% NaCl ~4–5 5% NaCl 10 | Šimat and Trumbić (2019) | ||
A. pegreffii | Anchovies, ungutted (Engraulis encrasicolus) naturally infected | Dry salting: Ungutted anchovies were mixed with NaCl (fish:NaCl ratio![]() ![]() ![]() | ≥![]() ![]() ![]() | Good panel acceptance and good quality grade; further routine analysis on larvae collected from other batches rendered equal results; cuticle damages by SEM | Anastasio et al. (2016) | |
A. pegreffii | Anchovies eviscerated (Engraulis encrasicolus) spiked with larvae in the middle of the fish. Two batches of 100 individual fish spiked with 4 Anisakis larvae in four places per fillet | Dry salting: Alternating layers of fish and NaCl were laid in cans, pressed (10![]() ![]() | Maturation of 10![]() | Šimat and Trumbić (2019) | ||
Anisakis sp.; Contracaecum sp.; Hysterothylacium sp.; Phocanema sp. | Anchovies (Engraulis anchoita) pre‐salted by immersion for 4![]() ![]() ![]() | Double salting (after the pre salting in brine, dry salting): anchovies were salted following local commercial salting techniques using 1 layer of fish – 1 layer of salt, successively from 1 to 24 h. |
1 h dry salting for Hysterothylatium, Contracaecum and Phocanema 16 h dry salting for Anisakis | Time of storage of these salted anchovies is 6–8 months | Lanfranchi and Sardella (2010) | |
Anisakidae larvae, genus: Anisakis (80.5%), Phocanema (19.5%) | Fresh cod fillets (Gadus morhua) naturally infected to produce ‘baccalà’ | Salting in brine plus dry salting: Cod fillets were salted in brine (13% NaCl) at 5°C for 24 h in a 1:1 fish:brine ratio; then dry salted and matured for up to 3 months at 5°C by stacking fish and salt (1:1) in alternating layers | ≥![]() ![]() | 15![]() | Smaldone et al. (2017) | |
Marinating | Anisakis type I | Larvae (N![]() ![]() |
(a): 5% citric acid, 5% acetic acid, 13% NaCl (30°C); (b) 5% citric acid, 5% acetic acid, 10% NaCl (30°C); (c) 2.5% citric acid, 2.5% acetic acid, 8% NaCl (30°C) |
The inactivation strongly depends on the larval size. Ranges for inactivation: (a) 12–17 h; (b) 21–19 h; (c) 22–32 h. | Complex relation between larval size and inactivation in the different marinating solutions. No obvious trend observed | Giarratana et al. (2012) |
A. pegreffii | Larvae (N![]() ![]() |
(a) 10%, 20% sugar, (b) fresh lemon juice, (c) lemon juice plus 5%, 10%, 15% acetic acid, (d) alcohol vinegar, (e) wine vinegar, (f) apple vinegar, (g) water:vinegar 1:1 mixtures with alcohol (50%); wine (50%), or apple vinegar (50%) at 4°C |
About 5 48 h in intact alcohol vinegar (treatment (d)). > |
Treatment (a) 10% or 20% sugar is not effective Treatments (g) using water:vinegar 1:1 mixtures with either alcohol (50%); wine (50%) or apple vinegar (50%) were not efficient | Šimat and Trumbić (2019) | |
A. pegreffii | Anchovies (Engraulis encrasicolus) butterfly fillets, 18 batches of 100 individual fish spiked with 4 Anisakis larvae in four places per fillet |
(a) Traditional ‘carpaccio’: brining in 20% NaCl, 20 min, followed by marinating in fresh lemon juice with 5% acetic acid and olive oil. 7 (b) White vinegar marinade: 1:1 wine vinegar:water solution with 7% NaCl. 22 | In anchovies, the carpaccio marinade process (NaCl 20%, 5% acetic acid and olive oil) and white vinegar (water: white vinegar 1:1 and 7% NaCl) marinade was not effective |
Šimat and Trumbić (2019) | ||
Other chemical products: Natural products | ||||||
Natural compounds | Contracaecum sp. | Larvae in water |
Larvae in solution of (a) geraniol (250 μg/mL; 125 μg/mL; 62.5 μg/mL; 31.2 μg/mL; 0 μg/mL); (b) citronellal (250 μg/mL;, 125 μg/mL, 62.5 μg/mL;, 31.2 μg/mL, 0 μg/mL) up to 48 h | Non lineal dose–response of neither geraniol nor citronellal. Geraniol (a), 90% mortality with 250 μg/mL after 48 h | Barros et al. (2009) c | |
A. simplex | Larvae in 0.9% NaCl in cell culture media (i.e. RPMI‐1640) plus 20% fetal bovine serum (FBS), pH 4.0, 95% O2/5% CO2, 37°C | Larvae in 0.9% NaCl, RPMI‐1640 media plus 20% FBS with (a) [10]‐gingerol, (b) [6]‐gingerol, (c) [10]‐shogaol, (d) [6]‐shogaol, extracted from roots of Zingiber officinale (200, 150, 100, 10, 0 μM), incubated for up to 72 h | (a) [10]‐gingerol, 200 μM, 72 h | At 48 h, at this concentration, larval spontaneous movements were lost | Lin et al. (2010) | |
Anisakis type I | Larvae axenised in 0.9% NaCl | Larvae in 0.9% NaCl with (a) nerolidol, (b) farnesol, (c) elemol (sesquiterpenes); 125; 62.5; 31.2; 0 μg/mL, incubated at 36°C in 5% CO2 up to 48 h |
At the maximum concentration 125 μg/mL, all (a, b, c) achieved 100% mortality in 4 At 62.5 μg/mL, 100% mortality at 4 h for (a) nerolidol and (c) elemol; at 31.2 μg/mL, 100% mortality at 4 h for (a) nerolidol and 80% for farnesol (b), while elemol was ineffective (c); order nerolidol > farnesol > elemol | Treatments against anisakiasis; cuticular alterations and rupture of the intestinal wall by nerolidol, while farnesol and elemol only damages in the cuticle; nerolidol and farnesol, higher in vitro activity than cuminic aldehyde, carvacrol, citral, citronellol, eucalyptol, geraniol, myrcene, perillaldehyde, α and β pinenes and ocimene | Navarro‐Moll et al. (2011) | |
Anisakis type I | Larvae axenised in 0.9% NaCl (bioassay) | In vivo test with female Wistar rats by addition of larvae in 0.9% NaCl with (a) nerolidol, (b) farnesol, (c) elemol (sesquiterpenes), 46.9 mg of each compound in 0.5 mL olive oil. Animals killed after 4![]() | In bioassay, order of activity nerolidol![]() ![]() | Treatments against anisakiasis. Mechanism proposed for nerolidol and farnesol: inhibition of biosynthesis of isoprene compounds and increase in the permeability of the membrane; in vivo these are less active than citral, citronellol and perillyl aldehyde, but more active than cuminic aldehyde, carvacrol, eucalyptol, geraniol, myrcene, α and β pinenes and ocimene | Navarro‐Moll et al. (2011) | |
Anisakis simplex (s. l.) | Larvae in 0.9% NaCl | Larvae in 0.9% NaCl with (a) terpinel‐4‐ol (0, 0.1, 1, 10 μL/mL); (b) levamisole as reference antiparasitic drug (100 μL/mL), 24 and 48 h exposure, incubated at 37°C in 5% CO2 | Treatments against anisakiasis; (a) Terpinen‐4‐ol did not show any larvicidal activity | Gómez‐Rincón et al. (2014) | ||
Anisakis | Larvae in 0.9 NaCl | Addition of R (+) limonene (LMN) in saline solution (0.9% NaCl) and 0%, 0.1%, 0.5%; 1% and 5% LMN up to 48 h |
24 h with 5% LMN; 48 h with 1% and 0.5% LMN | Damages in the cuticle and digestive tract | Giarratana, Muscolino, et al. (2015) | |
Anisakis | Larvae in marinating solutions | Addition of R (+) limonene (LMN) in marinating solution [1:1 vol/vol distilled water and vinegar (6% acetic acid), 3% NaCl, 1% citric acid] and 0%, 0.1%, 0.5%, 1%, and 5% LMN up to 48 h |
16 h‐5% LMN; 24 h in 1% and 0.5% LMN | Damages in the cuticle and digestive tract | Giarratana, Muscolino, et al. (2015) | |
Anisakis | Larvae in seeds oil | Addition of R (+) limonene (LMN) in seeds oil and 0%; 0.1%, 0.5%, 1%, and 5% LMN, up to 7![]() | Weak effect of LMN in seeds oil (55%, 7![]() | Giarratana, Muscolino, et al. (2015) | ||
Anisakis type I | Larvae in saline solutions and in marinating solutions |
Addition of allyl isothiocyanate (AITC): (a) strong marinade, [1:1 vol/vol distilled water and vinegar (6% acetic acid), 3% NaCl, 1% citric acid]; (b) mild marinade [3:1 vol/vol distilled water and vinegar (6% acetic acid), 1.5% NaCl, 0.5% citric acid]; (c) physiological saline [0.9% NaCl] and [AITC] | LT100 after 180–300 min in the marinating solutions at all [AITC]; after 300–390 min in physiological solutions | Damage in the digestive tract | Giarratana, Panebianco, et al. (2015) | |
Anisakis type I | Larvae in anchovies homogenates (Engraulis encrasicolus) |
Addition of AITC in homogenate with anchovies with: (a) strong marinade [1:1 vol/vol distilled water and vinegar (6% acetic acid), 3% NaCl, 1% citric acid] or (b) physiological saline [0.9% NaCl] and [AITC] | In both homogenates LT100 after 100 min with 0.1% AITC; with 0.05% AITC at about 425 min only in the strong solution | No effect at 0.01% AITC in either solution; no effect with physiological saline at 0.05% AITC; damage in the digestive tract | Giarratana, Panebianco, et al. (2015) | |
Anisakis type I | Anchovies fillets (spiked) (Engraulis encrasicolus). |
Experimental marinating processes with: (a) strong marinade [1:1 vol/vol distilled water and vinegar (6% acetic acid), 3% NaCl, 1% citric acid] and [AITC] (b) [AITC] | (b) Pre‐treated with 0.1% AITC in phosphate saline followed by rinsing up, and then marinated strong, 60 h |
No satisfactory inactivation in industrial marinating process, and strong mustard flavour and odour conferred to the fillets; absence of mustard flavour and odour in fillets pre‐treated with AITC with phosphate saline buffer prior to marinating process; Damage in the digestive tract | Giarratana, Panebianco, et al. (2015) | |
Anisakis (92.86% A. pegreffii; one hybrid between A. pegreffii and A. simplex) | Anchovies fillets (spiked) (Engraulis encrasicolus) | Addition of R(+) limonene [LMN]![]() ![]() | Marinating solution with LMN was not effective at any concentration |
Nalbone et al. (2022) | ||
Anisakis (92.86% A. pegreffii; one hybrid between A. pegreffii and A. simplex) | Anchovies fillets (spiked) (Engraulis encrasicolus) | Marinating [1:1 (vol/vol) tap water:wine vinegar (6% acetic acid), 3% NaCl, 1% citric acid] at 4°C for 24 h, marinating solution removed, packaging in seed oil (SO), addition of R(+) limonene [LMN]![]() ![]() ![]() | With SO complete inactivation occurred after 8, 10, 20![]() | No differences in odour and taste sensory scores in fillets treated with 1%, and 0.5% and control. Only 5% LMN influenced the sensory characteristics of the fillets. Presence of injuries proportional to [LNM] Different behaviour as compared to in vitro test (i.e. in larvae in solution performed previously (Giarratana, Muscolino, et al., 2015). |
Nalbone et al., 2022 | |
Anisakis (92.86% A. pegreffii; one hybrid between A. pegreffii and A. simplex) | Anchovies fillets (spiked) (Engraulis encrasicolus). | Addition of R(+) limonene LMN (1%, 0%), marinating [1:1 (vol/vol) tap water:wine vinegar (6% acetic acid), 3% NaCl, 1% citric acid] at 4°C for 24 h, marinating solution removed, packaging in seeds oil (SO), addition of [LMN] =1%, 0%, (i.e. double treatment), 4°C, up to 8![]() | Inactivation on double treatment (1% LMN) after 7![]() | Presence of injuries. Addition of 1% LMN did not substantially change the sensory properties. The stability of LMN during processing and storage and the toxicity arising from its degradation products is needed before considering an alternative to freezing | Nalbone et al. (2022) | |
Essential oils | A. pegreffii | Larvae in oil (bioassay) | Origanum elongatum essential oil, in vivo test with female Wistar rats (46.9 mg/mL in 0.5 mL olive oil), killed after 4 h, control test without essential oil |
Significant larvicidal activity (i.e. 1 out of 15 rats showed gastric lesions) Alterations in oesophagus and cuticle of the recovered larvae after experimental rats were killed | Abattouy et al. (2010) | |
A. simplex (s. l.) | Larvae in 0.9% NaCl | Saline solution with tea tree (Melaleuca alternifolia) essential oil (TTO) (10, 7, 5, 4, 3, 2, 1, 0.5, 0 μL/mL, 37°C in 5% CO2; levamisole as reference antiparasitic drug (100 μL/mL), 24 and 48 h exposure |
Dose‐dependent lethal effect. 10 μL/mL, 24 h; 7 μL/mL, 48 h | Treatments against anisakiasis; eight compounds detected, the major one, terpinen‐4‐ol (46.9%); 96% inhibition acetylcholinesterase activity at the highest concentration; effectiveness of TTO for oral administration has been shown at lower concentrations than those that have proven to be toxic | Gómez‐Rincón et al. (2014) | |
Anisakis type I | Larvae in marinated solution | Larvae in marinating solution [water:vinegar 1:1; 3% NaCl; 1% citric acid] with Thymus vulgaris essential oil (TEO) (10%, 5%, 1%, 0.5%, 0.1%, 0%) up to 120![]() | Main components of TEO: thymol, linalool, carvacrol, alfa pinene, beta pinene and the remaining, terpenic compounds; no effect with TEO in marinated solution | Giarratana et al. (2014) | ||
Anisakis type I | Larvae in oil | Larvae with Thymus vulgaris essential oil (TEO) (10%, 5%, 1%, 0.5%, 0.1%, 0%) in sunflower seeds oil |
TEO in sunflower seeds oil at LT100 at 10% and 5% after 7 and 14 h, respectively; at 1% after 96 h, at 0.5% and 0.1% at 120 h | Damages in the cuticle and digestive tract | Giarratana et al. (2014) | |
Anisakis type I | Larvae in 0.9% NaCl | Larvae in a saline solution (0.9% NaCl) with Tagetes minuta essential oil [TEO]![]() ![]() ![]() | Complete inactivation after 2 h in saline solution with 5% and 1% of TEO. At 0.5% and 0.1% TEO, complete mortality was reached at 4 and 20![]() | Interruption of the digestive tract and injuries in the cuticle; 10 compounds detected in TEO, the main is β ocimene followed by limonene and (Z)‐tagetone. More effective than Thymus vulgaris and Nepeta cataria essential oils | Giarratana, Muscolino, Ziino, Giuffrida, et al. (2017) | |
Anisakis type I | Larvae in marinate solutions | Larvae in an industrial marinating solution [water:vinegar 1:1; 3% NaCl; 1% citric acid] with Tagetes minuta essential oil [TEO]![]() ![]() | Complete inactivation after 4 h at 5% TEO, and 6![]() | Interruption of the digestive tract and injuries in the cuticle; 10 compounds detected in TEO, the main is β ocimene followed by limonene and (Z)‐tagetone. More effective than Thymus vulgaris and Nepeta cataria essential oils | Giarratana, Muscolino, Ziino, Giuffrida, et al. (2017) | |
Anisakis type I | Larvae in oil | Larvae in sunflower seeds oil with Tagetes minuta essential oil (TEO) in sunflower seeds oil with [TEO]![]() ![]() ![]() |
16 h, 5% TEO; 24 h, 1%TEO; 36 h, 0.5% TEO; 48 h, 0.1% TEO | Interruption of the digestive tract and injuries in the cuticle; 10 compounds detected in TEO, the main is β ocimene followed by limonene and (Z)‐tagetone. More effective than Thymus vulgaris and Nepeta cataria essential oils | Giarratana, Muscolino, Ziino, Giuffrida, et al. (2017) | |
Anisakis type I | Larvae in 0.9% NaCl | Larvae in saline solution with Nepeta cataria essential oil (catmint) [CEO]![]() ![]() |
0.1%, 30 h; 1%, 24 h; 5%, 12 h; 10%, 6 h | Fifteen compounds were detected in the essential oil. Larvicidal activity proportional to EO concentration; damage of digestive tract | Giarratana, Muscolino, Ziino, Lo Presti, et al. (2017) | |
Anisakis type I | Larvae in marinate solution | Larvae in marinating solution (water/vinegar 1:1, 3% NaCl, 1% citric acid) with Nepeta cataria essential oil (catmint) [CEO]![]() ![]() |
0.1% and 0.5%, 48 h; 1%, 36 h; 5%, 18 h; 10%, 12 h | Fifteen compounds were detected in the essential oil. Larvicidal activity proportional to EO concentration; damage of digestive tract | Giarratana, Muscolino, Ziino, Lo Presti, et al. (2017) | |
Anisakis type I | Larvae in sunflower seeds oil | Larvae in sunflower seeds oil with Nepeta cataria essential oil (catmint) [CEO]![]() ![]() |
10%, 48 h 5%, 120 h | Fifteen compounds were detected in the essential oil. Larvicidal activity proportional to EO concentration; damage of digestive tract | Giarratana, Muscolino, Ziino, Lo Presti, et al. (2017) | |
A. simplex | Naturally parasitised blue whiting (Micromesistius poutassou). Fish were gutted, washed and placed in glass containers |
Fish placed in glass containers covered with each essential oil solutions. The containers were closed and kept for 24 h at 4°C. Essential oils: (a) Spanish lavender essential oil (Lavandula stoechas), (b) lavender (Lavandula spica) EO, (c) marjoram (Origanum majorana) EO, (d) oregano (Origanum vulgare) EO, (e) rosemary (Rosmarinus officinalis) EO, (f) cumin (Cuminum cyminum) EO, (g) thyme (Thymus vulgaris) EO. They were diluted in 5% v/v in olive oil. (h) Controls in parallel | Drop in survival rate but not complete mortality with any of the EO; O. vulgare the highest mortality rate (53.9%) in 24 h. 60%–80% of the larvae escaped the muscle | Gómez‐Mateos Pérez et al. (2017) | ||
A. simplex | Larvae which survived the in vitro trials after exposure to different essential oils; in vivo test (bioassay) |
In vivo test by infection to female Wistar rats with surviving larvae from in vitro trials after exposure to essential oils (i.e. larvae that were in the muscle or escaped to the container). Tested essential oils: (a) Spanish lavender essential oil (Lavandula stoechas), (b) lavender (Lavandula spica) EO, (c) marjoram (Origanum majorana) EO, (d) oregano (Origanum vulgare) EO, (e) rosemary (Rosmarinus officinalis) EO, (f) cumin (Cuminum cyminum) EO, (g) thyme (Thymus vulgaris) EO. (h) Controls in parallel. Animals were killed after 4 h infection. Larvae were mixed with 0.5 mL water | The parasites did not penetrate the digestive tract wall in the following cases: Spanish lavender (muscle, container); cumin (muscle, container); lavender (muscle); marjoram (container); oregano (muscle, container) | The parasites only penetrated the digestive tract wall in five cases (11.4%) | Gómez‐Mateos Pérez et al. (2017) | |
A. simplex | Larvae in 0.9% NaCl | Larvae in 0.9% NaCl with Origanum syriacum essential oil 0–1 mg/mL, at 24 and 48 h | 0.125 mg/mL, 24 h | Essential oil composed primarily by oxygen‐containing monoterpenes, with carvacrol as the most abundant (82.6%). Inhibition of acetylcholinesterase activity; loss of agar penetrative capacity after 1 h at the LC50 concentration | López et al. (2019) | |
Anisakis type I | Anisakis larvae in marinating solutions | Larvae in marinating solution [1:1 (vol/vol) water:vinegar, 3% NaCl, 1% citric acid] and different concentrations of essential oils: (a) roots of Ulvaria sheffleri, (b) fruits of Zanthoxylum chalybeum and (c) Vepris dainelli (5%, 1%, 0.5%, 0.1%, 0%) for up to 24 h | Dose‐dependent larvicidal activity, LT100 for (Z. chalybeum (b), U. sheffleri (a) and V. dainelli (c) 3 h, 5 h and 5h for 5% concentrations, respectively |
Essential oils obtained from U. scheffleri roots, Z. chalybeum and V. dainelli fruits contained a total of 58, 18 and 20 chemical constituents. Tricyclo [5.3.0.0(3, 9)] decane was identified to be the principal constituent in the essential oils of Z. chalybeum and V. dainelli. Weak cytotoxicity of essential oils at low concentrations | Anza et al., 2021 | |
Anisakis type I | Larvae in 0.9% NaCl | Larvae in 0.9% NaCl with Thymus zygis subsp. gracilis essential oil (0, 0.01%, 0.05%, 0.1%) up to 24 h at room temperature |
0.1%, 60 min; 0.05%, 120 min; 0.01%, 240 min | A total of 84 components identified, the major components being p‐cymene, thymol, carvacrol, and gamma terpinene. Lesions in the cuticle, interruptions of digestive tract | Bouymajane et al. (2022) | |
Contracaecum sp. | 10 larvae in oil, in duplicate, estimated total of 120 larvae | Larvae in oil with Ginger oil (Zingiber officinale) (400, 200, 100, 50, 25, 0 μ/mL) for 12, 24 and 32 h at 27–30°C | Calculated mortality: 295 μ/mL for 32 h (CI95) | Pavanelli et al. (2018) | ||
Essential oils and natural compounds | Anisakis type I | Larvae axenised in 0.9% NaCl | Larvae in 0.9% NaCl with (a) Matricaria chamomilla essential oil (EO), (b) α‐bisabolol or (c) Chamazulene at 125 μg/mL, 62.5 μg/mL, 31.2 μg/mL, 0 μg/mL, incubated at 36°C in 5% CO2 up to 48 h |
(a) With Matricaria chamomilla EO, 125 μg/mL, 100% mortality at 4 h; (b) α‐bisabolol complete mortality at all concentrations | 130 compounds detected in essential oil. Main compounds E‐β‐farnesene, α‐bisaboloxide B, α‐bisabolol, chamazulene, bisaboloxide A. Treatments against anisakiasis; changes in larval cuticle, muscle cells and digestive tract with EO or bisabolol; (c) chamazulene was not effective | Romero et al. (2012) |
Anisakis type I | Larvae axenised in 0.9% NaCl (bioassay) | In vivo test with female Wistar rats by addition of larvae with (a) Matricaria chamomilla EO or (b) α‐bisabolol (46.9 mg) in 0.5% olive oil, control group | Treatments against anisakiasis. 23.9% and 4.4% larvae were dead in the case of rats treated with EO and α‐bisabolol but only 2.2% of animals treated with EO and 5.5% with α‐bisabolol showed gastric lesions vs. 93.3% controls, thus EO induced reduction in the L3 infective capacity. Very low toxicity of M. chamomilla EO | Romero et al. (2012) | ||
A. simplex (s. s.) (54%); A. pegreffii (29.2%); hybrid band pattern (14.1%) | Axenised larvae in 0.9% NaCl | Larvae in 0.9% with (a) pepermint (Mentha piperita) essential oil (250 μg/mL and 187.5 μg/mL up to 48 h); (b) menthyl acetate, (c) menthone and (d) menthol, at 250 μg/mL, 187.5 μg/mL, 125.0 μg/mL, 62.5 μg/mL, 31.2 μg/mL, 0 μg/mL, incubated at 36°C in 5% CO2 up to 48 h. (e) abendazole at 0.5, 1 mg/mL |
Pepermint essential oil, 250 μg/mL in 4 h Menthol, 250 μg/mL and 187 μg/mL in 4 h | Main components of essential oil, menthyl acetate, menthone and menthol. No effect of menthone and menthyl acetate at 48 h. No activity of abendazole. Cuticle and digestive tract alterations of larvae | Romero et al. (2014) | |
A. simplex (s. s.) (54%); A. pegreffii (29.2%); hybrid band pattern (14.1%) | Larvae in olive oil (bioassay) | In vivo test with female Wistar rats by addition of larvae with (a) Pepermint (Mentha piperita) essential oil, (b) menthyl acetate, (c) menthone (49.5 mg/0.5 mL olive oil). Animals killed after 4 h infection. 5,4 mg/ 150 g body weight abendazole. Parallel controls |
These compounds (a, b, c) did not induce irritative effects in the tissues of the rats. No lesions in the gastrointestinal tract, no larvae attached Some larvae were recovered alive after necropsy of the rats. The viability of these larvae was reduced in 4 h after their extraction from the rats | Romero et al. (2014) | ||
A. simplex | Larvae in 0.9% NaCl |
Larvae in 0.9% with: (a) Myristica fragans (nutmeg) extract (0.1–0.7 mg/mL); (b) myristicin (0.1, 1, 2 mg/mL), incubated at 37°C in 5% CO2 up to 48 h. Levamisole (0.1 mg/mL, 1 mg/mL, 2 mg/mL) as reference antiparasitic drug | Levamisole 0.1 mg/mL, 24 h |
Four main compounds: myristicin, myristic acid, terpinen‐4‐ol, methoxyeugenol. The highest mortality rate was 92% with Myristica fragans, 0.7 mg/mL, 24 h Low lethal effect of myristicin (b). No inhibition of acetylcholine esterase enzyme activity (mechanism of action for other anthelmintic drugs) | López et al. (2015) | |
A. simplex | Larvae in 0.9% NaCl | Larvae in 0.9% with (a) Origanum compactum EO, (b) carvacrol, (c) thymol at 0–1 mg/mL, at 37°C up to 48 h. |
Origanum compactum EO, 1 mg/mL 24 h Carvacrol, thymol 1 | O. compactum EO is characterised by eight chemical compounds accounting for 93.6% of the total composition. Major components, phenol carvacrol, thymol, gamma‐terpinene, p‐cymene. All (EO and isolated compounds) have inhibitory effect of the acetylcholin esterase activity. The penetration rate in agar decreased 25% for treated larvae with sublethal doses of the oil | López et al. (2018) | |
Plant extracts | Anisakis type I | Larvae in olive oil enriched with 5% natural plants and spices | Larvae immersed in olive oil enriched with (a) cumin (Cuminum cyminum), (b) turmeric (Curcuma longa), (c) clove (Syzygium aromaticum), (d) thyme (Thymus vulgaris), (e) lemon zest (Citrus limon) (5%), and (f) control, at room temperature up to 15![]() | Turmeric (b) and cumin (a) oils are the most effective (6 and 7.7![]() ![]() ![]() ![]() |
The major compounds of the different spices: In ‘cumin olive oil’: β‐pinene, γ‐terpinene, p‐cymene, and cumin aldehyde; ‘turmeric olive oil’: 1,8‐cineole, α‐phellandrene and p‐cymene; ‘thyme olive oil’: p‐cymene, carvacrol and γ‐terpinene; ‘clove olive oil’: eugenol, β‐caryophyllene. ‘lemon olive oil’: limonene, β‐pinene and γ‐terpinene. The different types of lesions are related to the different active components | Trabelsi et al. (2018) |
Anisakis type I | Anchovies fillets (spiked) (Engraulis encrasicolus) | Anchovies in marinating solution [1:1 (vol/vol) distilled water:vinegar (6% acetic acid), 3% NaCl, 1% citric acid, 24 h at room temperature] and 4![]() ![]() | Cumin olive (b) the most effective, i.e. complete inactivation at 5![]() ![]() | No significant change in typical taste for either oil tested. The odour was pleasant for panellists, but more intense for cumin oil as compared to turmeric oil. More evident changes in colour for turmeric oil, no modifications in colour with cumin oil. Considering the long shelf life 120![]() ![]() | Trabelsi et al. (2018) | |
Anisakis type I | Larvae in olive oils enriched with 5% spices | Larvae immersed in Tunisian enriched olive oil with (a) cardamom (CaFO), (b) cinnamon (CiFO), (c) ginger (GiFO), (d) laurel (LaFO), (e) rosemary (RoFO) (5%) and (f) control, at room temperature (20°C) for up to 15![]() |
In vitro, LT100 at 3 7.9 12.5 14.9 15.6 | The major compounds: cinnamon olive oil: (E)‐cinnamaldehyde, 1,8‐cineole, α‐pinene; cardamom‐flavoured olive oil: 1,8‐cineole and α‐terpinyl acetate; rosemary‐flavoured olive oil: 1,8‐cineole, α‐pinene, camphor, camphene; ginger flavoured oil: camphene, limonene, citral, n‐dodecane, zingiberene; olive oil flavoured with laurel: 1,8‐cineole, linalool, β‐pinene, α‐pinene. Lesions observed in parasites, interruptions of the digestive tract and cuticle damage | Trabelsi et al. (2019) | |
Anisakis type I | Anchovies fillets (spiked) (Engraulis encrasicolus) in marinating solution | Anchovies in marinating solution [1:1 (vol/vol) distilled water:vinegar (6% acetic acid), 3% NaCl, 1% citric acid, 24 h at room temperature] and 4![]() ![]() | 4![]() ![]() | Odour in CiFO was just perceptible only after 14![]() | Trabelsi et al. (2019) | |
Anisakis type I | Larvae in olive oils flavoured with natural plants and spices | In vitro larvicidal activity of olive oil flavoured with (a) cumin (Cm‐FOO) or (b) with a mixture with lemon, parsley and fresh garlic (10%) (Mix‐FOO), 20°C up to 24 h | 24 h in both conditions | Complex chemical composition and antioxidant potential of olive oils. Lesions in the cuticle, interruptions of the digestive tract | Trabelsi et al. (2021) | |
Anisakis type I | Anchovies fillets (spiked) (Engraulis encrasicolus) | Anchovies in marinating solution for 24 h [1:1 (vol/vol) distilled water:vinegar (6% acetic acid), 3% NaCl, 1% citric acid] at 4°C, covered with either (a) Cm‐FOO or (b) Mix‐FOO (10%) and maintained at 20°C | 8![]() ![]() | Good sensory acceptability with no differences between FOOs and control groups | Trabelsi et al. (2021) |
Abbreviations: [], concentration; CI95, 95% confidence interval; FBS, Fetal Bovine Serum; LC50, lethal concentration 50 or concentration that causes 50% inactivation; LT100, lethal time for complete i.e. 100% inactivation; MPa, megapascal; MW, megawatt; NaCl, Sodium chloride; RPMI‐1640, cell culture medium, i.e. Roswell Park Memorial Institute medium; RT, room temperature; SEM, scanning electron microscopy; W, Watt.
Natural products: AITC, allylisothiocyanate; CaFO, Tunisian enriched olive oil with cardamom; CiFO, Tunisian enriched olive oil with cinnamon; Cm‐FOO, olive oil flavoured with cumin; EO, essential oil; FOO: flavoured olive oil. GiFO, Tunisian enriched olive oil with ginger; LaFO, Tunisian enriched olive oil with laurel; LMN, R(+) Limonene; Mix‐FOO, olive oil flavoured with a mixture with lemon, parsley and fresh garlic (10%); RoFO, Tunisian enriched olive oil with rosemary; SO, seed oil; TEO, Tagetes minuta essential oil; TTO, tea tree essential oil.
3.3.3. Physical treatments
3.3.3.1. Conventional heating
Heating of fish is usually the last step where fish parasites can be inactivated before fish consumption. The ways of cooking have been classified into moist heat methods (i.e. boiling, poaching, steaming), dry heat methods (baking, grilling, frying, roasting, broiling) or a mixture of both (e.g. braising and stewing) (Sampels, 2015) resulting in a wide array of time and temperature combinations. Since 2010, the effect of heating has been revisited using model systems with isolated larvae as well as spiked fish but mostly for Anisakis larvae.
Vidaček et al. (2010) heated Anisakis L3, immersed in 10 mL of distilled water (5 larvae in 10 mL, five replicated measurements/condition), in a water bath and observed spontaneous movements in all larvae after exposure for 1 min at 60°C. The time to reach 60°C was ~3 min. They reported that more than 10 min at 60°C was needed to ensure the death of all larvae in both batches tested. At 70°C, larvae were inactivated within 1 min.
In another work on Anisakis, 12 L3 larvae were placed onto pieces of hake fillets covered with the matching portion of the other fillet from the same individual and wrapped, thus making a ‘sandwich’ (Vidaček et al., 2011). Each sandwich was vacuum sealed and stored for 20 h to allow the larvae to migrate into the fish flesh, and then heated in a water bath at 93°C. In these conditions, complete inactivation of larvae was obtained after 5 min and 3 min holding time once hake sandwiches reached 60°C and 70°C, respectively, in the core of the piece. The time to reach these temperatures in the thermal centre was 6.5 and 11 min, respectively. Sánchez‐Alonso, Carballeda‐Sangiao, González‐Muñoz, et al. (2021) used different heating regimes in hake sandwiches (i.e. (i) water bath set at 92°C up to achieve target temperatures of 50, 60 and 70°C and then cooled in ice water, (ii) oven set at 200°C until 50°C was reached in the core and cooled in ice water, (iii) oven set at 200°C and removal of sandwiches at 7, 10, 15 or 20 min and cooled at ambient temperature and (iv) water bath set at 60°C or 70°C, and once 58 and 68°C were reached, samples were cooled in ice water immediately or after 2.5 and 5 min). They reported that reaching 60°C for 1 min at the core of hake sandwiches spiked with either 15 or 35 Anisakis L3 (A. simplex, s. s) was not sufficient to kill all the larvae. However, none of the larvae that had been exposed to heating at >60°C, for at least 1 min in the thermal centre, were able to penetrate into agar, which suggests a low infective potential of these surviving larvae. If other parameters such as the time to reach the target temperature were considered, they reported that, provided a minimum cooking time was achieved (e.g. 14 min in the studied conditions) lower temperatures at the thermal centre (i.e. 55°C) were sufficient to kill all of the parasites. This is an important finding as many traditional cooking methods do not achieve a core temperature of 60°C (e.g. Huss, 1994).
Cryptocotyle lingua metacercariae were exposed to heating in artificially infected cod sandwiches and in naturally parasitised cod. They were inactivated in less than 15 min at 70°C, 80°C and 90°C and less than 10 min at 100°C, in 30 min at 60°C, but 2 h was needed when incubated at 50°C (Borges et al., 2015).
The thermal susceptibility of parasites is affected by the experimental conditions and methods used (matrix, larvae either isolated in incubation solutions or included in fish fillets naturally or artificially inoculated; heating profile obtained from direct/indirect hot water bath immersion or heating in an oven, frying, e.g.) (Guan, Usieto, Sánchez‐Alonso, et al., 2023) as well as larval species and implicit factors (age, size, etc.), all of which can significantly affect the results and at least in part, explain the discrepancies found in the literature (Table 7). In addition, when the treatments are performed in large liquid volumes or in infected fish, thermal gradients and the variable distribution of the parasites within the fish matrix introduce a confounding effect, making it difficult to characterise the thermal resistance of the parasites.
Quantification of the thermal effects in model systems
Quantitative characterisation of the thermal resistance of parasites, carried out through heat treatments in well controlled (quasi‐)isothermal conditions, is the first step in establishing the time–temperature combinations required to kill Anisakis that could be applicable in current cooking practices.
Giarratana et al. (2012) immersed Anisakis type I in glass test tubes containing prewarmed physiological saline solution maintained in a water bath at 45°C, 47°C, 48°C and 50°C (total n=
400 larvae). Lethal times (defined as the time required to inactivate the totality of larvae (n) exposed to a given temperature) varied from about 5 min at 50°C, 40 min at 47°C, to 80–90 min at 45°C. Guan, Usieto, Sánchez‐Alonso, et al. (2023) individually immersed more than 10,000 viable A. simplex (s. s.) L3 of 0.145 M NaCl (in 96‐well microplates one larvae per 30 μL, 96 larvae/microplate) and heated them at temperatures ranging from 44°C to 61°C in a thermal cycler under quasi‐isothermal conditions. At each temperature, the survival ratios (i.e. the number of surviving larvae after an exposure time to a lethal temperature divided by the initial number of larvae) were calculated at different exposure times until the death of all the larvae was achieved. Those time/temperature (t/T) conditions that killed all larvae (i.e. no survivors) were additionally replicated to get n
=
288 larvae per condition so that at these lethal times and temperatures, at least 99.65% of the Anisakis population will be dead (≈LT99.65). There was a negative exponential relation between the treatment temperature (46–61°C range) and the minimum exposure time required to achieve no survivors in the A. simplex (s. s.) sample used. For example, slightly more than 2 h and only 1 s were needed to kill all the larvae treated at 46°C and 61°C, respectively (Table 7).
Modelling survival curves: Predictive modelling is an alternative approach to quantitatively characterise the thermal resistance of biological hazards as a function of time and/or temperature, enabling an estimation of the lethal time with high probabilities. Guan, Usieto, Sánchez‐Alonso, et al. (2023) found that survival ratios during heat treatments described above, at a given temperature showed a sigmoidal shape with exposure time, with an initial shoulder for the shorter treatments at each temperature and a final tail for the longer heating times. Different primary models were used to characterise the survival curves of Anisakis L3 at each fixed temperature, with the Weibull model best fitting the observed data (Table 7), enabling an estimation of the δT values (i.e. the exposure time required at a certain temperature for a first decimal reduction of the survival ratio). The obtained δT values were used to build a secondary model describing their temperature dependence. Among the models tested, the empirical quadratic polynomial relationship of the temperature with log10 δ provided the best fitting. Validation experiments performed at temperatures different to those used to create the model corroborated its predictive capacity (Guan, Usieto, Sánchez‐Alonso, et al., 2023).
For the quantification of the efficacy of thermal treatments (e.g. in other parasites, fish matrices, etc.), the number of treated larvae to obtain results with sufficient statistical power needs to be high. Considering the high abundance and intensity of Anisakis infection present in fishery products, lethal time estimations with high confidence would require the assessment of thousands of larvae for a given t/T combination, and this is also the case for building predictive models. Achieving these high numbers is difficult using the current visual inspection method, and high‐throughput screening techniques that help to analyse the viability of a large number of larvae in a short time would be required (Guan, Usieto, Sánchez‐Alonso, et al., 2023).
On the other hand, the study of the characteristics of the surviving larvae after a thermal treatment could help in the assessment of the associated risks, i.e. if still viable their infectivity should be assessed. This would allow for a more realistic assessment of the effect of a given treatment on the risk of human infection. Guan et al. (2022) monitored the progressive loss of larvae function after isothermal treatments at 50°C and different exposure times, under quasi‐isothermal conditions, since these temperature–time combinations may actually occur in undercooked fish and lead to a wide range of survival ratios (0–1). Larvae that survived heat treatments were collected and agar penetrative capacity, lifespan in artificial gastric fluid, respiratory capacity and locomotor activity were monitored. Interestingly, agar penetrative ability was significantly reduced in larvae that had survived a thermal treatment at 50°C for >60 s, conditions which still rendered survival ratios of 1, while heat expositions for 90 s with survival ratios ≈
0.98 also reduced the resistance to simulated gastric fluid and the respiration capacity, suggesting a low infectivity of these larvae.
Since the experimental conditions used in model systems substantially differed from those occurring during the cooking of fish, future efforts should focus on predicting larvae viability at non‐isothermal conditions such as those that occur during fish cooking (i.e. also considering the heating up and cooling down temperatures and times), and also on the effect of the matrix on thermal susceptibility.
3.3.3.2. Microwave treatment
The method of heating of solid foods by microwaves involves dielectric and ionic mechanisms, water in the food being the primary component responsible for dielectric heating since as water oscillates at the high frequencies of the microwave ovens, heat is produced. The oscillatory migration of ions in the food is the second mechanism that generates heat under the influence of the oscillating electric field (Datta & Davidson, 2000; Ozkoc et al., 2014). As for microorganisms, it is thought that temperature/time history at the coldest location will determine the safety of the process (Datta & Davidson, 2000). However, uneven distribution of the heat may occur in standard domestic microwave ovens so that turning food during cooking, covering food and adding liquid as it cooks all help to even out the heating (Datta & Davidson, 2000; Ozkoc et al., 2014).
Several studies show that temperatures ≤74°C combined with different times (i.e. 74°C, 15 s was the temperature/time combination recommended in EFSA BIOHAZ Panel (2010)) are effective in killing A. simplex (s. l.) L3 larvae. For example, Vidaček et al. (2009) microwaved water suspensions of ovaries and viscera tissues heavily infected with larvae at 900 W for 30 s, and no survivors were found. The maximum temperatures of the samples ranged between 67.8°C and 69.2°C. In hake sandwiches processed in a commercial 900 W microwave oven for 3 min, 66.9°C was reached in the thermal centre of the sandwiches immediately after treatment and no larvae survived (Tejada et al., 2006). This was also the case for larvae in a microwave digestion oven which allowed control of the time to reach a preset temperature as well as optimal distribution of the microwaves (Vidaček et al., 2011). Thus, in conditions where the target temperature was reached in 1 min, 60°C (800 W) or 70°C (1,000 W) for a holding time of 1 min was lethal to Anisakis larvae immersed in distilled water. In hake sandwiches, 5 min and 3 min were needed, respectively, for killing all tested larvae under these microwave conditions. In another study, fish sandwiches spiked with Anisakis spp. were placed in a microwave oven at 600 W. Samples were neither covered nor rotated. After 1 min, a temperature sensor was inserted into the sample without removing it from the oven. Once the temperature was registered, the sample was removed and maintained at room temperature for 15 min. Surviving larvae were not detected when the registered temperature was 75.6°C (Lanfranchi & Sardella, 2010) (Table 7).
The U.S. Food and Drug Administration (FDA, 2023) recommends, for raw animal foods, an internal temperature of 74°C in all parts of microwaved animal‐derived foods plus an additional 2 min of standing time for the covered food to achieve temperature equilibrium, although this is not specifically for zoonotic parasites. In addition, food should be rotated or stirred throughout or midway during cooking to ensure a more even distribution of heat and covered to retain surface moisture.
Results of survival after microwave treatment vary among studies owing to the lack of standardisation of wattage in standard domestic microwave ovens (usually 800–1200 W), uneven distribution of the microwaves, differences in size, shape, composition and dipolar components in the food. The effect of moisture and salt content in the products is significantly higher using microwaves as compared to conventional processing due to their effect on dielectric properties (Vidaček et al., 2011). More data should be gathered in order to define more specific lethal conditions for parasites in microwaved fishery products.
3.3.3.3. High‐pressure processing
High‐pressure processing (HPP) is a non‐thermal treatment in which foods are subjected to isostatic pressures. Traditionally, this is an in‐batch process that can be applied to both solid and liquid prepacked foods. Pressure is transmitted rapidly and uniformly in an isostatic manner so that all parts of the food are subjected to the same pressure simultaneously.
An EFSA scientific opinion on the efficacy and safety of high‐pressure processing of food has been recently published (EFSA BIOHAZ Panel, 2022). Within the industrial context, pressures of between 400 and 600 MPa are most often applied for microbial inactivation in foods, with common holding times ranging from 1.5 to 6 min. The water used as pressure‐transmitting fluid is often prechilled at 4–8°C.
HPP ranging from 100 to 350 MPa (1–5 min) was applied to A. simplex larvae and parasitised hake (Merluccius merluccius) muscle. The larvae were killed at pressures ≥200 MPa and times ≥
1 min, producing alterations in the larva body and ruptures in the cuticle when observed by scanning electron microscopy (Vidacek et al., 2009). In this study, the increased shear resistance and changes in colour of the fish muscle were also detected. However, visual changes in appearance of the fish muscle were observed only at 300 MPa.
In studies undertaken since 2010, Lee et al. (2016) exposed the white spotted conger flesh containing 20 live larvae to different pressures (150 and 200 MPa for 1 and 5 min; 250 and 300 MPa for 1 min). The optimum HPP inactivation conditions for A. simplex L3 in the flesh were found to be 200 MPa at 5 min and 300 MPa for 1 min, since they rendered complete mortality of the tested larvae but the former was preferred since it resulted in no significant changes in colour and sensory analysis. In another study, infected hake sandwiches were treated at 200 MPa for 15, 30, 60–90 s. In these conditions, all A. simplex larvae survived, but none of them were able to penetrate into agar, thus suggesting an impaired infectivity after HPP application (Parasite Consortium, 2016). As indicated in the previous EFSA BIOHAZ Panel (2010) opinion, the changes in muscle colour and appearance may limit the application of HPP for the treatment of raw fish, but this treatment may be suitable for particular fish species or for processed fish (Table 7).
3.3.3.4. Pulsed electric fields
Pulsed electric field (PEF) treatments consist of subjecting a product placed between two electrodes, usually immersed in an aqueous solution, to high‐intensity electric fields (between 0.5 and 30 kV/cm) by applying intermittent pulses of short duration (microseconds to milliseconds) without increasing the product's temperature (Martínez et al., 2023).
Onitsuka et al. (2022) applied PEF to fillets of horse mackerel (Trachurus japonicus) spiked with Anisakis, presumably A. pegreffii. The fish fillets were immersed in a saline solution, and pulsed power was applied. They observed optimal inactivation at NaCl solutions with electrical conductivity of 5 mS/cm, charging voltage 15 kV, capacitance 80 μF, frequency 1 Hz, the distance between electrodes 11 cm and 500 pulses. Sensory evaluation after the treatment showed that, although slightly lower than the control, the fish retained their quality as sashimi.
Abad et al. (2023) evaluated the efficacy of PEF on the inactivation of Anisakis spp. larvae (n=
10 larvae per condition in triplicate, total 480 larvae in the calibration experiment) in an aqueous solution. Response surface methodology was used and a central composite design was constructed to evaluate parameters such as electric field strength (1–3 kV/cm), specific energy (3–50 kJ/kg) and pulse width (3–100 μs) on Anisakis survival and data obtained were described by a second‐order polynomial equation. The viability of Anisakis was highly dependent on field strength. The loss of survival as a function of specific energy was higher at the highest field strength (3 kV/cm). On the other hand, at the lowest field strength (1 kV/cm) pulse width exerted a significant influence, whereas no effect was found at the highest field strength (3 kV/cm). These results were validated with an independent experiment in aqueous solution with very good relationship between the predicted and experimental data. Maximum inactivation (i.e. 90%–100%) was obtained at 3 kV/cm and 50 kJ/kg.
In hake spiked with Anisakis (n=
10 larvae per condition, total of 600 larvae), Abad et al. (2023) used pulses of 30 μs and 50 kJ/kg specific energies, with varying field strengths (1 to 3 kV/cm) and compared the percentage of survivors with the expected mortality according to the polynomial model developed in isolated larvae. A good agreement was found with the model, confirming that a treatment condition using 30 μs, 50 kJ/kg and 3 kV/cm is capable of inactivating a significant percentage of Anisakis present in pieces of hake (i.e. 80%–100%). The authors observed that the application of this optimal PEF treatment to hake fillets affected moisture, water‐holding capacity and cooking loss to a lesser extent than if these fillets were frozen at −18°C for 48 h and then thawed.
In another study, Onitsuka et al. (2024) studied: (a) the effect of water conductivity, (b) the capacitance, (c) charging voltage of the energy storage capacitor, (d) the input energy density into the treatment electrode, (e) the distance between parallel plate electrodes, (f) the position of the larvae in the fish fillet, or (g) in whole fish, and (h) the fish species in spiked and in naturally parasitised fish. They observed that the higher the input energy density per pulse (J/cc/pulse), the higher the inactivation effect of Anisakis larvae. However, the inactivation efficiency was affected by the position of the larva in the fish flesh owing to the effect of the shape and composition on current distribution. The authors did not find differences between naturally parasitised and spiked fish. Unlike previous studies (Abad et al., 2023; Onitsuka et al., 2022), they achieved complete immobility even at about total input energy density of 10 J/cc, suggesting that Anisakis can be inactivated at relatively low energy. According to this study, the applied energy was significantly lower than that used in their previous work (260 J/cc) (Onitsuka et al., 2022) and of Abad et al. (2023) (3 kV/cm; 50 J/cc) (Table 7). The authors suggested that more studies need to be performed to improve efficiency and to standardise conditions (Onitsuka et al., 2024).
Martínez et al. (2023) reviewed the potential application of PEF technology in the control of food‐borne parasites. The authors highlighted the need to continue research related to the execution and scaling of PEF treatments to facilitate the development of industrially competitive processes. They considered that the presence of bubbles, the need to design new treatment chambers and the absence of sufficiently powerful equipment are problems to be solved for the treatment of solid products such as fish or fish fillets. The electrical parameters must also be carefully optimised to avoid alterations in quality and a case‐by‐case analysis of the PEF efficiency in each of the matrices is necessary.
3.3.3.5. Other physical methods
Drying is a processing/preservation method in which the water content and water activity are decreased due to osmotic pressure, resulting in a decreased bacterial and enzymatic activity (Sampels, 2015). One of the most popular dried fish products is ‘stockfish’, an unsalted, naturally dried fish product mainly made from cod. In the traditional process, beheaded and gutted cod are hung outdoors at 0–2°C for about 3 months, then left for maturation and storage in dry, ventilated warehouses for 2–12 months, and the final step consists in rehydration of the fish (2–15days at 2–4°C), so production usually takes a minimum of 5 months before the stockfish is ready for consumption. It was shown that this product was free of viable anisakid nematodes in a total production process of 7.5 months (Bao et al., 2020). However, there is no information on the inactivation within shorter periods of time.
Alternative techniques to conventional freezing include also the use of ultrasounds (US), which produces cavitation in liquids (formation, growth and implosion of tiny gas bubbles in the liquid when the ultrasonic waves pass through), variations in pressure in gas and movement of liquids in solid media. Fluid mixing and shear forces on a micro scale are generated by this high‐frequency vibration (Chavan et al., 2022). The effect of US (37 kHz and 1200 W, 0–30 min) on isolated Anisakis larvae in glass tubes has been studied (Oh et al., 2014). Viability was reduced to 5.6% when ultrasound alone was used to treat live larvae in test tubes for 30 min. When 1500 ppm chlorine was added and then, 37 kHz, 1200 W for 30 min was applied, larval inactivation was complete in isolated larvae, but this treatment was unsuccessful in naturally infected conger (Conger myriaster) viscera. Other authors have also reported the inability to inactivate Anisakis larvae at an ultrasound intensity of 40 kHz and 150 W, up to 30 min (Parasite Consortium, 2016) (Table 7).
There is no new data on the effect of irradiation, low voltage current and hot smoking as treatments to kill parasites of public health importance since the last EFSA BIOHAZ opinion was published in 2010.
3.3.4. Chemical treatments
3.3.4.1. Salting
Salting is a processing and preservation method by which the water content and water activity of the fish are lowered thus leading to lower concentrations of bacteria and enzymatic activity. The high salt concentration creates an environment where most bacteria and fungi cannot grow or survive (Sampels, 2015), but as stated in the previous EFSA opinion (EFSA BIOHAZ Panel, 2010). Anisakidae are to some extent resistant to this salt environment and traditional products need to be tested accordingly.
The resistance of anisakids (80% Anisakis and 20% Phocanema larvae) over time was assessed in ‘baccalà’ (salted cod), one of the salted products consumed in the Mediterranean countries. Smaldone et al. (2017) prepared ‘baccalà’ by a double salting process [i.e. first in a 13% NaCl solution at 5°C for 24 h in a 1:1 fish to brine ratio, and then dry salted and subjected to maturation for up to 3 months at 5°C by stacking cod and salt in alternating layers (1:1 weight)]. After 15days of ripening, the baccalà contained 18.6% NaCl, had a water activity of 0.751 and 24.15% water phase salt which resulted in all Anisakidae larvae (that were naturally present) being inactivated. Fifteen days is considered the minimum time required in order to obtain commercial salted cod.
Fillets of anchovies (Engraulis anchoita) were immersed in saturated NaCl solutions for 4days. They were then headed, gutted and trimmed, spiked inside the fillet with larvae of different genera and exposed to dry salting according to the process used locally, consisting in a layer of fish/salt in a 1:1 proportion for 1–24 h. Larvae of the genera Contracaecum, Hysterochylacium and Phocanema were inactivated after 1 h and Anisakis larvae rendered non‐viable at 16 h (Lanfranchi & Sardella, 2010). Interestingly, the storage time of this anchovy product in Argentina is 6–8 months.
The effectiveness of the traditional dry salting process for anchovies in southern Italy was assessed for A. pegreffii mortality (Anastasio et al., 2016). Ungutted fish were mixed with NaCl in a fish:salt ratio of 3:2, then placed in plastic drums, pressed and stored at 25°C for 120days. The results showed that, starting from the 15
days of the ripening process, all larvae were considered dead. At that stage, 21% NaCl concentration was reached in the salted anchovies. The time of treatment is eight times longer than the effective time requested to inactivate the anisakidae larvae. In another study, eviscerated anchovies were subjected to dry salting, pressed for 10
days at 16°C and matured for up to 20
days. Dry salting was effective in killing A. pegreffii after 10
days at 16°C (Šimat & Trumbić, 2019).
To determine which salt brines can be considered safe in terms of Anisakis inactivation, live larvae isolated from the viscera of conger eels were immersed into brines containing 5, 10, 15 or 20% NaCl at 4°C (20 larvae/solution) and stored for up to 7days (Oh et al., 2014). Only at 15% NaCl or higher, were all larvae inactivated at 7
days and lower salt concentrations rendered high survival ratios, specially at 5% NaCl. In another study, A. pegreffii larvae immersed in different brines ranging from 5% to 35% NaCl at 4°C (Šimat & Trumbić, 2019) were inactivated at 35% NaCl in 3
days, whereas 10
days were required using 5% NaCl (Šimat & Trumbić, 2019) (Table 7).
According to the review of Raether and Hänel (2003), Diphyllobothrium is inactivated by placing the fish in brine (12% NaCl).
3.3.4.2. Marinating
Marinating is a traditional process in which fish is immersed in vinegar and other ingredients, with the aim of inhibiting or retarding bacterial growth by lowering the pH and also conferring desired organoleptic characteristics on the product (EFSA BIOHAZ Panel, 2010; Sampels, 2015). Since 2010, different marinating solutions have been investigated by exposing isolated Anisakis spp. larvae in the solution, which included salt and sugar, lemon juice, acetic acid, alcohol, wine and/or apple vinegar. The survival of larvae immersed in these solutions was about 5days in lemon juice and lemon juice with acetic acid. In alcohol vinegar, larvae survived for only 48 h (Šimat & Trumbić, 2019). Other authors have included citric acid, acetic acid and NaCl at different concentrations and observed inactivation of isolated larvae at times ranging from 12–17 h to 22–32 h, and reported a complex relation between larval size and different marinating solutions in Anisakis type I larvae (Giarratana et al., 2012). However, these studies may not reflect what happens when the fish is marinated. Carpaccio and wine vinegar marinades, for example, failed to kill Anisakis spiked in anchovies during the marinating process, which were only inactivated after 60
days of storage (Šimat & Trumbić, 2019).
3.3.4.3. Other chemical procedures – The use of natural products
A wide range of compounds from plants have been investigated for potential application as anthelmintics against human parasitosis. These include plant extracts, essential oils or compounds isolated from these with different chemical compositions (Valero et al., 2015). These compounds could also be used as food additives and the in vitro and in vivo activity of these natural products against Anisakis has also been analysed with the aim of killing parasites present in different food products. Model systems with larvae immersed in different media such as water or saline solutions, marinating solutions or in oils have been used. Parasite inactivation has also been studied in fish homogenates and in fish muscle. Although in most cases the envisaged food applications refer to the industrial‐like production of the anchovies marinating process, other applications have been proposed, and thus, the anthelmintic effects of natural products are presented as a separate section from marinating. Most of the information published to date has focused on Anisakis type I with a few reports covering other anisakids (Table 7).
Natural compounds
Valero et al. (2015) reviewed the larvicidal activity of different natural products and observed that ar‐turmerone, a component isolated from the root of turmeric (Curcuma longa) has the highest larvicidal activity against Anisakis at 24 h and 25 μg/mL. Many compounds exert high larvicidal activity at ≤4 h in higher concentrations (i.e. 125 μg/mL). These included carvacrol, citronellol, citral, α‐pinene, ocimene, nerolidol, farnesol, elemol, α‐bisabolol or thymol (Mladineo et al., 2018; Navarro‐Moll et al., 2011; Romero et al., 2012; Valero et al., 2015).
However, the larvicidal activity of the natural compounds can be different depending on the matrix, as is the case for allylisothiocyanate (AITC), a natural antimicrobial found in seeds, leaves, stems and roots of cruciferous plants. The use of AITC killed all larvae present in anchovies within 60 h when they were pretreated with 0.1% of this compound in saline solution, rinsed and then marinated whereas when added directly to the marinating solution 0.1% AITC was ineffective (Giarratana, Panebianco, et al., 2015). With R(+) limonene (LMN), the major aromatic compound in essential oils obtained from oranges, grapefruits and lemons, larvae were inactivated after 24 h in a 5% LMN solutions and up to 48 h treatment was necessary at lower concentrations (i.e. 0.5%). When this compound was added to a marinating solution, 16 h was needed at 5% for complete inactivation but at 24 h 0.5% LMN was equally effective (Giarratana, Muscolino, et al., 2015). LMN had low larvicidal effect in oil, but complete inactivation was achieved in 8days when marinated anchovies were packaged in 5% LMN sunflower seed oil at 4°C. Moreover, its effect was stronger when a double treatment with 1% LMN was performed, i.e. when it was applied during both the marinating and the packaging in oil processes (Nalbone et al., 2022).
According to Barros et al. (2009), geraniol at 250 μg/mL inactivated 90% of Contracaecum sp. in 48 h (Table 7), whereas 125 μg/mL for 24 h was required for 100% inactivation of Anisakis sp. (Valero et al., 2015).
Essential oils
Many essential oils (EO) exert in vitro activity against Anisakis in saline solutions. These include tea tree oil (Melaleuca alternifolia), which has been reported to have the highest inactivation properties, with a lethal time of 24 h at 10 μg/mL (Gómez‐Rincón et al., 2014; Valero et al., 2015). Other essential oils such as lemongrass (Cymbopogon citratus), chamomile (Matricaria chamomilla) at 125 μg/mL and peppermint (Mentha piperita) oils at 250 μg/mL killed the larvae within 4 h (Romero et al., 2012, 2014; Valero et al., 2015). Tagetes minuta and Thymus zygis subsp. gracilis inactivated Anisakis in 2 and 4 h at 1% and 0.01% solutions, respectively (Bouymajane et al., 2022; Giarratana, Muscolino, Ziino, Giuffrida, et al., 2017). Nutmeg (Myristica fragans) essential oil had larvicidal activity (i.e. 92% mortality rate) between 500 and 700 μg/mL at 24 h and showed no signs of cytotoxicity (López et al., 2015). Essential oils from Origanum such as O. vulgaris (Valero et al., 2015), O. compactum (López et al., 2018) and O. syriacum (López et al., 2019) also had strong larvicidal activity. In the latter case, although complete mortality occurred at 24 h, agar penetration capacity was 0 after 1 h exposure to the oil at LC50. This suggests Origanum's potential to inhibit the invasion of the host tissues and reduce Anisakis pathogenic capacity (López et al., 2019). In marinated solutions, Ulvaria sheffleri, Zanthoxylum chalybeum and Vepris dainelli essential oils killed all Anisakis larvae present after 3, 5 and 5 h, respectively, at 5% concentration (Anza et al., 2021). These essential oils showed weak cytotoxicity at low concentrations. Examples of the effect of the matrix on the larvicidal activity include Tagetes minuta EO, where complete inactivation was reached after 4 h and 5% EO in marinated solution, whereas in sunflower seed oil, 16 h was needed at this concentration, or 24 h at 1% (Giarratana, Muscolino, Ziino, Giuffrida, et al., 2017). Thyme, Thymus vulgaris essential oil had only complete larvicidal effect in oil, no effect in marinated solutions (Giarratana et al., 2014) and very low effect (11.4% mortality in 5% v/v oil during 24 h) in naturally parasitised blue whiting (Micromesistius poutassou) (Gómez‐Mateos Pérez et al., 2017). Nepeta cataria (catmint) essential oil at 0.1% was sufficient to kill these larvae in 48 h but 10% was needed when dissolved in oil to achieve the same effect in this time (Giarratana, Muscolino, Ziino, Lo Presti, et al., 2017).
The highest biocidal activity in naturally parasitised blue whiting among different essential oils from Mediterranean plants, i.e. Spanish lavender (Lavandula stoechas), lavender (Lavandula spica), marjoram (Origanum majorana), oregano (Origanum vulgare), rosemary (Rosmarinus officinalis), cumin (Cuminum cyminum) and thyme (Thymus vulgaris) was achieved with oregano essential oil, with 53% mortality in 24 h. However, the other essential oils had little or no effect. Interestingly, between 60% and 88% of parasites treated with these essential oils migrated out of the muscle, and in vivo tests showed that rats inoculated with larvae that survived treatments with oregano, cumin and Spanish lavender did not show lesions in the digestive tract (Gómez‐Mateos Pérez et al., 2017).
In vitro exposure to ginger essential oil was lethal for all tested Contracaecum sp. larvae at concentrations of 295 μg/mL (CI95) after 32 h (Pavanelli et al., 2018).
Plant extracts
Saline solutions of plant extracts such as Perilla frutescens, turmeric (Curcuma longa), ginger (Zingiber officinale), wasabi (Wasabia japonica) and garlic (Allium sativum) killed Anisakis larvae in 3–11 h at concentrations of 1.25%–5% (Valero et al., 2015).
The addition of aromatic plants to virgin olive oil has been traditionally used in several dishes for improvement of their sensory characteristics, shelf‐life extension and enhancement of the nutritional characteristics (Akçar & Gümüşkesen, 2011), e.g. by pressing the olives together with the plants (Clodoveo et al., 2016). A number of plant extracts in oils have been shown to exert activity against Anisakis and the lethal time ranges from 1 day (cumin and a mixture of lemon, garlic and parsley), 3–8days [cinnamon (Cinnamomum verum), turmeric, cumin, rosemary], 10–15
days [lemon zest (Citrus lemon), laurel (Laurel nobilis), thyme, clove (Syzygium aromaticum), cardamom (Elettaria cardamomum), ginger] (Trabelsi et al., 2018, 2019, 2021). A number of these plant extracts were used in artificially parasitised anchovy fillets marinated in a typical industrial marinating process, and then stored in these flavoured oils. Based on the larvicidal activity and sensory scores, flavoured olive oils with cumin or with a mixture of lemon, parsley and garlic were considered good candidates for use in these traditional foods owing to their larvicidal activity without negatively affecting their sensory characteristics (Trabelsi et al., 2021).
The larvicidal activity of natural products induce alterations in the cuticle and digestive tract of anisakids. The effect of essential oils or plant extracts may be the result of several compounds acting synergically (Valero et al., 2015).
3.3.5. General considerations for physical and chemical treatments
The new physical (i.e. drying, PEF) and chemical (i.e. essential oils and plant extracts) approaches of inactivation of parasites, developed since 2010, are in the proof‐of‐concept phase of development or no sufficient data have been gathered. The new research data for technologies described in the previous opinion (heating, HPP, salting, marinating, natural compounds) have yet to clearly define the conditions required for complete inactivation of the parasites and describe the effect on sensory attributes. Within these studies, differences in methodologies, parasitic species, etc., prevent a comparison of the data when combined with inherent product factors such as fish composition, geometry, presence of other components, packaging, etc. It is essential that the efficacy of a given treatment has to be evaluated for a specific product. The application of chemical compounds requires them to be assessed for use on products that are intended for human consumption in addition to their ability to kill or inactivate the parasites. Whenever larval inactivation is measured in an efficient way (i.e. sufficient sample size in a short time) and well‐controlled conditions, quantitative risk models should be developed, as for other biological hazards (e.g. EFSA BIOHAZ Panel, 2015).
To date, freezing and heating are still the most efficient methods to kill Anisakis larvae. Several reports in the scientific literature show that the conditions of the freezing process can be designed for any fishery product to meet both safety and quality requirements (Cuesta et al., 2021; Della Morte et al., 2023; Podolska et al., 2019; Sánchez‐Alonso et al., 2020).
3.3.6. Strategies for parasite removal
Mechanical removal of parasites from infected parts is one of the most established ways of avoiding the presence of these parasites in the edible part of the fish. Early gutting will prevent migration of anisakids to the fish musculature, and filleting and trimming operations will decrease the parasite burden of the flesh.
In traditional fish processing, the operations are performed manually by experienced workers. Due to the advancement of cutting techniques in fish processing, advanced techniques for intelligent cutting and/or processing solutions are becoming available (Fu et al., 2023; Liu et al., 2022). Among them, gutting, which involves cutting and removing the viscera by washing out with water, extraction with claws or vacuum suction has a high importance in the removal of parasites, and is, for some species of high economic value, the first step that can be fully automated (Azarmdel et al., 2021).
Various studies indicate that trimming of the flesh by physically removing most of the belly flaps can considerably reduce the presence of anisakid larvae in the fillets of several commercially valuable fish species. For example, a recent investigation (Levsen et al., 2022) demonstrated that trimming by removing the belly flaps could reduce the presence of A. simplex (s. s.) larvae in the fillets by 86% in saithe (Pollachius virens), and even more than 90% in NE Arctic cod (Gadus morhua) and haddock (Melanogrammus aeglefinus). Similarly, trimming by removing the belly flaps could reduce the presence of Ph. decipiens (s. l.) in the flesh of NE Arctic cod by at least 45% (Levsen et al., 2022). A series of technological developments are available although better performance is usually achieved by hand cutting (Fu et al., 2023).
Anisakid nematodes respond to chemical and physical stimuli which can, in principle, be used as removal strategies from the fish matrices. The treatment of gutted and washed blue whiting with certain essential oils diluted in olive oil for 24 h at 4°C (i.e. Spanish lavender, cumin, lavender, marjoram, rosemary and thyme essential oils) resulted in ≥70% Anisakis L3 moving away from the fish muscle (Gómez‐Mateos Pérez et al., 2017). In another study, cod livers infected with C. osculatum L3 were immersed in solutions of different composition for up to 65 h at 37°C and the 24
mM HCl solution induced the escape rates >
80% after 35 h with slightly disintegrated, fragmented or soft tissues (Zuo et al., 2017). On the other hand, the incubation of Anisakis spp. and Phocanema spp. larvae with CO2 or O2 atmospheres as a procedure for their removal from the fish was shown to be unsuccessful (Guan et al., 2021).
3.3.7. Concluding remarks for TOR3
AQ3: What technical developments and new scientific data for inactivation and/or removal of viable parasites (identified in the answer to ToR1) in fishery products, in particular treatments other than freezing, have been described since the EFSA BIOHAZ 2010 scientific opinion?
Treatments to inactivate nematodes of the family Anisakidae (mostly for Anisakis spp.) in fishery products are being actively researched, but there are limited data for cestodes and trematodes.
The end point used in most of the inactivation studies is larval loss of mobility. There are new high‐throughput technological/instrumental solutions to observe mobility, but their use has been limited for the parasites covered in this opinion. Other in vitro tools related to larval behaviour and physiology are becoming available and may be used to characterise parasite infectivity.
To date, freezing and heating continue to be the most efficient methods to kill Anisakis larvae in fishery products when applied under well‐defined conditions.
New data on the thermal inactivation of A. simplex (s. s.) and Anisakis type I L3 in model systems heated in quasi‐isothermal conditions suggest a negative exponential relationship between lethal times and temperature. The susceptibility of Anisakis larvae (A. simplex (s. s.)) to isothermal lethal conditions could be described by the Weibull model. However, the actual lethal time/temperature combinations vary between experiments/species, and more data are needed both in model systems and fish products.
New reports on microwave heating have shown Anisakis larvae inactivation in fishery products at microwave conditions that achieved temperatures ranging from 60 to 76°C with exposure times of 5 min to 30 s, well within the temperature/time range known to thermally inactivate these nematodes. However, the lack of temperature homogeneity throughout the food inherent to this technology should be considered.
Traditional dry salting processes of anchovies successfully inactivated Anisakis. Studies on other traditional processes such as air‐drying of Arctic migrating cod (‘stockfish’) and double salting (salting in brine plus dry salting) of anchovies and cod (‘baccalà’), also suggest that anisakids are successfully inactivated. However, more data covering these and other parasites in more fish species and products is required to determine if these processes are always effective.
As some high‐pressure processing (HPP) pressure/time combinations reported corresponding to those commercially used for food products are successful in killing Anisakis larvae and maintaining the sensory characteristics of the fish, HPP could be used for specific fish products.
Pulsed electric field is considered a promising technology to inactivate Anisakis in fish products, but it needs further development before application.
Natural products (natural compounds, essential oils, plant extracts) have been applied to inactivate anisakids in food, but data on safety (toxicity) and organoleptic properties are lacking.
The results obtained with ultrasound treatment suggest that this method is not successful in killing Anisakis.
The marinade combinations with anchovies fillets have not proven successful despite in vitro solution‐based studies with isolated larvae have shown some effect.
Advanced processing techniques for intelligent cutting, which include gutting and trimming operations are becoming available and their implementation could have a significant impact on assuring the provision of parasite‐free products to consumers. Other strategies for the removal of parasites by chemical procedures are still in the proof‐of‐concept phase.
4. CONCLUSIONS
EFSA was requested to review and assess:
ToR1. The occurrence of parasites of public health importance in fishery products derived from the most relevant farmed fish species in the EU (in particular, but not limited to, Atlantic salmon, seabass, farmed seabream and turbot).
AQ1: What is the occurrence of parasites of public health importance in fishery products derived from the most relevant farmed finfish species in the EU/EFTA?
The most relevant farmed finfish species in the EU/EFTA, based on production and consumption data, are Atlantic salmon, rainbow trout, gilthead seabream, European seabass, Atlantic bluefin tuna, turbot, meagre, Atlantic cod, Atlantic halibut and greater amberjack in marine farming systems; and rainbow and brown trout, common carp, European and African catfish, European eel, tench and pikeperch in freshwater fish farming.
The parasites of public health importance occurring in the wild and with which farmed fish produced in EU/EFTA aquaculture could be infected, include:
in the marine environment the nematodes A. simplex (s. s.), A. pegreffii, Phocanema decipiens (s. l.) and Contracaecum osculatum (s. l.) as well as the trematode Cryptocotyle lingua;
in freshwater systems the trematodes Opisthorchis felineus, Metorchis spp., Pseudamphistomum truncatum, Paracoenogonimus ovatus and the cestode Dibothriocephalus spp.
The experts consider it to be 99%–100% certain (almost certain) that fish produced in recirculating aquaculture systems (RAS), or indoor or roofed facilities with filtered and/or treated water intake and exclusively fed with heat treated feed, are not exposed to zoonotic parasites. In contrast, fish farmed in marine offshore cages or flow‐through freshwater ponds or tanks are exposed to zoonotic parasites. However, to date there is no published evidence of infection in market quality Atlantic salmon and marine rainbow trout. There is similarly no published evidence of infection in gilthead seabream, turbot, meagre, Atlantic halibut, common carp or European catfish. Studies have not been published for brown trout, African catfish, European eel and pikeperch.
The published data currently available provide evidence of infection with zoonotic parasites in sea‐caged European seabass (A. pegreffii, A. simplex (s.s.)), Atlantic bluefin tuna (A. pegreffii, A. simplex (s.s.)) and Atlantic cod (C. lingua, A. simplex (s.l.)), as well as tench farmed in open pond systems (Pseudamphistomum truncatum and Paracoenogonimus ovatus).
Due to the lack of representative data, it is not possible to make informative estimates of the prevalence or the abundance of those parasites, that are considered to be of public health importance, for all fish species, farming systems and production area in the EU/EFTA.
ToR2: Diagnostic methods for the detection of parasites of public health importance in fishery products from such farmed fish species.
AQ2: What testing methods are currently available and may be available in the near future to test the fish species for the parasites identified in the answer to ToR1?
Since the last EFSA opinion (EFSA BIOHAZ Panel, 2010), some methods such as UV‐press and artificial digestion methods developed into ISO standards, whereas new technologies and methodologies have been developed for the detection, visualisation and isolation of zoonotic parasites in fish products and for the specific identification of the isolated parasites.
New UV‐scanning devices have been developed and are in use for the detection of Anisakis in fishery products. Novel optical (hyperspectral) sensing methodologies have also been developed and have found limited application in the industry, but further development and validation are required.
The molecular identification of isolated parasites from fishery products has been significantly improved by the application of PCR‐amplification and sequencing methods targeting both nuclear and mitochondrial sequences in the parasites. These genetic/molecular approaches (applied together with the use of microscopic identification techniques) should be regarded as the most reliable identification methods.
OMICs (genomics, metagenomics, transcriptomics, and proteomics) generated data are a useful resource for the selection of further molecular/genetic markers to be used for the identification and characterisation of zoonotic parasites.
The application of artificial intelligence and machine learning algorithms in image and video processing are also being tested for the high throughput detection and identification of parasites in fish.
ToR3. Technical developments and new scientific data available in relation to killing viable parasites of public health importance in fishery products, in particular treatments other than freezing.
AQ3. What technical developments and new scientific data for inactivation and/or removal of viable parasites (identified in the answer to ToR1) in fishery products, in particular treatments other than freezing, have been described since the EFSA BIOHAZ 2010 scientific opinion?
To date, freezing and heating continue to be the most efficient methods to kill Anisakis larvae in fresh fishery products.
In model systems attempts to quantitatively characterise the thermal inactivation have shown that a negative exponential relationship exists between lethal times and heating temperature. The susceptibility of Anisakis larvae to isothermal lethal conditions could be described by the Weibull model. However, the actual lethal combinations vary among studies and parasite species, and more data are needed both in model systems and real food products.
Traditional dry salting processes of anchovies successfully inactivated Anisakis. Studies on other traditional processes such as air drying of Arctic migrating cod (‘stockfish’) and double salting (salting in brine plus dry salting) of anchovies and cod (‘baccalà’), also suggest that anisakids are successfully inactivated. However more data covering these and other parasites in more fish species and products is required to determine if these processes are always effective.
Most of the studies on other inactivation methods have reported killing the parasites under defined laboratory conditions but further research is required to verify these findings especially under commercial conditions. In particular, pressure/time combinations used in industrial high pressure processing (HPP) could be applied in some specific products, and pulsed electric field (PEF) is considered a promising technology, although further development is needed. Also, the use of natural products could have some applications but data on safety and organoleptic properties is lacking.
Ultrasound treatment, and marinade combinations with anchovies fillets, have not proven successful, despite in vitro solution based studies with isolated larvae that have shown some effect.
Advanced processing techniques for intelligent gutting and trimming operations could have a significant impact on removing parasites from fish in the future.
5. RECOMMENDATIONS
When available, information on the fish origin (farmed or wild) should be included in the RASFF notifications on parasites in fishery products.
A baseline survey should be undertaken at the processing stage to address the data gaps on the occurrence of zoonotic parasites in fish species which are commonly produced in open systems. More regular epidemiological studies should be undertaken, especially of fish species exempt from freezing.
ISO methods such as ISO 23036‐1:2021 (UV‐ press) and ISO 23036‐2:2021 (artificial digestion) should be used for the detection of parasites in fisheries products in official control programmes.
Methods to visualise and isolate parasites must be complemented whenever possible by molecular methods for parasite identification (e.g. sequencing of nuclear and mitochondrial DNA gene loci).
Further research and development should focus on:
For detection methods:
improvement of real time non‐destructive (e.g. optical sensing methods) or destructive (UV‐press) automatic parasite detection systems with higher samples throughput;
validation of molecular detection methods using multiple markers for the accurate identification of the parasite at species level;
generation of more OMICs‐based data (e.g. genomics, metagenomics, transcriptomics and proteomics), focusing on all the zoonotic parasites for the selection of markers to be used for their detection, identification and characterisation.
For inactivation methods:
further predictive modelling approaches on anisakids, e.g. on thermal inactivation to provide safe cooking processes with minimal impact on the product quality, should be done (e.g. by coupling the momentary isothermal survival rate and the time–temperature profiles within the sample fish matrix, either known or modelled by using heat transfer simulation tools);
treatments to kill cestodes and trematodes;
further development of high‐throughput phenotypic screening methods to assess parasites infectivity;
as above, generation of more OMICs‐based data to select markers for inactivation studies;
optimisation of inactivation treatments for individual fishery preparations.
ABBREVIATIONS
- AITC
- Allylisothiocyanate
- AQ
- Assessment question
- ARMS‐PCR
- Amplification‐refractory mutation system‐PCR
- BIOHAZ
- Biological Hazards
- CaFO
- Tunisian enriched olive oil with cardamom
- CI95
- 95% Confidence Interval
- CID
- Collision‐induced dissociation
- CiFO
- Tunisian enriched olive oil with cinnamon
- Cm‐FOO
- Olive oil flavoured with cumin
- COMEXT
- Eurostat reference database for international trade in goods
- CRISPR‐Cas9
- Clustered regularly interspaced palindromic repeats ‐Cas9 technology
- CT
- Computed tomography
- DNA
- Deoxyribonucleic acid
- EA
- Extra‐gastrointestinal or ectopic anisakiasis
- EC
- European Commission
- ECDC
- European Centre for Disease Prevention and Control
- EF
- Elongation factor
- EFSA
- European Food Safety Authority
- EFTA
- European Food Trade Association
- ELISA
- Enzyme‐linked immunosorbent assay
- ENA
- European Nucleotide Archive
- EO
- Essential oil
- ESP
- Excretory/Secretory products
- EU
- European Union
- EUMOFA
- European market observatory for fisheries and aquaculture products
- EURL
- European Reference Laboratory
- EUROSTAT
- Statistical office of the European Union
- EVs
- Extracellular vesicles
- FAO
- Food and Agriculture Organisation
- FBS
- Foetal bovine serum
- FCCP
- Carbonyl cyanide 4‐trifluoromethoxy
- FHF
- Norwegian Seafood Research Fund
- FOO
- Olive oil flavoured
- GA
- Gastric anisakiasis
- GAA
- Gastro‐allergic anisakiasis
- GiFO
- Tunisian enriched olive oil with ginger
- GLOBEFISH‐FAO
- Multi‐donor funded project within the FAO Fisheries Division
- HPLC
- High‐performance liquid chromatography
- HPP
- High pressure processing
- HS
- Hyperspectral
- IA
- Invasive anisakiasis
- Ig
- Immunoglobulin
- ILT
- Infrared light locomotion
- ITS
- Internal transcribed spacers
- KCL
- Potassium chloride
- LaFO
- Tunisian enriched olive oil with laurel
- LAMP
- Loop‐mediated isothermal amplification
- LC
- Liquid chromatography
- LC50
- Lethal concentration 50, or concentration that causes 50% inactivation
- LC‐ESI‐MS
- Liquid chromatography‐electrospray ionisation‐mass spectrometry
- LC–MS
- Liquid chromatography‐mass spectrometry
- LMN
- R(+) Limonene
- LOD
- Limit of detection
- LT100
- 100% Lethal time
- LTQ XL™
- LTQ XL™ Linear ion trap mass spectrometer
- LWE
- Live weight equivalent
- MALDI‐TOF MS
- Matrix assisted laser desorption ionisation‐time of flight mass spectrometry
- min
- minute
- Mix‐FOO
- Olive oil flavoured with a mixture with lemon, parsley, and fresh garlic (10%)
- MPa
- Megapascal
- MRI
- Magnetic resonance imaging
- MS
- Mass spectrometry
- mtDNA
- mitochondrial DNA
- MW
- Megawatt
- NA
- Non available
- NaCl
- Sodium chloride
- NCBI
- National Center for Biotechnology Information
- NE
- Northeast
- NGS
- Next generation sequencing
- NPV
- Negative predicted value
- PCR
- Polymerase chain reaction
- PEF
- Pulsed electric fields
- PRM
- Parallel reaction monitoring
- qPCR
- quantitative PCR
- RAS
- Recirculating aquaculture system
- RASFF
- Rapid alert system for food and feed
- rDNA
- ribosomal DNA
- RFLP
- Restriction fragment length polymorphism
- RoFO
- Tunisian enriched olive oil with rosemary
- RPA
- Recombinase polymerase amplification
- RPMI‐1640
- Roswell Park Memorial Institute cell culture medium
- RT
- Room temperature
- s.l.
- sensu lato
- s.s.
- sensu stricto
- SEM
- Scanning electron microscopy
- SNP
- Single nucleotide polymorphism
- SO
- Seed oil
- Spp.
- species
- SQ
- Subassessment question
- SSRs
- Simple sequence repeats
- syn.
- synonym
- TDT
- Thermal death time
- TEO
- Tagetes minuta essential oil
- TMT
- Tandem mass tag
- ToR
- Term of reference
- TTO
- Tea tree (Melaleuca alternifolia) essential oil
- UK
- United Kingdom
- US
- ultrasound
- UV
- Ultraviolet
- UV‐Press
- Ultraviolet press
- W
- Watt
- WG
- working group
CONFLICT OF INTEREST
If you wish to access the declaration of interests of any expert contributing to an EFSA scientific assessment, please contact ue.aporue.asfe@tnemeganamtseretni.
COPYRIGHT FOR NON‐EFSA CONTENT
EFSA may include images or other content for which it does not hold copyright. In such cases, EFSA indicates the copyright holder and users should seek permission to reproduce the content from the original source.
PANEL MEMBERS
Ana Allende, Avelino Alvarez‐Ordóñez, Declan Bolton, Sara Bover‐Cid, Marianne Chemaly, Alessandra De Cesare, Lieve Herman, Friederike Hilbert, Konstantinos Koutsoumanis, Roland Lindqvist, Maarten Nauta, Romolo Nonno, Luisa Peixe, Giuseppe Ru, Marion Simmons, Panagiotis Skandamis, and Elisabetta Suffredini.
Supporting information
Protocol for the re‐evaluation of certain aspects of the EFSA Scientific Opinion of April 2010 on risk assessment of parasites in fishery products, based on new scientific data. Part 1: ToRs1–3
Data on human outbreaks and related human cases caused by Anisakis during the period extracted from the EFSA zoonoses and food‐borne outbreaks database
Data on consumption of fish products during the period 01 January 2010 to 31 December 2023 extracted from the EFSA Comprehensive Food Consumption Database
Literature searches and search strategy
ACKNOWLEDGEMENTS
The Panel wishes to thank the following for the support provided to this scientific output: EFSA staff: Isabelle Delaunois, Frank Boelaert, Eleonora Sarno; EC DG‐SANTE RASFF: Enrique Beltran; as well as the Pathogens in Food Database consortium (EFSA grant agreement GP/EFSA/BIOHAW/2023/05).
ANNEX A. Protocol for the re‐evaluation of certain aspects of the EFSA Scientific Opinion of April 2010 on risk assessment of parasites in fishery products, based on new scientific data. Part 1: ToRs1–3
A.1.
Annex A can be found in ‘supporting information’ section in the online version of this output.
ANNEX B. Data on notifications of parasites in food products from 01 January 2010 to 31 December 2023 extracted from the Rapid Alert System for Food and Feed (RASFF platform)
B.1.
Annex B can be found in ‘supporting information’ section in the online version of this output.
ANNEX C. Data on human outbreaks and related human cases caused by Anisakis during the period extracted from the EFSA zoonoses and food‐borne outbreaks database
C.1.
Annex C can be found in ‘supporting information’ section in the online version of this output.
ANNEX D. Data on consumption of fish products during the period 01 January 2010 to 31 December 2023 extracted from the EFSA Comprehensive Food Consumption Database
D.1.
Annex D can be found in ‘supporting information’ section in the online version of this output.
ANNEX E. Literature searches and search strategy
E.1.
Annex E can be found in ‘supporting information’ section in the online version of this output.
APPENDIX A. Production volume (tonnes), per capita apparent consumption and common farming and feeding practices of the most important farmed finfish species in Europe, by entity (EU) or country
A.1.
TABLE A.1
Production volume (tonnes), per capita apparent consumption and common farming and feeding practices of the most important farmed finfish species in Europe, by entity (EU) or country.
Fish species common name, Latin name a | Production volume (tonnes) by entity or country in 2021 | References | Apparent consumption most consumed fishery and aquaculture finfish (2021) b | Common farming practices | Common feeding practices | References | ||||
---|---|---|---|---|---|---|---|---|---|---|
Per capita consumption in kg, LWE (% of it farmed) | Larval stage | Juveniles/smolts | Grow‐out phase | Larval stage | Juveniles and adults | |||||
Marine |
Atlantic salmon Salmo salar Linnaeus, 1758 |
EU: 14,900, with 86% produced in Ireland Norway: 1,546,000 Iceland: 47,000 Faroe Islands: 95,000 |
EUMOFA Fish market report (EC DG‐MARE, 2023) EUMOFA species profile: Atlantic salmon https://www.fiskeridir.no/English/Aquaculture/Statistics/Booklets https://www.statice.is/publications/news‐archive/fisheries/aquaculture‐2021/ https://www.faroeseseafood.com/fishery‐aquaculture/stats/stats‐2021 | Salmon: 2.6 kg (94.4%) | Freshwater RAS or flow‐through hatchery tanks | Closed freshwater tanks | Offshore sea cages | Artificially reared live feed | Heat‐treated pelleted feed c | https://www.fao.org/fishery/en/culturedspecies/salmo_salar/en |
Marine or freshwater |
Rainbow trout Oncorhynchus mykiss (Walbaum, 1792) |
EU: 190, 150, with 22%, 18% and 14% produced in Italy, France and Denmark Norway: 89,000 Iceland: 1000 UK: 13.500 |
EUMOFA Fish market report (EC DG‐MARE, 2023) EUMOFA Species profile: rainbow trout https://www.fiskeridir.no/English/Aquaculture/Statistics/Booklets https://www.statice.is/publications/news‐archive/fisheries/aquaculture‐2021/ | Trout: 0.49 (98.4%) | Freshwater RAS or flow‐through hatchery tanks | Closed freshwater tanks or flow‐through ponds | Offshore sea cages (marine), or RAS‐based or flow‐through tanks or earth ponds (freshwater) | Artificially reared live feed or heat‐treated pelleted feed | Heat‐treated pelleted feed c | https://www.fao.org/fishery/en/culturedspecies/oncorhynchus_mykiss/en |
Marine |
Gilthead seabream Sparus aurata Linnaeus, 1758 | EU: 97,100, with 70% produced in Greece, 8% each in Italy and Croatia and 5% in Cyprus | EUMOFA Fish market report (EC DG‐MARE, 2023) | RAS or flow‐through hatchery tanks | RAS or flow‐through onshore tanks | RAS‐based or flow‐through onshore tanks or sea cages in brackish water lagoons, often co‐cultured with seabream | Artificially reared live feed | Heat‐treated pelleted feed b | https://www.fao.org/fishery/en/culturedspecies/sparus_aurata/en | |
Marine |
European seabass Dicentrarchus labrax (Linnaeus, 1758) | EU: 97,090, with Greece and Spain providing 77% |
EUMOFA Fish market report (EC DG‐MARE, 2023) EUMOFA species profile: European seabass | – | RAS or flow‐through hatchery tanks | RAS or flow‐through onshore tanks | RAS‐based or flow‐through onshore tanks or sea cages in brackish water lagoons, often co‐cultured with seabass | Artificially reared live feed | Heat‐treated pelleted feed c | https://www.fao.org/fishery/en/culturedspecies/dicentrarchus_labrax/en |
Marine |
Atlantic bluefin tuna Thunnus thynnus (Linnaeus, 1758) | EU: 17,921, with 75.6% produced in Malta and 24.4% in Croatia | https://eumofa.eu/aquaculture | Tuna: 286 (0.6%) | Largely based on fattening of wild‐caught juveniles or young adults in sea cages in the Mediterranean. To a lesser extent, production is based on stocking of sea cages with hatchery‐reared juveniles | Planktonic organisms and live fish feed | Frozen baitfish or pelleted dry feed | https://www.fao.org/fishery/en/culturedspecies/thunnus_thynnus/en | ||
Marine | Turbot Scophthalmus maximus (Linnaeus, 1758) | EU: 11.973, with 71% and 28% produced in Spain and Portugal, respectively | https://eumofa.eu/aquaculture | – | RAS or flow‐through hatchery tanks | RAS or flow‐through onshore tanks | Flow‐through onshore tanks or flat‐bottomed sea cages | Artificially reared live feed | Heat‐treated pelleted feed c | https://www.fao.org/fishery/en/culturedspecies/psetta_maxima/en |
Marine | Meagre Argyrosomus regius (Asso, 1801) | EU: 8400 (2019), with 76% produced in Spain and Greece, followed by France, Croatia and Portugal with 5% each | EUMOFA Case study, Meagre in the EU (2022) | – | RAS or flow‐through hatchery tanks | RAS or flow‐through onshore tanks | RAS‐based or flow‐through onshore tanks or sea cages in brackish water lagoons | Artificially reared live feed | Heat‐treated pelleted feed c | https://www.fao.org/fishery/en/culturedspecies/argyrosomus_regius/en |
Marine |
Atlantic halibut Hippoglossus hippoglossus (Linnaeus, 1758) |
Norway: 2716 UK: N/A | https://www.fiskeridir.no/English/Aquaculture/Statistics/Booklets | – | RAS or flow‐through hatchery tanks | RAS or flow‐through onshore tanks | Flat‐bottomed sea cages or, to a lesser extent, closed onshore RAS‐facilities | Artificially reared live feed | Heat‐treated pelleted feed c | https://www.seafish.org/responsible‐sourcing/aquaculture‐farming‐seafood/species‐farmed‐in‐aquaculture/aquaculture‐profiles/atlantic‐halibut/ |
Marine |
Atlantic cod Gadus morhua Linnaeus, 1758 |
Norway: 1622 EU: 350 |
EUMOFA species profile: Atlantic cod https://www.fiskeridir.no/English/Aquaculture/Statistics/Booklets | 1.75 (0.1%) | RAS or flow‐through hatchery tanks | RAS or flow‐through onshore tanks | Offshore sea cages | Artificially reared live feed | Heat‐treated pelleted feed c | https://www.fao.org/fishery/en/culturedspecies/gadus_morhua/en |
Marine |
Greater amberjack Seriola dumerili (Risso, 1810) | EU: 312. Main producer is Malta, with only minor production in Spain, Greece, Italy, Croatia and Cyprus | https://eumofa.eu/aquaculture | – | RAS or flow‐through hatchery tanks | RAS or flow‐through onshore tanks | Offshore sea cages or, to a lesser extent, onshore RAS‐facilities | Microalgae, rotifers, Artemia, pelleted food | Heat‐treated pelleted feed c or frozen sardines | https://www.fao.org/fishery/en/culturedspecies/seriola_dumerili/en |
Freshwater |
Brown trout Salmo trutta Linnaeus, 1758 | EU: included in stats for rainbow trout | EU: included in stats for rainbow trout | – | RAS or flow‐through hatchery tanks | Closed tanks | RAS‐based or flow‐through, tanks or earth ponds | Heat‐treated pelleted feed | Heat‐treated pelleted feed c | https://britishtrout.co.uk/about‐trout/trout‐farming/ |
Freshwater | Common carp Cyprinus carpio Linnaeus, 1758 | EU: 77.511, with 24%, 24% and 16% produced in Poland, Czechia and Hungary, respectively | EUMOFA Fish market report (EC DG‐MARE, 2023) | – | Hatchery tanks or spawning ponds | Nursery ponds or RAS/flow through‐facilities | Earth ponds or RAS/flow through‐facilities | Artificially or naturally (in spawning ponds) reared live feed or fine‐grained pelleted feed | Heat‐treated pelleted feed c | https://www.fao.org/fishery/en/culturedspecies/cyprinus_carpio/en |
Freshwater | European eel Anguilla anguilla (Linnaeus, 1758) | EU: 5.102, with 39%, 23% and 23% produced in the Netherlands, Germany and Denmark, respectively | EUMOFA Fish market report (EC DG‐MARE, 2023) | – | RAS‐based facilities are stocked with wild‐caught glass‐eels (around 0.2 g body weight) | Following feeding with pre‐frozen cod roe, eels are fed heat‐treated pelleted feed c | https://www.fao.org/fishery/en/culturedspecies/anguilla_anguilla/en | |||
Freshwater |
European catfish Silurus glanis Linnaeus, 1758 |
Farming production of European catfish, African catfish, tench and pikeperch is currently at a relatively low level in the EU Catfish (both species): 11,000, with 39.3%, 22.2% and 8.6% produced in Hungary, the Netherlands and Germany, respectively, as main producers Tench: 700 (mostly in France) Pikeperch: 641 (Denmark) | https://eumofa.eu/aquaculture | – | Hatchery tanks | Earth ponds or RAS‐facilities | Pelleted feed only | Heat‐treated pelleted feed c | ||
African catfish Clarias gariepinus Burchell, 1822 | – | Hatchery tanks | RAS‐facilities (in Europe) | Pelleted feed only | Heat‐treated pelleted feed c | |||||
Tench Tinca tinca (Linnaeus, 1758) | – | Released into ponds post‐hatching | Largely in extensive pond systems in central, eastern and southern Europe, sometimes in polyculture with common carp | As for Common carp | Heat‐treated pelleted feed c | |||||
Pikeperch Sander lucioperca (Linnaeus, 1758) | – | Hatchery tanks | Largely in RAS‐facilities, only minor production in traditional ponds systems | Heat‐treated pelleted feed/live feed may be used | Heat‐treated pelleted feed c | https://www.fao.org/fishery/en/culturedspecies/sander_lucioperca/en |
Abbreviations: LWE, live weight equivalent; N/A, not applicable; RAS, recirculating aquaculture system.


Notes
EFSA BIOHAZ Panel (EFSA Panel on Biological Hazards) , Koutsoumanis K., Allende A., Alvarez‐Ordóñez A., Bover‐Cid S., Chemaly M., De Cesare A., Herman L., Hilbert F., Lindqvist R., Nauta M., Nonno R., Peixe L., Ru G., Simmons M., Skandamis P., Suffredini E., Buchmann K., Careche M., … Bolton D. (2024). Re‐evaluation of certain aspects of the EFSA Scientific Opinion of April 2010 on risk assessment of parasites in fishery products, based on new scientific data. Part 1: ToRs1–3. EFSA Journal, 22(4), e8719. 10.2903/j.efsa.2024.8719 [Europe PMC free article] [Abstract] [CrossRef] [Google Scholar]
Adopted: 13 March 2024
Note: A plain language summary of this scientific opinion is available at https://efsa.onlinelibrary.wiley.com/doi/10.2903/j.efsa.2024.p220401
This article was originally published on the EFSA website www.efsa.europa.eu on 18 April 2024 as part of EFSA's urgent publication procedures.
Annexes A, C, D and E are available under the Supporting Information section .
Notes
1 https://efsa.onlinelibrary.wiley.com/doi/epdf/10.2903/j.efsa.2010.1543
2 Regulation (EC) No 853/2004 of the European Parliament and of the Council of 29 April 2004 laying down specific hygiene rules for food of animal origin. OJ L 139, 30.4.2004, p. 55–205.https://eur‐lex.europa.eu/legal‐content/EN/TXT/PDF/?uri=CELEX:32004R0853. Consolidated text from 15 February 2023: https://eur‐lex.europa.eu/legal‐content/EN/TXT/PDF/?uri=CELEX:02004R0853‐20230215
3 EFTA: includes Iceland, Liechtenstein, Norway and Switzerland. Faroe Islands (Denmark Kingdom) do not belong to the EU. Formal relations between the EU and the Faroe Islands are currently based on three separate bilateral agreements dealing with fisheries, trade in goods and scientific and technological cooperation. The United Kingdom (UK) left the European Union on 31 January 2020. These last two areas will be included based on their relevance in aquaculture production and imports into the EU. For all data presented in this scientific opinion, the “EU” acronym refers to 28 Member States including UK until 31 January 2020 and to 27 Member States from 1 February on.
4 Bao et al. (2023) proposed the resurrection of the genus Phocanema Myers, 1959, for the species of the Pseudoterranova decipiens (s.l.) complex: Phocanema decipiens (krabbei, 1878) Myers,1959; Phocanema krabbei (Paggi, Mattiucci, Gibson, Berland, Nascetti, Cianchi Bullini, 2000), Bao, Giulietti, Levsen, Karlsbakk, 2023; Phocanema bulbosum (Cobb, 1889) Bao, Giulietti, Levsen, Karlsbakk, 2023; Phocanema azarasi (Yamaguti and Arima, 1942) Bao, Giulietti, Levsen, Karlsbakk, 2023; Phocanema cattani (George Nascimento and Urritia, 2000) Bao, Giulietti, Levsen, Karlsbakk, 2023. For the current scientific opinion, we follow this nomenclature, independently of the names referred to in the original article.
5 https://food.ec.europa.eu/safety/rasff_en. Data included in Annex B were provided to EFSA by colleagues of the RASFF Team.
7 https://ec.europa.eu/eurostat
8 https://ec.europa.eu/eurostat/web/fisheries/overview
9 https://ec.europa.eu/eurostat/statistics‐explained/index.php?title=Aquaculture_statistics
10 https://eumofa.eu/dashboards
11 https://eumofa.eu/en/market‐analysis
12 https://www.fiskeridir.no/English/
14 https://www.oecd.org/agriculture/topics/fisheries‐and‐aquaculture/documents/report_cn_fish_gbr.pdf
16 https://www.efsa.europa.eu/en/data‐report/food‐consumption‐data
17 https://cordis.europa.eu/project/id/312068
20 EFSA's grant agreement GP/EFSA/BIOHAW/2023/05 (Pathogens in foods database: extension with Vibrio and parasites) with as beneficiaries Instituto Politécnico de Bragança (IPB) as coordinator and Agence nationale de sécurité sanitaire de l'alimentation, de l'environnement et du travail (Anses) as co‐beneficiary.
21 https://www.statice.is/publications/news‐archive/fisheries/aquaculture‐2021/
22 https://www.faroeseseafood.com/fishery‐aquaculture/stats/stats‐2021
23 https://www.fao.org/fishery/en/facp/GBR
24 https://www.seafish.org/seafood‐in‐numbers/aquaculture/
25 Following the nomenclature included in the WORMS database, https://www.marinespecies.org/
26 https://www.vesteralenhavbruk.com/uploads/documents/Fiskehelserapport_Akerbla_mai.pdf
27 https://www.fiskeridir.no/Yrkesfiske/Tall‐og‐analyse/Fangst‐og‐kvoter/Fangst/Fangst‐av‐leppefisk
28 https://cordis.europa.eu/project/id/312068/reporting/es
29 https://www.warf.org/technologies/summary/P220194WO01/
30 Also referred as high‐hydrostatic pressure processing (HHP) or ultra‐high‐pressure processing (UHP).
REFERENCES
- Abad V., Alejandre M., Hernández‐Fernández E., Raso J., Cebrián G., & Álvarez‐Lanzarote I. (2023). Evaluation of pulsed electric fields (PEF) parameters in the inactivation of Anisakis larvae in saline solution and hake meat. Food, 12(2), 264. 10.3390/foods12020264 [Europe PMC free article] [Abstract] [CrossRef] [Google Scholar]
- Abattouy N., Valero A., Romero M. C., Martín‐Sánchez J., González‐Tejero M. R., Lozano J., & Navarro M. C. (2010). In vivo activity of essential oil Origanum elongatum against larva L3 of Anisakis pegreffii . Ars Pharmaceutica, 51, 107–111. https://www.researchgate.net/publication/287638456 [Google Scholar]
- Aco‐Alburqueque R. P. M., Andolfi R., Gabrielli S., Santoro M., & Mattiussi S. (2022). Blastocystis in the “sentinel” organism Mytilus galloprovincialis in the central Tyrrhenian Sea: A bioindicator of marine environment contamination. Proceedings of the XXXII Congresso della Società Italiana di Parassitologia (SoIPa), Naples, 277 pp.
- Akçar H. H., & Gümüşkesen A. S. (2011). Sensory evaluation of flavored extra virgin olive oil. Gida, 36, 249–253. https://www.researchgate.net/publication/285777397 [Google Scholar]
- Anastasio A., Smaldone G., Cacace D., Marrone R., Lo Voi A., Santoro M., Cringoli G., & Pozio E. (2016). Inactivation of Anisakis pegreffii larvae in anchovies (Engraulis encrasicolus) by salting and quality assessment of finished product. Food Control, 64, 115–119. 10.1016/j.foodcont.2015.12.026 [CrossRef] [Google Scholar]
- Anza M., Endale M., Cardona L., Cortes D., Cabedo N., Trelis M., Fuentes M. V., & Abarca B. (2021). Chemical composition, cytotoxicity and larvicidal activity of essential oils of three medicinal plants of Ethiopian flora against Anisakis L3 larvae. Research Journal of Pharmacognosy, 8, 21–30. 10.22127/rjp.2021.291618.1719 [CrossRef] [Google Scholar]
- APROMAR . (2012). Evaluación de la presencia de nemátodos del género Anisakis en los pescados de acuicultura marina españoles. https://hdl.handle.net/10261/257314
- Arai T., Akao N., Seki T., Kumagai T., Ishikawa H., Ohta N., Hirata N., Nakaji S., Yamauchi K., Hirai M., Shiratori T., Kobayashi M., Fujii H., Ishii E., Naito M., Saitoh S., Yamaguchi T., Shibata N., Shimo M., & Tokiwa T. (2014). Molecular genotyping of Anisakis larvae in middle eastern Japan and endoscopic evidence for preferential penetration of normal over atrophic mucosa. PLoS One, 9, e89188. 10.1371/journal.pone.0089188 [Europe PMC free article] [Abstract] [CrossRef] [Google Scholar]
- Arcos S. C., Ciordia S., Roberston L., Zapico I., Jiménez‐Ruiz Y., González‐Muñoz M., Moneo I., Carballeda‐Sangiao N., Rodríguez‐Mahillo A., Albar J. P., & Navas A. (2014). Proteomic profiling and characterization of differential allergens in the nematodes Anisakis simplex sensu stricto and A. Pegreffii . Proteomics, 14, 1547–1568. 10.1002/pmic.201300529 [Abstract] [CrossRef] [Google Scholar]
- Arcos S. C., Robertson L., Ciordia S., Sánchez‐Alonso I., Careche M., Carballeda‐Sanguiao N., González‐ Muñoz M., & Navas A. (2020). Quantitative proteomics comparison of total expressed proteomes of Anisakis simplex sensu stricto, A. Pegreffii, and their hybrid genotype. Genes, 11(8), 913. 10.3390/genes11080913 [Europe PMC free article] [Abstract] [CrossRef] [Google Scholar]
- Arizono N., Miura T., Yamada M., Tegoshi T., & Onishi K. (2011). Human infection with Pseudoterranova azarasi roundworm. Emerging Infectious Diseases, 17, 555–556. 10.3201/eid1703.101350 [Europe PMC free article] [Abstract] [CrossRef] [Google Scholar]
- Arizono N., Yamada M., Tegoshi T., & Yoshikawa M. (2012). Anisakis simplex sensu stricto and Anisakis pegreffii: Biological characteristics and pathogenetic potential in human anisakiasis. Foodborne Pathogens and Disease, 9, 517–521. 10.1089/fpd.2011.1076 [Abstract] [CrossRef] [Google Scholar]
- Auer H., Leskowschek H., Engler J., Leitner G., Wentzel C., Wolkerstorfer W., & Schneider R. (2007). Epidemiology and nosology of anisakiosis, a rather rare helminthozoonosis in Central Europe–two case reports. Wiener Klinische Wochenschrift, 119, 106–109. 10.1007/s00508-007-0866-4 [Abstract] [CrossRef] [Google Scholar]
- Azarmdel H., Mohtasebi S. S., Jafary A., Behfar H., & Rosado Muñoz A. (2021). Design and simulation of a vision‐based automatic trout fish‐processing robot. Applied Sciences, 11, 5602. 10.3390/app11125602 [CrossRef] [Google Scholar]
- Babbott F. L., Frye W. W., & Gordon J. E. (1961). Intestinal parasites of man in Arctic Greenland. The American Journal of Tropical Medicine and Hygiene, 10, 185–190. 10.4269/ajtmh.1961.10.185 [Abstract] [CrossRef] [Google Scholar]
- Baird F. J., Su X., Aibinu I., Nolan M. J., Sugiyama H., Otranto D., Lopata A. L., & Cantacessi C. (2016). The Anisakis transcriptome provides a resource for fundamental and applied studies on allergy‐causing parasites. PLoS Neglected Tropical Diseases, 10(7), e0004845. 10.1371/journal.pntd.0004845 [Europe PMC free article] [Abstract] [CrossRef] [Google Scholar]
- Bao M., Cipriani P., Giulietti L., Roiha I. S., Paoletti M., Palomba M., & Levsen A. (2020). Air‐dried stockfish of Northeast Arctic cod do not carry viable anisakid nematodes. Food Control, 116, 107322. 10.1016/j.foodcont.2020.107322 [CrossRef] [Google Scholar]
- Bao M., Giulietti L., Levsen A., & Karlsbakk E. (2023). Resurrection of genus Phocanema Myers, 1959, as a genus independent from Pseudoterranova Mozgovoĭ, 1953, for nematode species (Anisakidae) parasitic in pinnipeds and cetaceans, respectively. Parasitology International, 97, 102794. 10.1016/j.parint.2023.102794 [Abstract] [CrossRef] [Google Scholar]
- Bao M., Strachan N. J., Hastie L. C., MacKenzie K., Seton H. C., & Pierce G. J. (2017). Employing visual inspection and magnetic resonance imaging to investigate Anisakis simplex s.l. infection in herring viscera. Food Control, 75, 40–47. 10.1016/j.foodcont.2016.12.030 [CrossRef] [Google Scholar]
- Barja J. L., & Toranzo A. E. (1993). Myoliquefaction post‐mortem caused by the myxosporean Kudoa thyrsites in reared Atlantic salmon in Spain. Bulletin of the European Association of Fish Pathologists, 13, 86. [Google Scholar]
- Barros L. A., Yamanaka A. R., Silva L. E., Vanzeler M. L., Braum D. T., & Bonaldo J. (2009). In vitro larvicidal activity of geraniol and citronellal against Contracaecum sp (Nematoda: Anisakidae). Brazilian Journal of Medical and Biological Research, 42, 918–920. 10.1590/S0100-879X2009005000023 [Abstract] [CrossRef] [Google Scholar]
- Bello E., Palomba M., Webb S. C., Paoletti M., Cipriani P., Nascetti G., & Mattiucci S. (2021). Investigating the genetic structure of the parasites Anisakis pegreffii and A. Berlandi (Nematoda: Anisakidae) in a sympatric area of the southern Pacific Ocean waters using a multilocus genotyping approach: First evidence of their interspecific hybridization. Infection, Genetics and Evolution, 92, 104887. 10.1016/j.meegid.2021.104887 [Abstract] [CrossRef] [Google Scholar]
- Bello E., Paoletti M., Webb S. C., Nascetti G., & Mattiucci S. (2020). Cross‐species utility of microsatellite loci for the genetic characterisation of Anisakis berlandi (Nematoda: Anisakidae). Parasite, 27, 9. 10.1051/parasite/2020004 [Europe PMC free article] [Abstract] [CrossRef] [Google Scholar]
- Borges J. N., Skov J., Bahlool Q. Z. M., Møller O. S., Kania P. W., Santos C. P., & Buchmann K. (2015). Viability of Cryptocotyle lingua metacercariae from Atlantic cod (Gadus morhua) after exposure to freezing and heating in the temperature range from −80°C to 100°C. Food Control, 50, 371–377. 10.1016/j.foodcont.2014.09.021 [CrossRef] [Google Scholar]
- Bouymajane A., Filali F. R., Ed‐Dra A., Aazza M., Nalbone L., Giarratana F., Alibrando F., Miceli N., Mondello L., & Cacciola F. (2022). Chemical profile, antibacterial, antioxidant, and anisakicidal activities of thymus zygis subsp. gracilis essential oil and its effect against listeria monocytogenes . International Journal of Food Microbiology, 383, 109960. 10.1016/j.ijfoodmicro.2022.109960 [Abstract] [CrossRef] [Google Scholar]
- Boysen A. T., Whitehead B., Stensballe A., Carnerup A., Nylander T., & Nejsum P. (2020). Fluorescent labeling of helminth extracellular vesicles using an in vivo whole organism approach. Biomedicine, 8(7), 213. 10.3390/biomedicines8070213 [Europe PMC free article] [Abstract] [CrossRef] [Google Scholar]
- Broglia A., & Kapel C. (2011). Changing dietary habits in a changing world: Emerging drivers for the transmission of foodborne parasitic zoonoses. Veterinary Parasitology, 182, 2–13. 10.1016/j.vetpar.2011.07.011 [Abstract] [CrossRef] [Google Scholar]
- Brooker A. J., Wootten R., Shinn A. P., & Bron J. E. (2016). An assessment of the potential for zoonotic parasitic nematode infections arising from the consumption of maricultured Atlantic halibut, Hippoglossus hippoglossus (L.), and rainbow trout, Oncorhynchus mykiss (Walbaum), in Scotland. Food Control, 66, 198–204. 10.1016/j.foodcont.2016.02.011 [CrossRef] [Google Scholar]
- Brunet J., Pesson B., Royant M., Lemoine J. P., Pfaff A. W., Abou‐Bacar A., Year H., Fréalle E., Dupouy‐Camet J., Merino‐Espinosa G., Gómez‐Mateos M., Martin‐Sanchez J., & Candolfi E. (2017). Molecular diagnosis of Pseudoterranova decipiens s.s in human, France. BMC Infectious Diseases, 17, 397. 10.1186/s12879-017-2493-7 [Europe PMC free article] [Abstract] [CrossRef] [Google Scholar]
- Bušelić I., Trumbić Ž., Hrabar J., Vrbatović A., Bočina I., & Mladineo I. (2018). Molecular and cellular response to experimental Anisakis pegreffii (Nematoda, Anisakidae) third‐stage larval infection in rats. Frontiers in Immunology, 9, 2055. 10.3389/fimmu.2018.02055 [Europe PMC free article] [Abstract] [CrossRef] [Google Scholar]
- Bush A. O., Lafferty K. D., Lotz J. M., & Shostak A. W. (1997). Parasitology meets ecology on its own terms: Margolis et al. Revisited. Journal of Parasitology, 83, 575–583. [Abstract] [Google Scholar]
- Caffara M., Gustinelli A., Mazzone A., & Fioravanti M. L. (2020). Multiplex PCR for simultaneous identification of the most common European Opisthorchiid and Heterophyid in fish or fish products. Food and Waterborne Parasitology, 19, e00081. 10.1016/j.fawpar.2020.e00081 [Europe PMC free article] [Abstract] [CrossRef] [Google Scholar]
- Caffara M., Serracca L., Gustinelli A., Vencia W., Rossini I., Prearo M., & Fioravanti M. L. (2017). Development and validation of species‐specific molecular diagnostic tool for Opisthorchis felineus (Digenea, Opisthorchiidae) metacercariae. International Journal of Food Microbiology, 242, 98–100. 10.1016/j.ijfoodmicro.2016.11.024 [Abstract] [CrossRef] [Google Scholar]
- Caldeira A. J. R., Alves C. P. P., & Santos M. J. (2021). Anisakis notification in fish: An assessment of the cases reported in the European Union rapid alert system for food and feed (RASFF) database. Food Control, 124, 107913. 10.1016/j.foodcont.2021.107913 [CrossRef] [Google Scholar]
- Cammilleri G., Costa A., Graci S., Buscemi M. D., Collura R., Vella A., Pulvirenti A., Cicero A., Giangrosso G., Schembri P., & Ferrantelli V. (2018). Presence of Anisakis pegreffii in farmed sea bass (Dicentrarchus labrax L.) commercialized in southern Italy: A first report. Veterinary Parasitology, 259, 13–16. 10.1016/j.vetpar.2018.06.021 [Abstract] [CrossRef] [Google Scholar]
- Cammilleri G., Ferrantelli V., Pulvirenti A., Drago C., Stampone G., del Rocío Quintero Macías G., Drago S., Arcoleo G., Costa A., Geraci F., & Di Bella C. (2020). Validation of a commercial loop‐mediated isothermal amplification (LAMP) assay for the rapid detection of Anisakis spp. DNA in Processed Fish Products. Food, 9(1), 92. 10.3390/foods9010092 [Europe PMC free article] [Abstract] [CrossRef] [Google Scholar]
- Carballeda‐Sangiao N., Olivares F., Rodríguez ‐Mahillo A. I., Careche M., Tejada M., Moneo I., & González‐Muñoz M. (2014). Identification of autoclave‐resistant Anisakis simplex allergens. Journal of Food Protection, 77, 605–609. 10.4315/0362-028X.JFP-13-278 [Abstract] [CrossRef] [Google Scholar]
- Carmo J., Marques S., Bispo M., & Serra D. (2017). Anisakiasis: A growing cause of abdominal pain! BMJ Case Reports, 2017. 10.1136/bcr-2016-218857 [Europe PMC free article] [Abstract] [CrossRef] [Google Scholar]
- Carrera M., Gallardo J. M., Pascual S., González Á. F., & Medina I. (2016). Protein biomarker discovery and fast monitoring for the identification and detection of Anisakids by parallel reaction monitoring (PRM) mass spectrometry. Journal of Proteomics, 142, 130–137. 10.1016/j.jprot.2016.05.012 [Abstract] [CrossRef] [Google Scholar]
- Castiglione D., Guardone L., Susini F., Alimonti F., Paternoster V., Ricci E., Nucera D., & Armani A. (2021). A case study on farmed European seabass and gilthead seabream in central Italy: The negligible parasitological risk of nematode larvae paves the way for the freezing derogation. Food Control, 125, 107964. 10.1016/j.foodcont.2021.107964 [CrossRef] [Google Scholar]
- Cavallero S., Bellini I., Pizzarelli A., Arcà B., & D'Amelio S. (2022). A miRNAs catalogue from third‐stage larvae and extracellular vesicles of Anisakis pegreffii provides new clues for host‐parasite interplay. Scientific Reports, 12, 9667. 10.1038/s41598-022-13594-3 [Europe PMC free article] [Abstract] [CrossRef] [Google Scholar]
- Cavallero S., Bruno A., Arletti E., Caffara M., Fioravanti M. L., Costa A., Cammilleri G., Graci S., Ferrantelli V., & D'Amelio S. (2017). Validation of a commercial kit aimed to the detection of pathogenic anisakid nematodes in fish products. International Journal of Food Microbiology, 257, 75–79. 10.1016/j.ijfoodmicro.2017.06.011 [Abstract] [CrossRef] [Google Scholar]
- Cavallero S., Lombardo F., Salvemini M., Pizzarelli A., Cantacessi C., & D'Amelio S. (2020). Comparative transcriptomics reveals clues for differences in pathogenicity between Hysterothylacium aduncum, Anisakis simplex sensu stricto and Anisakis pegreffii . Genes, 11, 321. 10.3390/genes11030321 [Europe PMC free article] [Abstract] [CrossRef] [Google Scholar]
- Cavallero S., Lombardo F., Su X., Salvemini M., Cantacessi C., & D'Amelio S. (2018). Tissue‐specific transcriptomes of Anisakis simplex (sensu stricto) and Anisakis pegreffii reveal potential molecular mechanisms involved in pathogenicity. Parasites & Vectors, 11, 31. 10.1186/s13071-017-2585-7 [Europe PMC free article] [Abstract] [CrossRef] [Google Scholar]
- Cavallero S., Scribano D., & D'Amelio S. (2016). First case report of invasive pseudoterranoviasis in Italy. Parasitology International, 65, 488–490. 10.1016/j.parint.2016.07.003 [Abstract] [CrossRef] [Google Scholar]
- Cech G., Sándor D., Molnár K., Varga Á., Caffara M., Fioravanti M. L., Buchmann K., & Székely C. (2021). Digenean trematodes in Hungarian freshwater aquacultures. Food and Waterborne Parasitology, 22, e00101. 10.1016/j.fawpar.2020.e00101 [Europe PMC free article] [Abstract] [CrossRef] [Google Scholar]
- Chavan P., Sharma P., Sharma S. R., Mittal T. C., & Jaiswal A. K. (2022). Application of high‐intensity ultrasound to improve food processing efficiency: A review. Food, 11, 122. 10.3390/foods11010122 [Europe PMC free article] [Abstract] [CrossRef] [Google Scholar]
- Chen X., Zhao L., Wang J., Wang H., Qiu Y., Dong Z., Zhang C., Liu M., Wang X., & Bai X. (2022). Rapid visual detection of anisakid nematodes using recombinase polymerase amplification and SYBR green I. Frontiers in Microbiology, 13. 10.3389/fmicb.2022.1026129 [Europe PMC free article] [Abstract] [CrossRef] [Google Scholar]
- Cipriani P., Acerra V., Bellisario B., Sbaraglia G. L., Cheleschi R., Nascetti G., & Mattiucci S. (2016). Larval migration of the zoonotic parasite Anisakis pegreffii (Nematoda: Anisakidae) in European anchovy, Engraulis encrasicolus: Implications to seafood safety. Food Control, 59, 148–157. 10.1016/j.foodcont.2015.04.043 [CrossRef] [Google Scholar]
- Cipriani P., Giulietti L., Bao M., Palomba M., Mattiucci S., & Levsen A. (2024). Post‐mortem tissue migration of Anisakis simplex (s.s.) larvae (Nematoda: Anisakidae) in three commercially harvested fish species from the Northeast Atlantic: The role of storage time and temperature. Food Control, 157, 110162. 10.1016/j.foodcont.2023.110162 [CrossRef] [Google Scholar]
- Cipriani P., Giulietti L., Shayo S. D., Storesund J. E., Bao M. G., Palomba M., Mattiucci S., & Levsen A. (2022). Anisakid nematodes in Trichiurus lepturus and Saurida undosquamis (Teleostea) from the south‐West Indian Ocean: Genetic evidence for the existence of sister species within Anisakis typica (s.l.), and food‐safety considerations. Food and Waterborne Parasitology, 28, e00177. 10.1016/j.fawpar.2022.e00177 [Europe PMC free article] [Abstract] [CrossRef] [Google Scholar]
- Clodoveo M. L., Dipalmo T., Crupi P., Durante V., Pesce V., Maiellaro I., Lovece A., Mercurio A., Laghezza A., & Corbo F. (2016). Comparison between different flavored olive oil production techniques: Healthy value and process efficiency. Plant Foods for Human Nutrition, 71, 81–87. 10.1007/s11130-016-0528-7 [Abstract] [CrossRef] [Google Scholar]
- Crotta M., Ferrari N., & Guitian J. (2016). Qualitative risk assessment of introduction of anisakid larvae in Atlantic salmon (Salmo salar) farms and commercialization of products infected with viable nematodes. Food Control, 69, 275–284. 10.1016/j.foodcont.2016.04.058 [CrossRef] [Google Scholar]
- Cuesta F., Sánchez‐Alonso I., Navas A., & Careche M. (2021). Calculation of full process freezing time in minced fish muscle. Methods, 8, 101292. 10.1016/j.mex.2021.101292 [Europe PMC free article] [Abstract] [CrossRef] [Google Scholar]
- D'Amelio S., Bellini I., Chiovoloni C., Magliocco C., Pronio A., Di Rocco A., Pentassuglio I., Rosati M., Russo G., & Cavallero S. (2023). A case of gastroallergic and intestinal anisakiasis in Italy: Diagnosis based on double endoscopy and molecular identification. Pathogens, 12(9), 1172. 10.3390/pathogens12091172 [Europe PMC free article] [Abstract] [CrossRef] [Google Scholar]
- Dantas‐Torres F. (2023). Artificial intelligence, parasites and parasitic diseases. Parasites & Vectors, 16(1), 340. 10.1186/s13071-023-05972-1 [Europe PMC free article] [Abstract] [CrossRef] [Google Scholar]
- Daschner A., Levsen A., Cipriani P., & Cuéllar Del Hoyo C. (2021). Anisakis allergy: Unjustified social alarm versus healthy diet. Parasitology Research, 120, 769–771. 10.1007/s00436-020-07029-z [Abstract] [CrossRef] [Google Scholar]
- Datta A. K., & Davidson P. M. (2000). Microwave and radio frequency processing. Journal of Food Science, 65, 32–41. 10.1111/j.1750-3841.2000.tb00616.x [CrossRef] [Google Scholar]
- Della Morte D., Ambrosi C., Chiereghin F., Infante M., Pastore D., Pacifici F., Scaramella M., Gentile L., Mulas F., & Quintavalle G. (2023). Methods for inactivation of seafood Anisakis larvae and prevention of human anisakiasis: A mini‐review. European Review for Medical and Pharmacological Sciences, 27, 5246–5256. 10.26355/eurrev_202306_32643 [Abstract] [CrossRef] [Google Scholar]
- Diez G., Chust G., Andonegi E., Santurtún M., Abaroa C., Bilbao E., Maceira A., & Mendibil I. (2022). Analysis of potential drivers of spatial and temporal changes in anisakid larvae infection levels in European hake, Merluccius merluccius (L.), from the north‐East Atlantic fishing grounds. Parasitology Research, 121(7), 1903–1920. 10.1007/s00436-022-07446-2 [Abstract] [CrossRef] [Google Scholar]
- Dinas S., Diakou A., Vasiliadis A., Chaintoutis S. C., Massa E., Konstantinou G. N., Totsi A., Xakis A., & Papavasiliou C. (2024). First case of human anisakiosis in Greece: Acute invasive infection mimicking peritoneal malignancy. Pathogens, 13, 149. 10.3390/pathogens13020149 [Europe PMC free article] [Abstract] [CrossRef] [Google Scholar]
- Duan Y., Al‐Jubury A., Kania P. W., & Buchmann K. (2021). Trematode diversity reflecting the community structure of Danish freshwater systems: Molecular clues. Parasites & Vectors, 14, 43. 10.1186/s13071-020-04536-x [Europe PMC free article] [Abstract] [CrossRef] [Google Scholar]
- Duflot M., Cresson P., Julien M., Chartier L., Bourgau O., Palomba M., Mattiucci S., Midelet G., & Gay M. (2023). Black spot diseases in seven commercial fish species from the English channel and the North Sea: Infestation levels, identification and population genetics of Cryptocotyle spp. Parasite, 30, 28. 10.1051/parasite/2023028 [Europe PMC free article] [Abstract] [CrossRef] [Google Scholar]
- Duflot M., Midelet G., Bourgau O., Buchmann K., & Gay M. (2021). Optimization of tools for the detection and identification of Cryptocotyle metacercariae in fish: Digestion method and viability studies. Journal of Fish Diseases, 44, 1777–1784. 10.1111/jfd.13495 [Europe PMC free article] [Abstract] [CrossRef] [Google Scholar]
- ECA (European Court of Auditors) . (2023). EU aquaculture policy–Stagnating production and unclear results despite increased EU funding (Special report No 25/2023). https://www.eca.europa.eu/en/publications?ref=SR‐2023‐25
- EC DG‐MARE (European Commission, Directorate‐General for Maritime Affairs and Fisheries) . (2023). Th EU Fish Market 2023 edition. Publications Office of the European Union . https://data.europa.eu/doi/10.2771/38507
- EFSA and ECDC (European Food Safety Authority and European Centre for Disease Prevention and Control) . (2023). The European Union one health 2022 zoonoses report. EFSA Journal, 21(12), e8442. 10.2903/j.efsa.2023.8442 [Europe PMC free article] [Abstract] [CrossRef] [Google Scholar]
- EFSA BIOHAZ Panel (Panel on Biological Hazards) . (2010). Scientific opinion on risk assessment of parasites in fishery products. EFSA Journal, 8(4), 1543. 10.2903/j.efsa.2010.1543 [CrossRef] [Google Scholar]
- EFSA BIOHAZ Panel (Panel on Biological Hazards) . (2015). Scientific opinion on the evaluation of heat treatments, different from those currently established in the EU legislation, that could be applied to live bivalve molluscs from B and C production areas, that have not been submitted to purification or relaying, in order to eliminate pathogenic microorganisms. EFSA Journal, 13(12), 4332. 10.2903/j.efsa.2015.4332 [CrossRef] [Google Scholar]
- EFSA BIOHAZ Panel (Panel on Biological Hazards) , Koutsoumanis K., Alvarez‐Ordóñez A., Bolton D., Bover‐Cid S., Chemaly M., Davies R., De Cesare A., Herman L., Hilbert F., Lindqvist R., Nauta M., Peixe L., Ru G., Simmons M., Skandamis P., Suffredini E., Castle L., Crotta M., … Allende A. (2022). The efficacy and safety of high‐pressure processing of food. EFSA Journal, 20(3), 7128. 10.2903/j.efsa.2022.7128 [Europe PMC free article] [Abstract] [CrossRef] [Google Scholar]
- EFSA Scientific Committee , Benford D., Halldorsson T., Jeger M. J., Knutsen H. K., More S., Naegeli H., Noteborn H., Ockleford C., Ricci A., Rychen G., Schlatter J. R., Silano V., Solecki R., Turck D., Younes M., Craig P., Hart A., Von Goetz N., … Hardy A. (2018a). Guidance on uncertainty analysis in scientific assessments. EFSA Journal, 16(1), 5123. 10.2903/j.efsa.2018.5123 [Europe PMC free article] [Abstract] [CrossRef] [Google Scholar]
- EFSA Scientific Committee , Benford D., Halldorsson T., Jeger M. J., Knutsen H. K., More S., Naegeli H., Noteborn H., Ockleford C., Ricci A., Rychen G., Schlatter J. R., Silano V., Solecki R., Turck D., Younes M., Craig P., Hart A., Von Goetz N., … Hardy A. (2018b). The principles and methods behind EFSA's guidance on uncertainty analysis in scientific assessment. EFSA Journal, 16(1), 5122. 10.2903/j.efsa.2018.5122 [Europe PMC free article] [Abstract] [CrossRef] [Google Scholar]
- EFSA Scientific Committee , More S., Bampidis V., Benford D., Bragard C., Hernández ‐Jerez A. F., Bennekou S. H., Koutsoumanis K., Lambre C., Machera K., Mullins E., Nielsen S. S., Schrenk D., Turck D., Younes M., Kraft A., Naegeli H., Tsaioun K., Aiassa E., … Halldorsson T. I. (2023). Guidance on protocol development for EFSA generic scientific assessments. EFSA Journal, 21(10), 8312. 10.2903/j.efsa.2023.8312 [Europe PMC free article] [Abstract] [CrossRef] [Google Scholar]
- Erkinharju T., Dalmo R. A., Hansen M., & Seternes T. (2021). Cleaner fish in aquaculture: Review on diseases and vaccination. Reviews in Aquaculture, 13, 189–237. 10.1111/raq.12470 [CrossRef] [Google Scholar]
- Esteban J. G., Muñoz‐Antoli C., Borras M., Colomina J., & Toledo R. (2014). Human infection by a "fish tapeworm", Diphyllobothrium latum, in a non‐endemic country. Infection, 42, 191–194. 10.1007/s15010-013-0491-2 [Abstract] [CrossRef] [Google Scholar]
- Evans P. G., & Bjørge A. (2013). Impacts of climate change on marine mammals. MCCIP Science Review, 2013, 134–148. 10.14465/2013.arc15.134-148 [CrossRef] [Google Scholar]
- Fæste C. K., Jonscher K. R., Dooper M. M., Egge‐Jacobsen W., Moen A., Daschner A., Egaas E., & Christians U. (2014). Characterisation of potential novel allergens in the fish parasite Anisakis simplex . EuPA Open Proteomics, 4, 140–155. 10.1016/j.euprot.2014.06.006 [Europe PMC free article] [Abstract] [CrossRef] [Google Scholar]
- Fæste C. K., Levsen A., Lin A. H., Larsen N., Plassen C., Moen A., Do T. V., & Egaas E. (2015). Fish feed as source of potentially allergenic peptides from the fish parasite Anisakis simplex (s.l.). Animal Feed Science and Technology, 202, 52–61. 10.1016/j.anifeedsci.2015.01.006 [CrossRef] [Google Scholar]
- Fæste C. K., Moen A., Schniedewind B., Anonsen J. H., Klawitter J., & Christians U. (2016). Development of liquid chromatography‐tandem mass spectrometry methods for the quantitation of Anisakis simplex proteins in fish. The Journal of Chromatography A, 1432, 58–72. 10.1016/j.chroma.2016.01.002 [Abstract] [CrossRef] [Google Scholar]
- Fæste C. K., Plassen C., Løvberg K. E., Moen A., & Egaas E. (2015). Detection of proteins from the fish parasite Anisakis simplex in Norwegian farmed salmon and processed fish products. Food Analytical Methods, 8, 1390–1402. 10.1007/s12161-014-0003-8 [CrossRef] [Google Scholar]
- FAO . (2022). The state of world fisheries and aquaculture 2022. Rome, FAO. 10.4060/cc0461en [CrossRef] [Google Scholar]
- FDA (U.S. Food and Drug Administration) . (2023). Food code 2022–recommendations of the United States Public Health Service Food and Drug Administration [Press release]. Chapter 3–22, 87 pp. https://www.fda.gov/food/fda‐food‐code/food‐code‐2022
- Fernandez‐Jover D., Faliex E., Sánchez‐Jerez P., Sasal P., & Bayle‐Sempere J. T. (2010). Coastal fish farming does not affect the total parasite communities of wild fish in SW Mediterranean. Aquaculture, 300, 10–16. 10.1016/j.aquaculture.2009.12.006 [CrossRef] [Google Scholar]
- Fioravanti M. L., Gustinelli A., Rigos G., Buchmann K., Caffara M., Pascual S., & Pardo M. A. (2021). Negligible risk of zoonotic anisakid nematodes in farmed fish from European mariculture, 2016 to 2018. Eurosurveillance, 26. 10.2807/1560-7917.es.2021.26.2.1900717 [Europe PMC free article] [Abstract] [CrossRef] [Google Scholar]
- Fiorenza E. A., Wendt C. A., Dobkowski K. A., King T. L., Pappaionou M., Rabinowitz P., Samhouri J. F., & Wood C. L. (2020). It's a wormy world: Meta‐analysis reveals several decades of change in the global abundance of the parasitic nematodes Anisakis spp. and Pseudoterranova spp. in marine fishes and invertebrates. Global Change Biology, 26, 2854–2866. 10.1111/gcb.15048 [Abstract] [CrossRef] [Google Scholar]
- Fiskeridirektoratet . (2022). Key Figures from Norwegian Aquaculture Industry 2021 . https://www.fiskeridir.no/English/Aquaculture/Statistics/Booklets
- Franssen F., Gerard C., Cozma‐Petrut A., Vieira‐Pinto M., Jambrak A. R., Rowan N., Paulsen P., Rozycki M., Tysnes K., Rodríguez‐Lázaro D., & Robertson L. (2019). Inactivation of parasite transmission stages: Efficacy of treatments on food of animal origin. Trends in Food Science & Technology, 83, 114–128. 10.1016/j.tifs.2018.11.009 [CrossRef] [Google Scholar]
- Fu J., He Y., & Cheng F. (2023). Intelligent cutting in fish processing: Efficient, high‐quality, and safe production of fish products. Food and Bioprocess Technology, 1‐22, 828–849. 10.1007/s11947-023-03163-5 [CrossRef] [Google Scholar]
- García Pérez J. C., Rodríguez‐ Pérez R., Ballestero A., Zuloaga J., Fernández‐Puntero B., Arias‐Díaz J., & Caballero M. L. (2015). Previous exposure to the fish parasite Anisakis as a potential risk factor for gastric or colon adenocarcinoma. Medicine (Baltimore), 94, e1699. 10.1097/md.0000000000001699 [Europe PMC free article] [Abstract] [CrossRef] [Google Scholar]
- Gay M., Bao M., MacKenzie K., Pascual S., Buchmann K., Bourgau O., Couvreur C., Mattiucci S., Paoletti M., Hastie L. C., Levsen A., & Pierce G. J. (2018). Infection levels and species diversity of ascaridoid nematodes in Atlantic cod, Gadus morhua, are correlated with geographic area and fish size. Fisheries Research, 202, 90–102. 10.1016/j.fishres.2017.06.006 [CrossRef] [Google Scholar]
- Giarratana F., Giuffrida A., Gallo F., Ziino G., & Panebianco A. (2012). Study of the resistance variability of Anisakis larvae to some technological stressors. In A. Pugliese , editor; , A. Gaiti , editor; , & C. Boiti , editor. (Eds.), Veterinary science: Current aspects in biology, animal pathology, clinic and food hygiene (pp. 155–159). Springer. 10.1007/978-3-642-23271-8_26 [CrossRef] [Google Scholar]
- Giarratana F., Muscolino D., Beninati C., Giuffrida A., & Panebianco A. (2014). Activity of Thymus vulgaris essential oil against Anisakis larvae. Experimental Parasitology, 142, 7–10. 10.1016/j.exppara.2014.03.028 [Abstract] [CrossRef] [Google Scholar]
- Giarratana F., Muscolino D., Panebianco F., Patania A., Benianti C., Ziino G., & Giuffrida A. (2015). Activity of R(+) limonene against Anisakis larvae. Italian Journal of Food Safety, 4, 5499. 10.4081/ijfs.2015.5499 [Europe PMC free article] [Abstract] [CrossRef] [Google Scholar]
- Giarratana F., Muscolino D., Ziino G., Giuffrida A., Marotta S. M., Lo Presti V., Chiofalo V., & Panebianco A. (2017). Activity of Tagetes minuta Linnaeus (Asteraceae) essential oil against L3 Anisakis larvae type 1. Asian Pacific Journal of Tropical Medicine, 10, 461–465. 10.1016/j.apjtm.2017.05.005 [Abstract] [CrossRef] [Google Scholar]
- Giarratana F., Muscolino D., Ziino G., Lo Presti V., Rao R., Chiofalo V., Giuffrida A., & Panebianco A. (2017). Activity of catmint (Nepeta cataria) essential oil against Anisakis larvae. Tropical Biomedicine, 34, 22–31. [Abstract] [Google Scholar]
- Giarratana F., Panebianco F., Muscolino D., Beninati C., Ziino G., & Giuffrida A. (2015). Effect of allyl isothiocyanate against Anisakis larvae during the anchovy marinating process. Journal of Food Protection, 78, 767–771. 10.4315/0362-028x.Jfp-14-441 [Abstract] [CrossRef] [Google Scholar]
- Giulietti L., Karlsbakk E., Cipriani P., Bao M., Storesund J. E., Marathe N. P., & Levsen A. (2022). Long‐term investigation of the ‘soft flesh’ condition in Northeast Atlantic mackerel induced by the myxosporean parasite Kudoa thyrsites (Cnidaria, Myxozoa): Temporal trends and new molecular epidemiological observations. Fisheries Research, 248, 106221. 10.1016/j.fishres.2021.106221 [CrossRef] [Google Scholar]
- Godinez‐Gonzales C., Roca‐Gerones X., Cancino‐Faure B., Montoliu I., & Fisa R. (2017). Quantitative SYBR green qPCR technique for the detection of the nematode parasite Anisakis in commercial fish‐derived food. International Journal of Food Microbiology, 261, 89–94. [Abstract] [Google Scholar]
- Goffredo E., Azzarito L., Di Taranto P., Mancini M. E., Normanno G., Didonna A., Faleo S., Occhiochiuso G., D'Attoli L., Pedarra C., Pinto P., Cammilleri G., Graci S., Sciortino S., & Costa A. (2019). Prevalence of anisakid parasites in fish collected from Apulia region (Italy) and quantification of nematode larvae in flesh. International Journal of Food Microbiology, 292, 159–170. 10.1016/j.ijfoodmicro.2018.12.025 [Abstract] [CrossRef] [Google Scholar]
- Goldberg T., & Owens L. (2024). Universal method for parasite and eukaryotic endosymbiont identification. P220194wo01. https://www.warf.org/technologies/summary/P220194WO01
- Gomes T. L., Quiazon K. M., Itoh N., Fujise Y., & Yoshinaga T. (2023). Effects of temperature on eggs and larvae of Anisakis simplex sensu stricto and Anisakis pegreffii (Nematoda: Anisakidae) and its possible role on their geographic distributions. Parasitology International, 92, 102684. 10.1016/j.parint.2022.102684 [Abstract] [CrossRef] [Google Scholar]
- Gómez‐Mateos Pérez M., Navarro Moll C., Merino Espinosa G., & Valero López A. (2017). Evaluation of different Mediterranean essential oils as prophylactic agents in anisakidosis. Pharmaceutical Biology, 55, 456–461. 10.1080/13880209.2016.1247880 [Europe PMC free article] [Abstract] [CrossRef] [Google Scholar]
- Gómez‐Morales M. A., Castro C. M., Lalle M., Fernández R., Pezzotti P., Abollo E., & Pozio E. (2018). UV‐press method versus artificial digestion method to detect Anisakidae L3 in fish fillets: Comparative study and suitability for the industry. Fisheries Research, 202, 22–28. 10.1016/j.fishres.2016.12.011 [CrossRef] [Google Scholar]
- Gòmez‐Morales M. A., Ludovisi A., Giuffra E., Manfredi M. T., Piccolo G., & Pozio E. (2008). Allergenic activity of Molicola horridus (Cestoda, Trypanorhyncha), a cosmopolitan fish parasite, in a mouse model. Veterinary Parasitology, 157, 314–320. 10.1016/j.vetpar.2008.07.010 [Abstract] [CrossRef] [Google Scholar]
- Gómez‐Rincón C., Langa E., Murillo P., Valero M. S., Berzosa C., & López V. (2014). Activity of tea tree (Melaleuca alternifolia) essential oil against L3 larvae of Anisakis simplex . Biomed Research International, 2014, 549510. 10.1155/2014/549510 [Europe PMC free article] [Abstract] [CrossRef] [Google Scholar]
- Goncharov S., & Soroka N. (2016). Experimental infection of laboratory rats with metacercaria of trematodes Paracoenogonimus ovatus (Trematoda, Cyathocotylidae) . The Animal Biology, 18(1), 17–21. 10.15407/animbiol18.01.017 [CrossRef] [Google Scholar]
- Guan A., Usieto M., Cobacho Arcos S., Sánchez‐Alonso I., Otero L., & Careche M. (2023). High throughput screening method for Anisakis L3 detection of locomotor activity and viability under thermal‐induced stress. Proceedings of the 51st Conference of the West European Fish Technologists’ Association, Copenhagen, Denmark, 16th‐19th October 2023s.
- Guan A., Usieto M., Otero L., Navas A., Cobacho Arcos S., Sánchez‐Alonso I., & Careche M. (2022). Study of the progressive changes in Anisakis third stage larvae upon heating until their inactivation. Proceedings of the 50th Conference of the West European Fish Technologists’ Association, Rotterdam, The Netherlands 17–21 de October 2022s.
- Guan A., Usieto M., Sánchez‐Alonso I., Arcos S. C., Careche M., & Otero L. (2023). Modeling survival curves of Anisakis L3 after isothermal heat treatments at lethal temperatures. Food Control, 154, 109975. 10.1016/j.foodcont.2023.109975 [CrossRef] [Google Scholar]
- Guan A., Van Damme I., Devlieghere F., & Gabriël S. (2021). Effect of temperature, CO2 and O2 on motility and mobility of Anisakidae larvae. Scientific Reports, 11, 4279. 10.1038/s41598-021-83505-5 [Europe PMC free article] [Abstract] [CrossRef] [Google Scholar]
- Guardone L., Malandra R., Costanzo F., Castigliego L., Tinacci L., Gianfaldoni D., Guidi A., & Armani A. (2016). Assessment of a sampling plan based on visual inspection for the detection of anisakid larvae in fresh anchovies (Engraulis encrasicolus). A first step towards official validation? Food Analytical Methods, 9, 1418–1427. 10.1007/s12161-015-0316-2 [CrossRef] [Google Scholar]
- Guardone L., Nucera D., Lodola L. B., Tinacci L., Acutis P. L., Guidi A., & Armani A. (2018). Anisakis spp. larvae in different kinds of ready to eat products made of anchovies (Engraulis encrasicolus) sold in Italian supermarkets. International Journal of Food Microbiology, 268, 10–18. 10.1016/j.ijfoodmicro.2017.12.030 [Abstract] [CrossRef] [Google Scholar]
- Gustinelli A., Menconi V., Prearo M., Caffara M., Righetti M., Scanzio T., Raglio A., & Fioravanti M. L. (2016). Prevalence of Diphyllobothrium latum (Cestoda: Diphyllobothriidae) plerocercoids in fish species from four Italian lakes and risk for the consumers. International Journal of Food Microbiology, 235, 109–112. 10.1016/j.ijfoodmicro.2016.06.033 [Abstract] [CrossRef] [Google Scholar]
- Heia K., Washburn K. E., & Skjelvareid M. H. (2017). Automatic quality control of internal defects in cod–results from hyperspectral, ultrasound and X‐ray imaging (research report 28/2017). Nofima AS. http://hdl.handle.net/11250/2480578
- Herath H. M. P. D., Taki A. C., Rostami A., Jabbar A., Keiser J., Geary T. G., & Gasser R. B. (2022). Whole‐organism phenotypic screening methods used in early‐phase anthelmintic drug discovery. Biotechnology Advances, 57, 107937. 10.1016/j.biotechadv.2022.107937 [Abstract] [CrossRef] [Google Scholar]
- Herrero B., Vieites J. M., & Espiñeira M. (2011). Detection of anisakids in fish and seafood products by real‐time PCR. Food Control, 22, 933–939. 10.1016/j.foodcont.2010.11.028 [CrossRef] [Google Scholar]
- Heuch P. A., Jansen P. A., Hansen H., Sterud E., MacKenzie K., Haugen P., & Hemmingsen W. (2011). Parasite faunas of farmed cod and adjacent wild cod populations in Norway: A comparison. Aquaculture Environment Interactions, 2, 1–13. 10.3354/aei00027 [CrossRef] [Google Scholar]
- Højgaard D. P. (1998). Impact of temperature, salinity and light on hatching of eggs of Anisakis simplex (Nematoda, Anisakidae), isolated by a new method, and some remarks on survival of larvae. Sarsia, 83, 21–28. 10.1080/00364827.1998.10413666 [CrossRef] [Google Scholar]
- Huss H. H. (1994). Assurance of seafood quality. Food & Agriculture Organization Fisheries Technical Paper, FAO No. 334. https://www.fao.org/3/t1768e/t1768e00.htm
- Ioannou Kapota A. (2012). Health status of sea bass (Dicentrarchus labrax) and sea bream (Sparus aurata) farmed in Greece and Italy in relation to the presence of pathogenic zoonoses and Ectoparasites, [dissertation thesis]. Alma Mater Studiorum University of Bologna. https://amsdottorato.unibo.it/4817/ [Google Scholar]
- ISO (International Organization for Standardization) . (2012a). Microbiology of the food chain Methods for the detection of Anisakidae L3 larvae in fish and fishery products ‐ Part 2: Artificial digestion method (ISO Standard No. 23036‐2:2021). https://www.iso.org/standard/74373.html
- ISO (International Organization for Standardization) . (2012b). Microbiology of the food chain methods for the detection of Anisakidae L3 larvae in fish and fishery products ‐ Part 1: UV‐press method (ISO Standard No. 23036‐1:2021). https://www.iso.org/standard/74372.html
- Jaruboonyakorn P., Tejangkura T., & Chontananarth T. (2022). Multiplex PCR development for the simultaneous and rapid detection of two pathogenic flukes, Dactylogyrus spp. and Centrocestus formosanus, in ornamental fishes. Aquaculture, 548, 737660. 10.1016/j.aquaculture.2021.737660 [CrossRef] [Google Scholar]
- Kang G., Choi K. M., Cho D. H., Joo M. S., Heo M. J., Woo W. S., & Park C. I. (2020). The first detection of Kudoa hexapunctata in farmed pacific bluefin tuna in South Korea, Thunnus orientalis (Temminck and Schlegel, 1844). Animals (Basel), 10. 10.3390/ani10091705 [Europe PMC free article] [Abstract] [CrossRef] [Google Scholar]
- Karami A. M., Marnis H., Korbut R., Zuo S. Z., Jaafar R., Duan Y. J., Mathiessen H., Al‐Jubury A., Kania P. W., & Buchmann K. (2022). Absence of zoonotic parasites in salmonid aquaculture in Denmark: Causes and consequences. Aquaculture, 549, 737793. 10.1016/j.aquaculture.2021.737793 [CrossRef] [Google Scholar]
- Kawai T., Sekizuka T., Yahata Y., Kuroda M., Kumeda Y., Iijima Y., Kamata Y., Sugita‐Konishi Y., & Ohnishi T. (2012). Identification of Kudoa septempunctata as the causative agent of novel food poisoning outbreaks in Japan by consumption of Paralichthys olivaceus in raw fish. Clinical Infectious Diseases, 54, 1046–1052. 10.1093/cid/cir1040 [Abstract] [CrossRef] [Google Scholar]
- Klapper R., Karl H., & Ostermeyer U. (2021). Intrinsic properties of anisakid nematode larvae as a potential tool for the detection in fish products. International Journal of Food Microbiology, 343, 109094. 10.1016/j.ijfoodmicro.2021.109094 [Abstract] [CrossRef] [Google Scholar]
- Kochanowski M., Różycki M., Dąbrowska J., Karamon J., Sroka J., Antolak E., Bełcik A., & Cencek T. (2020). Development and application of novel chemiluminescence immunoassays for highly sensitive detection of Anisakis simplex proteins in thermally processed seafood. Pathogens, 9, 777. 10.3390/pathogens9100777 [Europe PMC free article] [Abstract] [CrossRef] [Google Scholar]
- Kołodziejczyk L., Szostakowska B., Sobecka E., Szczucki K., & Stankiewicz K. (2020). First case of human anisakiasis in Poland. Parasitology International, 76, 102073. 10.1016/j.parint.2020.102073 [Abstract] [CrossRef] [Google Scholar]
- Kondrashin A. V., Morozova L. F., Stepanova E. V., Turbabina N. A., Maksimova M. S., Anikina A. S., Shahin‐jafari A., Morozov A. E., Mikhaylov D. V., Kupriyanova Y. D., & Morozov E. N. (2023). A rare human helminth infection in Russia. Tropical Medicine and Infectious Disease, 8(8), 403. 10.3390/tropicalmed8080403 [Europe PMC free article] [Abstract] [CrossRef] [Google Scholar]
- Králová‐Hromadová I., Radačovská A., Čisovská Bazsalovicsová E., & Kuchta R. (2021). Ups and downs of infections with the broad fish tapeworm Dibothriocephalus latus in Europe from 1900 to 2020: Part I . Advances in Parasitology, 114, 75–166. 10.1016/bs.apar.2021.08.008 [Abstract] [CrossRef] [Google Scholar]
- Kristmundsson A., & Freeman M. A. (2014). Negative effects of Kudoa islandica n. sp. (Myxosporea: Kudoidae) on aquaculture and wild fisheries in Iceland. International Journal for Parasitology: Parasites and Wildlife, 3, 135–146. 10.1016/j.ijppaw.2014.06.001 [Europe PMC free article] [Abstract] [CrossRef] [Google Scholar]
- Kroeger M., Karl H., Simmler B., & Singer P. (2018). Viability test device for anisakid nematodes. Heliyon, 4, e00552. 10.1016/j.heliyon.2018.e00552 [Europe PMC free article] [Abstract] [CrossRef] [Google Scholar]
- Kuchta R., Radačovská A., Čisovská Bazsalovicsová E., Viozzi G., Semenas L., Arbetman M., & Scholz T. (2019). Host switching of zoonotic broad fish tapeworm (Dibothriocephalus latus) to salmonids, Patagonia. Emerging Infectious Diseases, 25, 2156–2158. 10.3201/eid2511.190792 [Europe PMC free article] [Abstract] [CrossRef] [Google Scholar]
- Kuchta R., Scholz T., Brabec J., & Narduzzi‐Wicht B. (2015). Chapter 17. Diphyllobothrium, Diplogonoporus and Spirometra . In L. Xiao , editor; , U. Ryan , editor; , & Y. Feng , editor. (Eds.), Biology of foodborne parasites: Section III important foodborne helminths (1st ed.). CRC Press, Food microbiology series (pp. 299–326). 10.1201/b18317 [CrossRef] [Google Scholar]
- Lanfranchi A. L., & Sardella N. H. (2010). Anisakids survival after microwaving, freezing and salting fish from Argentina. Food Science and Technology Research, 16, 499–504. 10.3136/fstr.16.499 [CrossRef] [Google Scholar]
- Lee A. K., Kim S. M., & Choi K. Y. (1985). A case of human infection with the larva of Terranova type a. Journal of Pathology and Translational Medicine, 19, 463–467. [Google Scholar]
- Lee K. H., Park S. Y., & Ha S. D. (2016). Inactivation of Anisakis simplex L3 in the flesh of white spotted conger (Conger myriaster) by high hydrostatic pressure and its effect on quality. Food Additives and Contaminants Part A, 33, 1010–1015. 10.1080/19440049.2016.1183108 [Abstract] [CrossRef] [Google Scholar]
- Levsen A., Cipriani P., Palomba M., Giulietti L., Storesund J. E., & Bao M. (2022). Anisakid parasites (Nematoda: Anisakidae) in three commercially important gadid fish species from the southern Barents Sea, with emphasis on key infection drivers and spatial distribution within the hosts. Parasitology, 149, 1942–1957. 10.1017/s0031182022001305 [Europe PMC free article] [Abstract] [CrossRef] [Google Scholar]
- Levsen A., & Maage A. (2016). Absence of parasitic nematodes in farmed, harvest quality Atlantic salmon (Salmo salar) in Norway–results from a large‐scale survey. Food Control, 68, 25–29. 10.1016/j.foodcont.2016.03.020 [CrossRef] [Google Scholar]
- Levsen A., Svanevik C. S., Cipriani P., Mattiucci S., Gay M., Hastie L. C., Bušelić I., Mladineo I., Karl H., Ostermeyer U., Buchmann K., Højgaard D., González A. F., Pascual S., & Pierce G. J. (2018). A survey of zoonotic nematodes of commercial key fish species from major European fishing grounds – Introducing the FP7 PARASITE exposure assessment study. Fisheries Research, 202, 4–21. 10.1016/j.fishres.2017.09.009 [CrossRef] [Google Scholar]
- Li J., Lian Z., Wu Z., Zeng L., Mu L., Yuan Y., Bai H., Guo Z., Mai K., Tu X., & Ye J. (2023). Artificial intelligence–based method for the rapid detection of fish parasites (Ichthyophthirius multifiliis, Gyrodactylus kobayashii, and Argulus japonicus). Aquaculture, 563, 738790. 10.1016/j.aquaculture.2022.738790 [CrossRef] [Google Scholar]
- Lim H., Jung B. K., Cho J., Yooyen T., Shin E. H., & Chai J. Y. (2015). Molecular diagnosis of cause of anisakiasis in humans, South Korea. Emerging Infectious Diseases, 21, 342–344. 10.3201/eid2102.140798 [Europe PMC free article] [Abstract] [CrossRef] [Google Scholar]
- Lin R. J., Chen C. Y., Lee J. D., Lu C. M., Chung L. Y., & Yen C. M. (2010). Larvicidal constituents of Zingiber officinale (ginger) against Anisakis simplex . Planta Medica, 76, 1852–1858. 10.1055/s-0030-1249971 [Abstract] [CrossRef] [Google Scholar]
- Liu W., Lyu J., Wu D., Cao Y., Ma Q., Lu Y., & Zhang X. (2022). Cutting techniques in the fish industry: A critical review. Food, 11(20), 3206. 10.3390/foods11203206 [Europe PMC free article] [Abstract] [CrossRef] [Google Scholar]
- Llarena‐Reino M., Piñeiro C., Antonio J., Outeriño L., Vello C., González Á. F., & Pascual S. (2013). Optimization of the pepsin digestion method for anisakids inspection in the fishing industry. Veterinary Parasitology, 191, 276–283. 10.1016/j.vetpar.2012.09.015 [Abstract] [CrossRef] [Google Scholar]
- Llorens C., Arcos S. C., Robertson L., Ramos R., Futami R., Soriano B., Ciordia S., Careche M., González‐ Muñoz M., Jiménez ‐Ruiz Y., Carballeda‐Sangiao N., Moneo I., Albar J. P., Blaxter M., & Navas A. (2018). Functional insights into the infective larval stage of Anisakis simplex s.s., Anisakis pegreffii and their hybrids based on gene expression patterns. BMC Genomics, 19, 592. 10.1186/s12864-018-4970-9 [Europe PMC free article] [Abstract] [CrossRef] [Google Scholar]
- Lopez I., & Pardo M. A. (2010). Evaluation of a real‐time polymerase chain reaction (PCR) assay for detection of Anisakis simplex parasite as a food‐borne allergen source in seafood products. Journal of Agricultural and Food Chemistry, 58, 1469–1477. 10.1021/jf903492f [Abstract] [CrossRef] [Google Scholar]
- López V., Cascella M., Benelli G., Maggi F., & Gómez‐Rincón C. (2018). Green drugs in the fight against Anisakis simplex‐larvicidal activity and acetylcholinesterase inhibition of Origanum compactum essential oil. Parasitology Research, 117, 861–867. 10.1007/s00436-018-5764-3 [Europe PMC free article] [Abstract] [CrossRef] [Google Scholar]
- López V., Gerique J., Langa E., Berzosa C., Valero M. S., & Gómez‐Rincón C. (2015). Anthelmintic effects of nutmeg (Myristica fragans) on Anisakis simplex L3 larvae obtained from Micromesistius potassou . Research in Veterinary Science, 100, 148–152. 10.1016/j.rvsc.2015.03.033 [Abstract] [CrossRef] [Google Scholar]
- López V., Pavela R., Gómez‐Rincón C., Les F., Bartolucci F., Galiffa V., Petrelli R., Cappellacci L., Maggi F., Canale A., Otranto D., Sut S., Dall'Acqua S., & Benelli G. (2019). Efficacy of Origanum syriacum essential oil against the mosquito vector Culex quinquefasciatus and the gastrointestinal parasite Anisakis simplex, with insights on acetylcholinesterase inhibition. Molecules, 24. 10.3390/molecules24142563 [Europe PMC free article] [Abstract] [CrossRef] [Google Scholar]
- Łopieńska‐Biernat E., Stryiński R., Polak I., Pawlikowski B., Pawlak J., & Podolska M. (2020). Effect of freezing on the metabolic status of L3 larvae of Anisakis simplex s. s. Infection, Genetics and Evolution, 82, 104312. 10.1016/j.meegid.2020.104312 [Abstract] [CrossRef] [Google Scholar]
- Martínez de Velasco G., Rodero M., Chivato T., & Cuéllar C. (2007). Seroprevalence of anti‐Kudoa sp. (Myxosporea: Multivalvulida) antibodies in a Spanish population. Parasitology Research, 100, 1205–1211. 10.1007/s00436-006-0390-x [Abstract] [CrossRef] [Google Scholar]
- Martínez J. M., Abad V., Quílez J., Raso J., Cebrián G., & Álvarez‐Lanzarote I. (2023). Pulsed electric fields (PEF) applications in the inactivation of parasites in food. Trends in Food Science & Technology, 138, 470–479. 10.1016/j.tifs.2023.06.030 [CrossRef] [Google Scholar]
- Marzano V., Pane S., Foglietta G., Levi Mortera S., Vernocchi P., Onetti Muda A., & Putignani L. (2020). Mass spectrometry based‐proteomic analysis of Anisakis spp.: A preliminary study towards a new diagnostic tool. Genes (Basel), 11. 10.3390/genes11060693 [Europe PMC free article] [Abstract] [CrossRef] [Google Scholar]
- Mata C., Stöck M., & Knopf K. (2023). Combining morphological and molecular characteristics for the identification of muscle metacercariae in tench (Tinca tinca). Proceedings of the 38th Ichthyoparasitological symposium Oer‐Erkenschwick, Germany, 11 pp.
- Mathison B. A., Mehta N., & Couturier M. R. (2021). Human acanthocephaliasis: A thorn in the side of parasite diagnostics. Journal of Clinical Microbiology, 59, e0269120. 10.1128/jcm.02691-20 [Europe PMC free article] [Abstract] [CrossRef] [Google Scholar]
- Mattiucci S., Acerra V., Paoletti M., Cipriani P., Levsen A., Webb S. C., Canestrelli D., & Nascetti G. (2016). No more time to stay ‘single’ in the detection of Anisakis pegreffii, A. Simplex (s. s.) and hybridization events between them: A multi‐marker nuclear genotyping approach. Parasitology, 143, 998–1011. 10.1017/s0031182016000330 [Europe PMC free article] [Abstract] [CrossRef] [Google Scholar]
- Mattiucci S., Bello E., Paoletti M., Webb S. C., Timi J. T., Levsen A., Cipriani P., & Nascetti G. (2019). Novel polymorphic microsatellite loci in Anisakis pegreffii and A. Simplex (s. s.) (Nematoda: Anisakidae): Implications for species recognition and population genetic analysis. Parasitology, 146, 1387–1403. 10.1017/s003118201900074x [Abstract] [CrossRef] [Google Scholar]
- Mattiucci S., Cipriani P., Levsen A., Paoletti M., Nascetti G. (2018). Molecular epidemiology of Anisakis and Anisakiasis: An ecological and evolutionary road map. In Rollinson D. and Stothard J.R. (Eds.), Academic Press. Advances in Parasitology 99 (pp. 93–263). 10.1016/bs.apar.2017.12.001 [Abstract] [CrossRef] [Google Scholar]
- Mattiucci S., Cipriani P., Paoletti M., Levsen A., & Nascetti G. (2017). Reviewing biodiversity and epidemiological aspects of anisakid nematodes from the north‐east Atlantic ocean. Journal of Helminthology, 91, 422–439. 10.1017/s0022149x1700027x [Abstract] [CrossRef] [Google Scholar]
- Mattiucci S., Colantoni A., Crisafi B., Mori‐Ubaldini F., Caponi L., Fazii P., Nascetti G., & Bruschi F. (2017). IgE sensitization to Anisakis pegreffii in Italy: Comparison of two methods for the diagnosis of allergic anisakiasis. Parasite Immunology, 39. 10.1111/pim.12440 [Abstract] [CrossRef] [Google Scholar]
- Mattiucci S., Fazii P., De Rosa A., Paoletti M., Megna A. S., Glielmo A., De Angelis M., Costa A., Meucci C., Calvaruso V., Sorrentini I., Palma G., Bruschi F., & Nascetti G. (2013). Anisakiasis and gastroallergic reactions associated with Anisakis pegreffii infection, Italy. Emerging Infectious Diseases, 19, 496–499. 10.3201/eid1903.121017 [Europe PMC free article] [Abstract] [CrossRef] [Google Scholar]
- Mattiucci S., & Nascetti G. (2008). Advances and trends in the molecular systematics of anisakid nematodes, with implications for their evolutionary ecology and host‐parasite co‐evolutionary processes. Advances in Parasitology, 66, 47–148. 10.1016/s0065-308x(08)00202-9 [Abstract] [CrossRef] [Google Scholar]
- Mattiucci S., Paoletti M., Borrini F., Palumbo M., Palmieri R. M., Gomes V., Casati A., & Nascetti G. (2011). First molecular identification of the zoonotic parasite Anisakis pegreffii (Nematoda: Anisakidae) in a paraffin‐embedded granuloma taken from a case of human intestinal anisakiasis in Italy. BMC Infectious Diseases, 11, 82. 10.1186/1471-2334-11-82 [Europe PMC free article] [Abstract] [CrossRef] [Google Scholar]
- Mattiucci S., Paoletti M., Colantoni A., Carbone A., Gaeta R., Proietti A., Frattaroli S., Fazii P., Bruschi F., & Nascetti G. (2017). Invasive anisakiasis by the parasite Anisakis pegreffii (Nematoda: Anisakidae): Diagnosis by real‐time PCR hydrolysis probe system and immunoblotting assay. BMC Infectious Diseases, 17, 530. 10.1186/s12879-017-2633-0 [Europe PMC free article] [Abstract] [CrossRef] [Google Scholar]
- MedECC . (2020). Climate and environmental change in the Mediterranean Basin – Current situation and risks for the future (first Mediterranean assessment report). Union for the Mediterranean, Plan Bleu, UNEP/MAP, Marseille, France. 10.5281/zenodo.4768833 [CrossRef]
- Mehrdana F., Kania P. W., Nazemi S., & Buchmann K. (2017). Immunomodulatory effects of excretory/secretory compounds from Contracaecum osculatum larvae in a zebrafish inflammation model. PLoS One, 12, e0181277. 10.1371/journal.pone.0181277 [Europe PMC free article] [Abstract] [CrossRef] [Google Scholar]
- Mehrdana F., Lavilla M., Kania P. W., Pardo M. A., Audicana M. T., Longo N., & Buchmann K. (2021). Evidence of IgE‐mediated cross‐reactions between Anisakis simplex and Contracaecum osculatum proteins. Pathogens, 10. 10.3390/pathogens10080950 [Europe PMC free article] [Abstract] [CrossRef] [Google Scholar]
- Mehrdana F., Marana M. H., Skov J., Bahlool Q. Z., Sindberg D., Mundeling M., Overgaard B. C., Kania P. W., & Buchmann K. (2015). Eye fluke infection status in Baltic cod, Gadus morhua, after three decades and their use as ecological indicators. Acta Parasitologica, 60, 423–429. 10.1515/ap-2015-0058 [Abstract] [CrossRef] [Google Scholar]
- Menghi C. I., Gatta C. L., Arias L. E., Santoni G., Nicola F., Smayevsky J., Degese M. F., & Krivokapich S. J. (2020). Human infection with Pseudoterranova cattani by ingestion of “ceviche” in Buenos Aires, Argentina. Revista Argentina de Microbiología, 52, 118–120. 10.1016/j.ram.2019.06.005 [Abstract] [CrossRef] [Google Scholar]
- Mercken E., Van Damme I., Soba B., Vangeenberghe S., Serradell A., De Sterck T., Lumain J. P. L., & Gabriel S. (2022). Sensitivity of candling as routine method for the detection and recovery of ascaridoids in commercial fish fillets. Scientific Reports, 12, 1358. 10.1038/s41598-022-05235-6 [Europe PMC free article] [Abstract] [CrossRef] [Google Scholar]
- Mercken E., Van Damme I., Vangeenberghe S., Serradell A., De Sterck T., Lumain J. P. L., & Gabriël S. (2020). Ascaridoids in commercial fish: Occurrence, intensity and localization in whole fish and fillets destined for the Belgian market. International Journal of Food Microbiology, 327, 108657. 10.1016/j.ijfoodmicro.2020.108657 [Abstract] [CrossRef] [Google Scholar]
- Mladineo I. (2022). Functional signature of the Anisakis excretory gland cell. Proceedings of the ICOPA. Copenhagen, Denmark, 21–26 August 2022.
- Mladineo I., Charouli A., Jelić F., Chakroborty A., & Hrabar J. (2023). In vitro culture of the zoonotic nematode Anisakis pegreffii (Nematoda, Anisakidae). Parasites & Vectors, 16, 51. 10.1186/s13071-022-05629-5 [Europe PMC free article] [Abstract] [CrossRef] [Google Scholar]
- Mladineo I., Petrić M., Šegvić T., & Dobričić N. (2010). Scarcity of parasite assemblages in the Adriatic‐reared European sea bass (Dicentrarchus labrax) and sea bream (Sparus aurata). Veterinary Parasitology, 174, 131–138. 10.1016/j.vetpar.2010.08.015 [Abstract] [CrossRef] [Google Scholar]
- Mladineo I., & Poljak V. (2014). Ecology and genetic structure of zoonotic Anisakis spp. from Adriatic commercial fish species. Applied and Environmental Microbiology, 80, 1281–1290. 10.1128/AEM.03561-13 [Europe PMC free article] [Abstract] [CrossRef] [Google Scholar]
- Mladineo I., Popović M., Drmić‐Hofman I., & Poljak V. (2016). A case report of Anisakis pegreffii (Nematoda, Anisakidae) identified from archival paraffin sections of a Croatian patient. BMC Infectious Diseases, 16, 42. 10.1186/s12879-016-1401-x [Europe PMC free article] [Abstract] [CrossRef] [Google Scholar]
- Mladineo I., Segvić T., & Petrić M. (2011). Do captive conditions favor shedding of parasites in the reared Atlantic bluefin tuna (Thunnus thynnus)? Parasitology International, 60, 25–33. 10.1016/j.parint.2010.09.007 [Abstract] [CrossRef] [Google Scholar]
- Mladineo I., Trumbic Ž., Hrabar J., Vrbatovic A., Bušelic I., Ujevic I., Roje‐Busatto R., Babic I., & Messina C. (2018). Efficiency of target larvicides is conditioned by ABC‐mediated transport in the zoonotic nematode Anisakis pegreffii . Antimicrobial Agents and Chemotherapy, 62, e00916–e00918. 10.1128/aac.00916-18 [Europe PMC free article] [Abstract] [CrossRef] [Google Scholar]
- Mladineo I., Trumbić Ž., Radonić I., Vrbatović A., Hrabar J., & Bušelić I. (2017). Anisakis simplex complex: Ecological significance of recombinant genotypes in an allopatric area of the Adriatic Sea inferred by genome‐derived simple sequence repeats. International Journal for Parasitology, 47, 215–223. 10.1016/j.ijpara.2016.11.003 [Abstract] [CrossRef] [Google Scholar]
- Mo T. A., Gahr A., Hansen H., Hoel E., Oaland O., & Poppe T. T. (2014). Presence of Anisakis simplex (Rudolphi, 1809 det. Krabbe, 1878) and Hysterothylacium aduncum (Rudolphi, 1802) (Nematoda; Anisakidae) in runts of farmed Atlantic salmon, Salmo salar L. Journal of Fish Diseases, 37, 135–140. 10.1111/jfd.12096 [Abstract] [CrossRef] [Google Scholar]
- Moratal S., Dea‐Ayuela M. A., Martí‐Marco A., Puigcercós S., Marco‐Hirs N. M., Doménech C., Corcuera E., Cardells J., Lizana V., & López‐Ramon J. (2022). Molecular characterization of cryptosporidium spp. in cultivated and wild marine fishes from Western Mediterranean with the first detection of zoonotic cryptosporidium ubiquitum . Animals (Basel), 12. 10.3390/ani12091052 [Europe PMC free article] [Abstract] [CrossRef] [Google Scholar]
- Moratal S., Zrzavá M., Hrabar J., Dea‐Ayuela M. A., López‐Ramon J., & Mladineo I. (2023). Fecundity, in vitro early larval development and karyotype of the zoonotic nematode Anisakis pegreffii . Veterinary Parasitology, 323, 110050. 10.1016/j.vetpar.2023.110050 [Abstract] [CrossRef] [Google Scholar]
- Morsy K., Badr A. M., Abdel‐Ghaffar F., El Deeb S., & Ebead S. (2017). Pathogenic potential of fresh, frozen, and thermally treated Anisakis spp. type II (L3) (Nematoda: Anisakidae) after oral inoculation into Wistar rats: A histopathological study. Journal of Nematology, 49, 427–436. /article/pmc/5770291 [Europe PMC free article] [Abstract] [Google Scholar]
- Mossali C., Palermo S., Capra E., Piccolo G., Botti S., Bandi C., D'Amelio S., & Giuffra E. (2010). Sensitive detection and quantification of Anisakid parasite residues in food products. Foodborne Pathogens and Disease, 7, 391–397. 10.1089/fpd.2009.0428 [Abstract] [CrossRef] [Google Scholar]
- Nagasawa K. (2012). The biology of Contracaecum osculatum sensu lato and C. Osculatum a (Nematoda: Anisakidae) in Japanese waters: A review. Journal of Graduate School Biosphere Science Hiroshima University, 51, 61–69. 10.15027/34527 [CrossRef] [Google Scholar]
- Najjari M., Sadjjadi S. M., Khodadadi H., Farzaneh M. R., & Mattiucci S. (2022). Anisakis spp, DNA detection in paraffin‐embedded tissue biopsies recovered from patients with gastritis using real‐time PCR in Bushehr, Persian gulf, Iran. Molecular and Biochemical Parasitology, 251, 111494. 10.1016/j.molbiopara.2022.111494 [Abstract] [CrossRef] [Google Scholar]
- Nalbone L., Panebianco F., Cammilleri G., Ferrantelli V., & Giarratana F. (2022). Anisakicidal effects of R (+) limonene: An alternative to freezing treatment in the industrial anchovy marinating process. Food, 11. 10.3390/foods11081121 [Europe PMC free article] [Abstract] [CrossRef] [Google Scholar]
- Nam U. H., Kim J. O., & Kim J. H. (2020). De novo transcriptome sequencing and analysis of Anisakis pegreffii (Nematoda: Anisakidae) third‐stage and fourth stage larvae. Journal of Nematology, 52, 1–16. 10.21307/jofnem-2020-041 [Europe PMC free article] [Abstract] [CrossRef] [Google Scholar]
- Navarro‐Moll M. C., Romero M. C., Montilla M. P., & Valero A. (2011). In vitro and in vivo activity of three sesquiterpenes against L3 larvae of Anisakis type I. Experimental Parasitology, 127, 405–408. 10.1016/j.exppara.2010.09.008 [Abstract] [CrossRef] [Google Scholar]
- Nordholm A., Kurtzhals J. A. L., Karami A. M., Kania P. W., & Buchmann K. (2020). Nasal localization of a Pseudoterranova decipiens larva in a Danish patient with suspected allergic rhinitis. Journal of Helminthology, 94, e187. 10.1017/S0022149X20000681 [Abstract] [CrossRef] [Google Scholar]
- Ogata N., & Tagishi H. (2021). The inhibitory effect of wood creosote on the movement of Anisakis larvae: an implication for the treatment of acute anisakiasis. Pharmacology, 106, 637–643. 10.1159/000518961 [Abstract] [CrossRef] [Google Scholar]
- Oh S. R., Zhang C. Y., Kim T. I., Hong S. J., Ju I. S., Lee S. H., Kim S. H., Cho J. I., & Ha S. D. (2014). Inactivation of Anisakis larvae in salt‐fermented squid and pollock tripe by freezing, salting, and combined treatment with chlorine and ultrasound. Food Control, 40, 46–49. 10.1016/j.foodcont.2013.11.023 [CrossRef] [Google Scholar]
- Onitsuka C., Nakamura K., Wang D., Matsuda M., Tanaka R., Inoue Y., & Namihira T. (2024). Dependence of anisakid larva inactivation by pulsed power on various parameters. Journal of Food Engineering, 360, 111715. 10.1016/j.jfoodeng.2023.111715 [CrossRef] [Google Scholar]
- Onitsuka C., Nakamura K., Wang D. Y., Matsuda M., Tanaka R., Inoue Y., Kuroda R., Noda T., Negoro K., Negoro T., & Namihira T. (2022). Inactivation of anisakis larva using pulsed power technology and quality evaluation of horse mackerel meat treated with pulsed power. Fisheries Science, 88, 337–344. 10.1007/s12562-022-01593-2 [CrossRef] [Google Scholar]
- Onsurathum S., Pinlaor P., Haonon O., Chaidee A., Charoensuk L., Intuyod K., Boonmars T., Laummaunwai P., & Pinlaor S. (2016). Effects of fermentation time and low temperature during the production process of Thai pickled fish (pla‐som) on the viability and infectivity of Opisthorchis viverrini metacercariae. International Journal of Food Microbiology, 218, 1–5. 10.1016/j.ijfoodmicro.2015.11.001 [Abstract] [CrossRef] [Google Scholar]
- Ozkoc S. O., Sumnu G., & Sahin S. (2014). Recent developments in microwave heating. In D.‐W. Sun , editor. (Ed.), Emerging technologies for food processing, Acedemic Press; (pp. 361–383). 10.1016/B978-0-12-411479-1.01001-9 [CrossRef] [Google Scholar]
- Paggi L., Nascetti G., Cianchi R., Orecchia P., Mattiucci S., D'Amelio S., Berland B., Brattey J., Smith J. W., & Bullini L. (1991). Genetic evidence for three species within Pseudoterranova decipiens (Nematoda, Ascaridida, Ascaridoidea) in the North Atlantic and Norwegian and Barents seas. International Journal for Parasitology, 21, 195–212. 10.1016/0020-7519(91)90010-5 [Abstract] [CrossRef] [Google Scholar]
- Palmer R. (1994). Kudoa–the Irish experience. Proceedings Kudoa Workshop, Nanaimo, BC, 17–18 February, Aquaculture Industry development Report 94–01, 18–21 pp. D. C. Conley (ed.), British Columbia Ministry of Agriculture, Fisheries & Food, Victoria, British Columbia, Canada.
- Palomba M., Cipriani P., Giulietti L., Levsen A., Nascetti G., & Mattiucci S. (2020). Differences in gene expression profiles of seven target proteins in third‐stage larvae of Anisakis simplex (sensu stricto) by sites of infection in blue whiting (Micromesistius poutassou). Genes (Basel), 11. 10.3390/genes11050559 [Europe PMC free article] [Abstract] [CrossRef] [Google Scholar]
- Palomba M., Libro P., Di Martino J., Roca‐Geronès X., Macali A., Castrignanò T., Canestrelli D., & Mattiucci S. (2023). De novo transcriptome assembly of an Antarctic nematode for the study of thermal adaptation in marine parasites. Scientific Data, 10, 720. 10.1038/s41597-023-02591-4 [Europe PMC free article] [Abstract] [CrossRef] [Google Scholar]
- Palomba M., Libro P., Di Martino J., Rughetti A., Santoro M., Mattiucci S., & Castrignanò T. (2022). De novo transcriptome assembly and annotation of the third stage larvae of the zoonotic parasite Anisakis pegreffii . BMC Research Notes, 15, 223. 10.1186/s13104-022-06099-9 [Europe PMC free article] [Abstract] [CrossRef] [Google Scholar]
- Palomba M., Marchiori E., Tedesco P., Fioravanti M., Marcer F., Gustinelli A., Aco‐Alburqueque R., Belli B., Canestrelli D., Santoro M., Cipriani P., & Mattiucci S. (2023). An update and ecological perspective on certain sentinel helminth endoparasites within the Mediterranean Sea. Parasitology, 150(12), 1–19. 10.1017/s0031182023000951 [Europe PMC free article] [Abstract] [CrossRef] [Google Scholar]
- Palomba M., Paoletti M., Colantoni A., Rughetti A., Nascetti G., & Mattiucci S. (2019). Gene expression profiles of antigenic proteins of third stage larvae of the zoonotic nematode Anisakis pegreffii in response to temperature conditions. Parasite, 26, 52. 10.1051/parasite/2019055 [Europe PMC free article] [Abstract] [CrossRef] [Google Scholar]
- Palomba M., Paoletti M., Webb S. C., Nascetti G., & Mattiucci S. (2020). A novel nuclear marker and development of an ARMS‐PCR assay targeting the metallopeptidase 10 (nas 10) locus to identify the species of the Anisakis simplex (s. l.) complex (Nematoda, Anisakidae). Parasite, 27, 39. 10.1051/parasite/2020033 [Europe PMC free article] [Abstract] [CrossRef] [Google Scholar]
- Palomba M., Rughetti A., Mignogna G., Castrignanò T., Rahimi H., Masuelli L., Napoletano C., Pinna V., Giorgi A., Santoro M., Schininà M. E., Maras B., & Mattiucci S. (2023). Proteomic characterization of extracellular vesicles released by third stage larvae of the zoonotic parasite Anisakis pegreffii (Nematoda: Anisakidae). Frontiers in Cellular and Infection Microbiology, 13. 10.3389/fcimb.2023.1079991 [Europe PMC free article] [Abstract] [CrossRef] [Google Scholar]
- Paoletti M., Mattiucci S., Colantoni A., Levsen A., Gay M., & Nascetti G. (2018). Species‐specific real time‐PCR primers/probe systems to identify fish parasites of the genera Anisakis, Pseudoterranova and Hysterothylacium (Nematoda: Ascaridoidea). Fisheries Research, 202, 38–48. 10.1016/j.fishres.2017.07.015 [CrossRef] [Google Scholar]
- PARASITE Consortium . (2016). Parasite risk assessment with integrated tools in EU fish production: Report on treatments for killing parasites in fishery Product. (Collaborative Project Targeted to a Special Group). European Union's Seventh Framework Programme for research, technological development and demonstration under grant agreement no 312 068. https://cordis.europa.eu/project/id/312068
- Pardo González M., Cavazza G., Gustinelli A., Caffara M., & Fioravanti M. (2020). Absence of anisakis nematodes in smoked farmed Atlantic salmon (Salmo salar) products on sale in European countries. Italian Journal of Food Safety, 9, 8615. 10.4081/ijfs.2020.8615 [Europe PMC free article] [Abstract] [CrossRef] [Google Scholar]
- Pascual S., Antonio J., Cabo M. L., & Piñeiro C. (2010). Anisakis survival in refrigerated fish products under CO2 modified‐atmosphere. Food Control, 21, 1254–1256. 10.1016/j.foodcont.2010.03.002 [CrossRef] [Google Scholar]
- Pavanelli G. C., Simas I. P. N., Gonçalves J. E., & Castro A. (2018). Ginger oil (Zingiber officinale) in the fight against larvae of Contracaecum sp. that cause human zoonoses. Mundo saúde (Impr.):[534–547], 42, 534–547. [Google Scholar]
- Peñalver J., Dolores E. M., & Muñoz P. (2010). Absence of anisakid larvae in farmed European sea bass (Dicentrarchus labrax L.) and gilthead sea bream (Sparus aurata L) in Southeast Spain. Journal of Food Protection, 73, 1332–1334. 10.4315/0362-028x-73.7.1332 [Abstract] [CrossRef] [Google Scholar]
- Podolska M., Pawlikowski B., Nadolna‐Ałtyn K., Pawlak J., Komar‐Szymczak K., & Szostakowska B. (2019). How effective is freezing at killing Anisakis simplex, Pseudoterranova krabbei, and P. Decipiens larvae? An experimental evaluation of time‐temperature conditions. Parasitology Research, 118, 2139–2147. 10.1007/s00436-019-06339-1 [Europe PMC free article] [Abstract] [CrossRef] [Google Scholar]
- Pozio E., Armignacco O., Ferri F., & Gomez Morales M. A. (2013). Opisthorchis felineus, an emerging infection in Italy and its implication for the European Union. Acta Tropica, 126, 54–62. 10.1016/j.actatropica.2013.01.005 [Abstract] [CrossRef] [Google Scholar]
- Radačovská A., Bazsalovicsová E., & Králová‐Hromadová I. (2019). Results on search for the broad fish tapeworm Dibothriocephalus latus (Linnaeus, 1758), (syn. Diphyllobothrium latum) (Cestoda: Diphyllobothriidea), in the Danube River. Helminthologia, 56, 256–260. 10.2478/helm-2019-0001 [Europe PMC free article] [Abstract] [CrossRef] [Google Scholar]
- Raether W., & Hänel H. (2003). Epidemiology, clinical manifestations and diagnosis of zoonotic cestode infections: An update. Parasitology Research, 91, 412–438. 10.1007/s00436-003-0903-9 [Abstract] [CrossRef] [Google Scholar]
- Rausch R. L., Scott E. M., & Rausch V. R. (1967). Helminths in eskimos in Western Alaska, with particular reference to Diphyllobothrium infection and anaemia. Transactions of the Royal Society of Tropical Medicine and Hygiene, 61, 351–357. 10.1016/0035-9203(67)90008-9 [CrossRef] [Google Scholar]
- Roca‐Geronès X., Alcover M. M., Godínez‐González C., González‐Moreno O., Masachs M., Fisa R., & Montoliu I. (2020). First molecular diagnosis of clinical cases of gastric anisakiosis in Spain. Genes (Basel), 11(4), 452. 10.3390/genes11040452 [Europe PMC free article] [Abstract] [CrossRef] [Google Scholar]
- Roiha I. S., Maage A., & Levsen A. (2021). Farmed rainbow trout (Oncorhynchus mykiss) in Norway are at low risk of carrying anisakid nematodes. Journal of Applied Aquaculture, 33, 279–290. 10.1080/10454438.2020.1785368 [CrossRef] [Google Scholar]
- Romero M., Valero A., Navarro‐Moll M. C., & Martín‐Sánchez J. (2013). Experimental comparison of pathogenic potential of two sibling species Anisakis simplex s.s. and Anisakis pegreffii in Wistar rat. Tropical Medicine & International Health, 18, 979–984. 10.1111/tmi.12131 [Abstract] [CrossRef] [Google Scholar]
- Romero M. C., Navarro M. C., Martín‐Sánchez J., & Valero A. (2014). Peppermint (Mentha piperita) and albendazole against anisakiasis in an animal model. Tropical Medicine & International Health, 19, 1430–1436. 10.1111/tmi.12399 [Abstract] [CrossRef] [Google Scholar]
- Romero M. C., Valero A., Martín‐Sánchez J., & Navarro‐Moll M. C. (2012). Activity of Matricaria chamomilla essential oil against anisakiasis. Phytomedicine, 19, 520–523. 10.1016/j.phymed.2012.02.005 [Abstract] [CrossRef] [Google Scholar]
- Ruitenberg E. J. (1970). Anisakiasis. pathogenesis, serodiagnosis and prevention. Chapter V “Methods to examine the penetration capacity of Anisakis larvae” 67–74. 138 pp. Publisher: Rijksuniversiteit, Utrecht. CABI Record Number: 19730805062.
- Saelens G., Planckaert S., Martínez‐Sernández V., Ubeira F. M., Devreese B., & Gabriël S. (2022). Targeted proteomics and specific immunoassays reveal the presence of shared allergens between the zoonotic nematodes Anisakis simplex and Pseudoterranova decipiens . Scientific Reports, 12, 4127. 10.1038/s41598-022-08113-3 [Europe PMC free article] [Abstract] [CrossRef] [Google Scholar]
- Salati F., Meloni M., Cau M., & Angelucci G. (2013). Presence of Contracaecum spp. in teleosts cultured and fished in Sardinia. Veter Parasitol, 196(3), 382–387. 10.1016/j.vetpar.2013.03.014 [Abstract] [CrossRef] [Google Scholar]
- Sales K., Miranda D. E. O., da Silva F. J., Otranto D., Figueredo L. A., & Dantas‐Torres F. (2020). Evaluation of different storage times and preservation methods on phlebotomine sand fly DNA concentration and purity. Parasites & Vectors, 13, 399. 10.1186/s13071-020-04270-4 [Europe PMC free article] [Abstract] [CrossRef] [Google Scholar]
- Sampels S. (2015). The effects of processing technologies and preparation on the final quality of fish products. Trends Food Science Technology, 44(2), 131–146. 10.1016/j.tifs.2015.04.003 [CrossRef] [Google Scholar]
- Sánchez‐Alonso I., Carballeda‐Sangiao N., González‐Muñoz M., Arcos S. C., Navas A., & Careche M. (2021). Thermal patterns of heat treated Anisakis L3‐infected fishery products allow separation into low, intermediate and high risk groups of potential use in risk management. Food Control, 124, 107837. 10.1016/j.foodcont.2020.107837 [CrossRef] [Google Scholar]
- Sánchez‐Alonso I., Carballeda‐Sangiao N., González‐Muñoz M., Navas A., Arcos S. C., Mendizábal A., Cuesta F., & Careche M. (2020). Freezing kinetic parameters influence allergenic and infective potential of Anisakis simplex L3 present in fish muscle. Food Control, 118, 107373. 10.1016/j.foodcont.2020.107373 [CrossRef] [Google Scholar]
- Sánchez‐Alonso I., Carballeda‐Sangiao N., González‐Muñoz M., Navas A., Arcos S. C., Mendizábal A., Tejada M., & Careche M. (2018). Pathogenic potential of Anisakis L3 after freezing in domestic freezers. Food Control, 84, 61–69. 10.1016/j.foodcont.2017.07.010 [CrossRef] [Google Scholar]
- Sánchez‐Alonso I., Carballeda‐Sangiao N., Rodríguez S., Tejada M., Navas A., Arcos S. C., González‐Muñoz M., & Careche M. (2021). Anisakis simplex (s.l.) resistance to the action of gastric enzymes depends upon previous treatments applied to infected fish mince and affects antigen release. Journal of the Science of Food and Agriculture, 101, 3908–3916. 10.1002/jsfa.11031 [Abstract] [CrossRef] [Google Scholar]
- Sánchez‐Alonso I., Navas A., Arcos S. C., González‐Muñoz M., Carballeda‐Sangiao N., & Careche M. (2019). Respiratory analysis as a tool to detect physiological changes in Anisakis larvae subjected to stress. Parasitology Research, 118, 1127–1135. 10.1007/s00436-019-06260-7 [Abstract] [CrossRef] [Google Scholar]
- Sánchez‐Alonso I., Rodríguez S., Tejada M., Navas A., González‐Muñoz M., & Careche M. (2021). The artificial digestion method underestimates the viability of Anisakis simplex (s.l.) L3 present in processed fish products. Food and Waterborne Parasitology, 23, e00121. 10.1016/j.fawpar.2021.e00121 [Europe PMC free article] [Abstract] [CrossRef] [Google Scholar]
- Sándor D., Gyöngy M., Nyeste K., Czeglédi I., Székely C., Buchmann K., & Cech G. (2020). Digenean Holostephanus (Trematoda: Digenea: Cyathocotylidae) metacercariae in common carp (Cyprinus carpio Linnaeus, 1758) muscle: Zoonotic potential and sensitivity to physico‐chemical treatments. Journal of Helminthology, 94, e117. 10.1017/S0022149X1900110X [Abstract] [CrossRef] [Google Scholar]
- Santoro M., Viscardi M., Boccia F., Borriello G., Lucibelli M. G., Auriemma C., Anastasio A., Veneziano V., Galiero G., & Baldi L. (2020). Parasite load and STRs genotyping of toxoplasma gondii isolates from Mediterranean mussels (Mytilus galloprovincialis) in southern Italy. Frontiers in Microbiology, 11, 355. 10.3389/fmicb.2020.00355 [Europe PMC free article] [Abstract] [CrossRef] [Google Scholar]
- Santos M. J., Matos M., Guardone L., Golden O., Armani A., Caldeira A. J. R., & Vieira‐Pinto M. (2022). Preliminary data on the occurrence of Anisakis spp. in European hake (Merluccius merluccius) caught off the Portuguese coast and on reports of human anisakiosis in Portugal. Microorganisms, 10, 331. 10.3390/microorganisms10020331 [Europe PMC free article] [Abstract] [CrossRef] [Google Scholar]
- Setyawan A. C., Jensen H. M., Kania P. W., & Buchmann K. (2020). Baltic cod endohelminths reflect recent ecological changes. Journal of Helminthology, 94, e155. 10.1017/S0022149X20000176 [Abstract] [CrossRef] [Google Scholar]
- Setyawan A. C., Zuo S., Kania P. W., & Buchmann K. (2019). Endoparasitic helminths in Baltic salmon Salmo salar: Ecological implications. Diseases of Aquatic Organisms, 135, 193–199. 10.3354/dao03391 [Abstract] [CrossRef] [Google Scholar]
- Shamsi S., & Barton D. P. (2023). A critical review of anisakidosis cases occurring globally. Parasitology Research, 122, 1733–1745. 10.1007/s00436-023-07881-9 [Europe PMC free article] [Abstract] [CrossRef] [Google Scholar]
- Shamsi S., & Butcher A. R. (2011). First report of human anisakidosis in Australia. Medical Journal of Australia, 194, 199–200. 10.5694/j.1326-5377.2011.tb03772.x [Abstract] [CrossRef] [Google Scholar]
- Sidorova T. V., Kutyrev I. A., Khabudaev K. V., Sukhanova L. V., Zheng Y., Dugarov Z. N., & Mazur O. E. (2023). Comparative transcriptomic analysis of the larval and adult stages of Dibothriocephalus dendriticus (Cestoda: Diphyllobothriidea). Parasitology Research, 122, 145–156. 10.1007/s00436-022-07708-z [Abstract] [CrossRef] [Google Scholar]
- Šimat V., & Trumbić Ž. (2019). Viability of Anisakis spp. larvae after direct exposure to different processing media and non‐thermal processing in anchovy fillets. Fishes, 4(1), 19. 10.3390/fishes4010019 [CrossRef] [Google Scholar]
- Simonetta S. H., & Golombek D. A. (2007). An automated tracking system for Caenorhabditis elegans locomotor behavior and circadian studies application. Journal of Neuroscience Methods, 161, 273–280. 10.1016/j.jneumeth.2006.11.015 [Abstract] [CrossRef] [Google Scholar]
- Sithithaworn P., Saijunta W., Andrews R. H., & Petney T. N. (2015). Chapter 16. Clonorchis, Opistorchis and Metorchis. In L. Xiao , editor; , U. Ryan , editor; , & Y. Feng , editor. (Eds.), Biology of foodborne parasites: Section III important foodborne helminths (1st ed., pp. 275–298). Food Microbiology Series . 10.1201/b18317 [CrossRef] [Google Scholar]
- Sivertsen A. H., Heia K., Hindberg K., & Godtliebsen F. (2012). Automatic nematode detection in cod fillets (Gadus morhua L.) by hyperspectral imaging. Journal of Food Engineering, 111, 675–681. 10.1016/j.jfoodeng.2012.02.036 [Abstract] [CrossRef] [Google Scholar]
- Sivertsen A. H., Heia K., Stormo S. K., Elvevoll E., & Nilsen H. (2011). Automatic nematode detection in cod fillets (Gadus morhua) by transillumination hyperspectral imaging. Journal of Food Science, 76, S77–S83. 10.1111/j.1750-3841.2010.01928.x [Abstract] [CrossRef] [Google Scholar]
- Skirnisson K. (2022). Human Pseudoterranova and Anisakis cases in Iceland 2004–2020. Læknablađiđ, 108, 79–83. [Abstract] [Google Scholar]
- Skov J., Kania P. W., Dalsgaard A., Jørgensen T. R., & Buchmann K. (2009). Life cycle stages of heterophyid trematodes in Vietnamese freshwater fishes traced by molecular and morphometric methods. Veterinary Parasitology, 160, 66–75. 10.1016/j.vetpar.2008.10.088 [Abstract] [CrossRef] [Google Scholar]
- Skov J., Kania P. W., Jørgensen T. R., & Buchmann K. (2008). Molecular and morphometric study of metacercariae and adults of Pseudamphistomum truncatum (Opisthorchiidae) from roach (Rutilus rutilus) and wild American mink (Mustela vison). Veterinary Parasitology, 155, 209–216. 10.1016/j.vetpar.2008.05.011 [Abstract] [CrossRef] [Google Scholar]
- Skov J., Mehrdana F., Marana M. H., Bahlool Q. Z. M., Jaafar R. M., Sindberg D., Jensen H. M., Kania P. W., & Buchmann K. (2014). Parasite infections of rainbow trout (Oncorhynchus mykiss) from Danish mariculture. Aquaculture, 434, 486–492. 10.1016/j.aquaculture.2014.08.041 [CrossRef] [Google Scholar]
- Smaldone G., Marrone R., Palma G., Sarnelli P., & Anastasio A. (2017). Preliminary study on the inactivation of anisakid larvae in baccalà prepared according to traditional methods. Italian Journal of Food Safety, 6, 195–198. 10.4081/ijfs.2017.6964 [Europe PMC free article] [Abstract] [CrossRef] [Google Scholar]
- Sobecka E., Wierzbicka J., & Hałupka M. (2010). A comparative study on the parasite fauna of Wels catfish Silurus glanis (L.) from a heated‐water‐channel fish farm and a natural water body. Bulletin of the European Association of Fish Pathologists, 30, 8–14. https://eafp.org/download/2010‐Volume30/Issue1/Sobecka.pdf [Google Scholar]
- Song H., Ryoo S., Jung B. K., Cho J., Chang T., Hong S., Shin H., Sohn W. M., & Chai J. Y. (2022). Molecular diagnosis of Pseudoterranova decipiens sensu stricto infections, South Korea, 2002–2020. Emerging Infectious Diseases, 28, 1283–1285. 10.3201/eid2806.212483 [Europe PMC free article] [Abstract] [CrossRef] [Google Scholar]
- Stenevik E., & Sundby S. (2007). Impacts of climate change on commercial fish stocks in Norwegian waters. Marine Policy, 31, 19–31. 10.1016/j.marpol.2006.05.001 [CrossRef] [Google Scholar]
- Strøm S. B., Haarder S., Korbut R., Mejer H., Thamsborg S. M., Kania P. W., & Buchmann K. (2015). Third‐stage nematode larvae of Contracaecum osculatum from Baltic cod (Gadus morhua) elicit eosinophilic granulomatous reactions when penetrating the stomach mucosa of pigs. Parasitology Research, 114, 1217–1220. 10.1007/s00436-014-4306-x [Abstract] [CrossRef] [Google Scholar]
- Stryiński R., Mateos J., Carrera M., Jastrzębski J. P., Bogacka I., & Łopieńska‐Biernat E. (2022). Tandem mass tagging (TMT) reveals tissue‐specific proteome of L4 larvae of Anisakis simplex s. s.: Enzymes of energy and/or carbohydrate metabolism as potential drug targets in anisakiasis. International Journal of Molecular Sciences, 23, 4336. 10.3390/ijms23084336 [Europe PMC free article] [Abstract] [CrossRef] [Google Scholar]
- Stryiński R., Mateos J., Pascual S., González Á. F., Gallardo J. M., Łopieńska‐Biernat E., Medina I., & Carrera M. (2019). Proteome profiling of L3 and L4 Anisakis simplex development stages by TMT‐based quantitative proteomics. Journal of Proteomics, 201, 1–11. 10.1016/j.jprot.2019.04.006 [Abstract] [CrossRef] [Google Scholar]
- Sung G. H., Park I. J., Koo H. S., Park E. H., & Lee M. O. (2023). Molecular detection and genotype analysis of Kudoa septempunctata from food poisoning outbreaks in Korea. Parasites, Hosts and Diseases, 61, 15–23. [Europe PMC free article] [Abstract] [Google Scholar]
- Suzuki J., Murata R., Hosaka M., & Araki J. (2010). Risk factors for human Anisakis infection and association between the geographic origins of Scomber japonicus and anisakid nematodes. International Journal of Food Microbiology, 137, 88–93. 10.1016/j.ijfoodmicro.2009.10.001 [Abstract] [CrossRef] [Google Scholar]
- Suzuki J., Murata R., & Kodo Y. (2021). Current status of anisakiasis and Anisakis larvae in Tokyo, Japan. Food Safety (Tokyo), 9, 89–100. 10.14252/foodsafetyfscj.D-21-00004 [Europe PMC free article] [Abstract] [CrossRef] [Google Scholar]
- Suzuki J., Murata R., Yokoyama H., Sadamasu K., & Kai A. (2015). Detection rate of diarrhoea‐causing Kudoa hexapunctata in Pacific bluefin tuna Thunnus orientalis from Japanese waters. International Journal of Food Microbiology, 194, 1–6. 10.1016/j.ijfoodmicro.2014.11.001 [Abstract] [CrossRef] [Google Scholar]
- Sy I., Conrad L., & Becker S. L. (2022). Recent advances and potential future applications of MALDI‐TOF mass spectrometry for identification of helminths. Diagnostics, 12, 3035. 10.3390/diagnostics12123035 [Europe PMC free article] [Abstract] [CrossRef] [Google Scholar]
- Taki A. C., Byrne J. J., Wang T., Sleebs B. E., Nguyen N., Hall R. S., Korhonen P. K., Chang B. C. H., Jackson P., Jabbar A., & Gasser R. B. (2021). High‐throughput phenotypic assay to screen for anthelmintic activity on Haemonchus contortus . Pharmaceuticals (Basel), 14(7), 616. 10.3390/ph14070616 [Europe PMC free article] [Abstract] [CrossRef] [Google Scholar]
- Tavares‐Dias M. (2018). Current knowledge on use of essential oils as alternative treatment against fish parasites. Aquatic Living Resources, 31, 13. 10.1051/alr/2018001 [CrossRef] [Google Scholar]
- Tedde T., Marangi M., Papini R., Salza S., Normanno G., Virgilio S., & Giangaspero A. (2019). Toxoplasma gondii and other zoonotic protozoans in Mediterranean mussel (Mytilus galloprovincialis) and blue mussel (Mytilus edulis): A food safety concern? Journal of Food Protection, 82, 535–542. 10.4315/0362-028X.JFP-18-157 [Abstract] [CrossRef] [Google Scholar]
- Tejada M., Solas M. T., Navas A., & Mendizábal A. (2006). Scanning electron microscopy of Anisakis larvae following different treatments. Journal of Food Protection, 69, 1379–1387. 10.4315/0362-028X-69.6.1379 [Abstract] [CrossRef] [Google Scholar]
- Thaenkham U., Phuphisut O., Pakdee W., Homsuwan N., Sa‐nguankiat S., Waikagul J., Nawa Y., & Dung T. (2011). Rapid and simple identification of human pathogenic heterophyid intestinal fluke metacercariae by PCR‐RFLP. Parasitology International, 60, 503–506. 10.1016/j.parint.2011.09.004 [Abstract] [CrossRef] [Google Scholar]
- Trabelsi N., Marotta S. M., Giarratana F., Taamali A., Zarrouk M., Ziino G., & Giuffrida A. (2018). Use of Tunisian flavored olive oil as anisakicidal agent in industrial anchovy marinating process. Journal of the Science of Food and Agriculture, 98, 3446–3451. 10.1002/jsfa.8857 [Abstract] [CrossRef] [Google Scholar]
- Trabelsi N., Nalbone L., Di Rosa A. R., Ed‐Dra A., Nait‐Mohamed S., Mhamdi R., Giuffrida A., & Giarratana F. (2021). Marinated anchovies (Engraulis encrasicolus) prepared with flavored olive oils (Chétoui cv.): Anisakicidal effect, microbiological, and sensory evaluation. Sustainability, 13(9), 5310. 10.3390/su13095310 [CrossRef] [Google Scholar]
- Trabelsi N., Nalbone L., Marotta S. M., Taamali A., Abaza L., & Giarratana F. (2019). Effectiveness of five flavored Tunisian olive oils on Anisakis larvae type 1: Application of cinnamon and rosemary oil in industrial anchovy marinating process. Journal of the Science of Food and Agriculture, 99, 4808–4815. 10.1002/jsfa.9736 [Abstract] [CrossRef] [Google Scholar]
- Trumbić Ž., Hrabar J., Palevich N., Carbone V., & Mladineo I. (2021). Molecular and evolutionary basis for survival, its failure, and virulence factors of the zoonotic nematode Anisakis pegreffii . Genomics, 113, 2891–2905. 10.1016/j.ygeno.2021.06.032 [Abstract] [CrossRef] [Google Scholar]
- Uberoi E., Hutton G., Ward M., & Ares E. (2022). UK fisheries statistics. House of Commons library. https://commonslibrary.parliament.uk/research‐briefings/sn02788/ [Google Scholar]
- Valero A., Romero M. C., Gómez‐Mateos M., Hierro I., & Navarro M. C. (2015). Natural products: Perspectives in the pharmacological treatment of gastrointestinal anisakiasis. Asian Pacific Journal of Tropical Medicine, 8, 612–617. 10.1016/j.apjtm.2015.07.017 [Abstract] [CrossRef] [Google Scholar]
- Vidaček S., De Las Heras C., Solas M. T., García M. L., Mendizabal A., & Tejada M. (2011). Viability and antigenicity of Anisakis simplex after conventional and microwave heating at fixed temperatures. Journal of Food Protection, 74, 2119–2126. 10.4315/0362-028X.JFP-11-108 [Abstract] [CrossRef] [Google Scholar]
- Vidaček S., de las Heras C., Solas M. T., Mendizabal A., Rodriguez‐Mahillo A. I., González‐Muñoz M., & Tejada M. (2009). Anisakis simplex allergens remain active after conventional or microwave heating and pepsin treatments of chilled and frozen L3 larvae. Journal of the Science of Food and Agriculture, 89, 1997–2002. 10.1002/jsfa.3677 [CrossRef] [Google Scholar]
- Vidaček S., de las Heras C., Solas M. T., Mendizábal A., Rodríguez‐Mahillo A. I., & Tejada M. (2010). Antigenicity and viability of Anisakis larvae infesting hake heated at different time‐temperature conditions. Journal of Food Protection, 73, 62–68. http://hdl.handle.net/10261/67388 [Abstract] [Google Scholar]
- Vidacek S., de las Heras C., Solas M. T., Rodríguez Mahillo A. I., & Tejada M. (2009). Effect of high hydrostatic pressure on mortality and allergenicity of Anisakis simplex L3 and on muscle properties of infested hake. Journal of the Science of Food and Agriculture, 89, 2228–2235. 10.1002/jsfa.3712 [CrossRef] [Google Scholar]
- Waeschenbach A., & Littlewood T. (2017). A molecular framework for the Cestoda. In J. JNCaK , editor. (Ed.), Planetary biodiversity inventory (2008–2017): Tapeworms from vertebrate bowels of the earth (pp. 431–451). University of Kansas, Natural History Museum. https://nhm.openrepository.com/bitstream/handle/10141/622760/2017_PBICestoda_Chapt22_Molecules.pdf?sequence=1 [Google Scholar]
- Wąsikowska B., & Linowska A. A. (2021). Application of the rough set theory to the analysis of food safety in fish processing. Procedia Computer Science, 192, 3342–3350. 10.1016/j.procs.2021.09.107 [CrossRef] [Google Scholar]
- Wąsikowska B., Sobecka E., Bielat I., Legierko M., & Więcaszek B. (2018). A novel method for predicting anisakid nematode infection of Atlantic cod using rough set theory. Journal of Food Protection, 81(3), 502–508. 10.4315/0362-028x.Jfp-17-371 [Abstract] [CrossRef] [Google Scholar]
- Weitzel T., Sugiyama H., Yamasaki H., Ramirez C., Rosas R., & Mercado R. (2015). Human infections with Pseudoterranova cattani nematodes, Chile. Emerging Infectious Diseases, 21, 1874–1875. 10.3201/eid2110.141848 [Europe PMC free article] [Abstract] [CrossRef] [Google Scholar]
- Werner M. T., Faeste C. K., Levsen A., & Egaas E. (2011). A quantitative sandwich ELISA for the detection of Anisakis simplex protein in seafood. European Food Research and Technology, 232, 157–166. 10.1007/s00217-010-1373-9 [CrossRef] [Google Scholar]
- White H. D., Bates M. J., & Wilson P. (1992). For information specialists: Interpretations of reference and bibliographic work. Norwood, N.J., Ablex pub. Norwood, N.J. v, 310 pages. ISBN‐10: 0893919837.
- Xu S., Lu H., Fan C., Qiu G., Ference C., Liang X., & Peng J. (2023). Visible and near‐infrared hyperspectral imaging as an intelligent tool for parasite detection in sashimi. LWT, 181, 114747. 10.1016/j.lwt.2023.114747 [CrossRef] [Google Scholar]
- Zuloaga J., Rodríguez‐Bobada C., Corcuera M. T., Gómez‐Aguado F., González P., Rodríguez‐ Pérez R., Arias‐Díaz J., & Caballero M. L. (2013). A rat model of intragastric infection with Anisakis spp. live larvae: Histopathological study. Parasitology Research, 112, 2409–2411. 10.1007/s00436-013-3359-6 [Abstract] [CrossRef] [Google Scholar]
- Zuo S., Barlaup L., Mohammadkarami A., Al‐Jubury A., Chen D., Kania P. W., & Buchmann K. (2017). Extrusion of Contracaecum osculatum nematode larvae from the liver of cod (Gadus morhua). Parasitology Research, 116, 2721–2726. 10.1007/s00436-017-5580-1 [Abstract] [CrossRef] [Google Scholar]
Articles from EFSA Journal are provided here courtesy of Wiley
Full text links
Read article at publisher's site: https://doi.org/10.2903/j.efsa.2024.8719
Read article for free, from open access legal sources, via Unpaywall:
https://onlinelibrary.wiley.com/doi/pdfdirect/10.2903/j.efsa.2024.8719
Citations & impact
Impact metrics
Citations of article over time
Alternative metrics

Discover the attention surrounding your research
https://www.altmetric.com/details/162829728
Article citations
Sequence Segmentation of Nematodes in Atlantic Cod with Multispectral Imaging Data.
Foods, 13(18):2952, 18 Sep 2024
Cited by: 0 articles | PMID: 39335880 | PMCID: PMC11430828
Prevalence of Anisakiasis in Madrid (Spain) after 20 Years of Preventive Legislation.
Pathogens, 13(9):782, 11 Sep 2024
Cited by: 0 articles | PMID: 39338973 | PMCID: PMC11435309
Re-evaluation of certain aspects of the EFSA Scientific Opinion of April 2010 on risk assessment of parasites in fishery products, based on new scientific data. Part 1: ToRs1-3.
EFSA J, 22(4):e8719, 22 Apr 2024
Cited by: 14 articles | PMID: 38650612 | PMCID: PMC11033839
The Occurrence of Freshwater Fish-Borne Zoonotic Helminths in Italy and Neighbouring Countries: A Systematic Review.
Animals (Basel), 13(24):3793, 08 Dec 2023
Cited by: 0 articles | PMID: 38136832 | PMCID: PMC10741178
Review Free full text in Europe PMC
Assessing Portuguese health risks: <i>Anisakiks</i> parasite in Atlantic chub mackerel (<i>Scomber colias</i>) sold in Portuguese markets.
EFSA J, 21(suppl 1):e211004, 30 Nov 2023
Cited by: 1 article | PMID: 38047133 | PMCID: PMC10687749
Go to all (14) article citations
Data
Data behind the article
This data has been text mined from the article, or deposited into data resources.
Data Citations
- (2 citations) DOI - 10.5281/zenodo.10790873
BioProject (3)
- (2 citations) BioProject - PRJEB496
- (2 citations) BioProject - PRJEB6697
- (2 citations) BioProject - PRJNA878701
Similar Articles
To arrive at the top five similar articles we use a word-weighted algorithm to compare words from the Title and Abstract of each citation.
Negligible risk of zoonotic anisakid nematodes in farmed fish from European mariculture, 2016 to 2018.
Euro Surveill, 26(2), 01 Jan 2021
Cited by: 8 articles | PMID: 33446302 | PMCID: PMC7809721
Parasites in fishery products - Laboratorial and educational strategies to control.
Exp Parasitol, 211:107865, 23 Feb 2020
Cited by: 4 articles | PMID: 32101764
Validation of the TrichinEasy® digestion system for the detection of Anisakidae larvae in fish products.
Acta Parasitol, 61(2):369-375, 01 Mar 2016
Cited by: 4 articles | PMID: 27078661
Vaccines for fish in aquaculture.
Expert Rev Vaccines, 4(1):89-101, 01 Feb 2005
Cited by: 237 articles | PMID: 15757476
Review