Abstract
Free full text

Sustained release of 5-aminosalicylic acid from azoreductase-responsive polymeric prodrugs for prolonged colon-targeted colitis therapy
Abstract
Ulcerative colitis (UC) is a challenging inflammatory gastrointestinal disorder, whose therapies encounter limitations in overcoming insufficient colonic retention and rapid systemic clearance. In this study, we report an innovative polymeric prodrug nanoformulation for targeted UC treatment through sustained 5-aminosalicylic acid (5-ASA) delivery. Amphiphilic polymer-based 13.5 nm micelles were engineered to incorporate azo-linked 5-ASA prodrug motifs, enabling cleavage via colonic azoreductases. In vitro, micelles exhibited excellent stability under gastric/intestinal conditions while demonstrating controlled 5-ASA release over 24 h in colonic fluids. Orally administered micelles revealed prolonged 24-h retention and a high accumulation within inflamed murine colonic tissue. At an approximately 60% dose reduction from those most advanced recent studies, the platform halted DSS colitis progression and outperformed standard 5-ASA therapy through a 77–97% suppression of inflammatory markers. Histological analysis confirmed intact colon morphology and restored barrier protein expression. This integrated prodrug nanoformulation addresses limitations in colon-targeted UC therapy through localized bioactivation and tailored pharmacokinetics, suggesting the potential of nanotechnology-guided precision delivery to transform disease management.
Supplementary Information
The online version contains supplementary material available at 10.1186/s12951-024-02724-w.
Introduction
Oral drug administration, one of the most common routes for therapeutic delivery, is widely favored for its convenience, non-invasiveness, and patient compliance [1]. However, the oral route also presents several challenges that can impede the effectiveness of treatment [2]. The primary concerns is the variable bioavailability of drugs, influenced by factors such as gastrointestinal pH, enzymatic degradation. Furthermore, the first-pass metabolism in the liver can significantly alter the concentration of the drug located at the desired position, which lead to a higher dose to achieve the desired therapeutic effect, and in turn increase the risk of side effects [3]. Thus, taking full advantage of these characters is crucial in designing effective oral drug delivery systems, particularly when addressing chronic and localized conditions.
The advent of nanotechnology in drug delivery has revolutionized the field of oral medications [4]. Nanomedicines offer numerous advantages, including enhanced solubility, stability, and the potential for targeted delivery to specific sites within the body. However, despite these advancements, oral nanomedicines have their own set of challenges, such as premature release and robust release at target site [5]. Oral prodrug is another method also used to enhance the bioavailability and efficacy of active pharmaceutical ingredients [6]. Prodrugs are chemically modified, inactive derivatives of active drugs, designed to undergo transformation within the body to release the active drug. This strategy often addresses the limitations of direct drug delivery by improving solubility and stability. However, a major concern is ensuring that the conversion to the active drug occurs precisely at the targeted site [7]. In conditions where specific localization is required, achieving site-specific activation of prodrugs can be particularly challenging. Furthermore, the many enzymes in the gastrointestinal environment can activate the prodrug with robust kinetics, thus reducing the therapeutic efficiency. Thus, while oral prodrugs offer a promising avenue for improved drug delivery, addressing their inherent challenges is crucial for developing effective treatments, particularly for targeted therapy in diseases.
Ulcerative colitis (UC) is a chronic inflammatory bowel disease whose treatments primarily rely on oral-based small-molecule anti-inflammatory drugs, such as 5-aminosalicylic acid (5-ASA), aiming to induce and maintain remission and manage symptoms during flare-ups [8–12]. However, as an oral drug, its effectiveness for UC is often hampered by the challenges mentioned above [13–15]. Moreover, many patients with UC experience periods of increased intestinal motility and promoted emptying, which can further complicate the retention and effectiveness of orally administered therapies [16–18]. Thus, strategies to overcome retention and specificity would great benefit the therapeutic of UC.
Nanoparticles combining prodrugs have been used for 5-ASA oral delivery to treat UC [19–25]. These systems, designed to extend the drug’s presence in the colon, often involve surface modifications such as imparting a negative charge to target inflamed colonic mucosa selectively [26–28]. However, rapid drug release and subsequent clearance from the colon remain significant limitations of these systems, even with those who were linked through covalent bonds [29–31]. As a result, drugs get cleared before they have a chance to exert their therapeutic effect. Zero-order, sustained release allows constant therapeutic levels to be maintained, overwhelming drug-delivery systems with robust releasing [32–37]. Hence, the desired drug delivery nanoparticle designs should be equipped with not only targeted retention, but also extended slow-release profile.
Our laboratory has developed a series of polymers for different applications [38–45]. Very interestingly, we found that the molecules trapped inside the hyperbranched structure of polymer can alternate its reaction kinetic towards the outside environment [46]. Thus, in this study our investigative efforts focused on the development of a polymeric prodrug nanoformulation, characterized by its structural simplicity, which affords targeted and sustained release of therapeutic agents within the colon (Fig. 1a). This nanoformulation prodrug design involves an amphiphilic polymer-based micellar architecture, yielding a delivery vehicle of nanosized micelles to avoid premature drug absorption. The surface charge of these micelles has been engineered to possess a negative charge, thereby enhancing affinity for the positively charged inflamed colonic tissue of UC and ensuring preferential localization [47]. 5-ASA is conjugated via an azo linkage, which is specifically cleavable within the colonic environment by azoreductase enzymes [48–51]. The polymer’s side chains strategically hinder the prodrug, moderating its release and enabling a controlled, extended drug release profile. This design effectively tempers the rapid cleavage of azo-linked molecules, allowing for an extended drug release timeline that surpasses previous research in both retention and drug release duration. Such a desired drug delivery behavior led to a decrease of 5-ASA dosage dramatically but with a commendable therapeutic effect, offering a novel drug delivery system that promises to improve patient outcomes by addressing the limitations of conventional treatments.
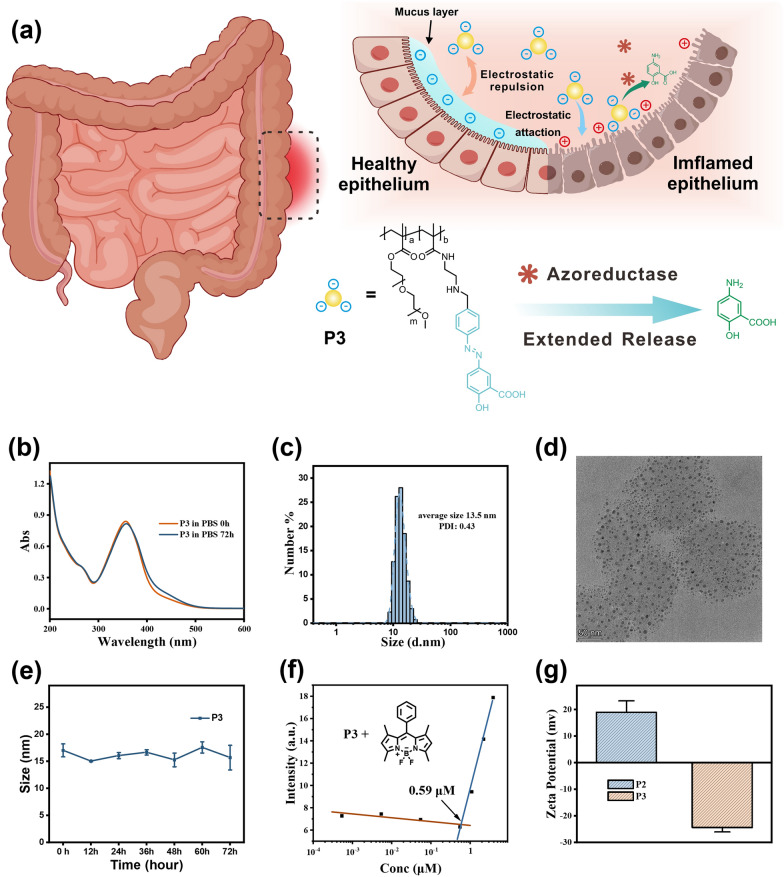
a Proposed mechanism of P3 to treat UC. b Temporal dependence of UV–Vis absorption profile for P3 (0.5 mg
Results and discussion
Synthesis and characterization
The synthesis of the 5-ASA polymeric prodrug (P3) involved the incorporation of three essential components: a hydrophobic poly(methacrylate) backbone, hydrophilic side chain, and azo-linked 5-ASA prodrug units. This was achieved through a three-step procedure, as described in Scheme S1. In the first step, a radical copolymerization reaction was carried out using polyethylene glycol methacrylate (PEGMA, Mn = 950 g
The UV–vis absorption measurements demonstrated the presence of an absorption peak at 370 nm in P3, which was identical to the peak observed in compound 3 (Fig. 1b and S1b). By utilizing UV absorption measurements and the molar extinction coefficient of compound 3 (22,800
In vitro drug release
The release profile of 5-ASA from a model azo prodrug was first investigated in vitro using compound 3. Compound 3 contains an azo linker attaching a 4-aminobenzaldehyde group to 5-ASA, providing a representative small molecule mimic of the polymeric azo prodrug. Analysis of compound 3 by HPLC revealed a peak at a retention time of 4.2 min under the elution condition (Fig. 2a). To simulate colonic azoreductase activity, compound 3 (100

a HPLC chromatograms of model compound 3 (0.1 mM) after reaction with SDT (2.5 mM) for 0.5 h. C18 chromatography performed using 10–100% acetonitrile: water gradient mobile phase. b UV–vis absorption spectra of P3 (0.05 mg
Similar release behavior was observed for polymeric prodrug P3. The original absorption peak at 370 nm decreased upon treatment with different equivalents of SDT in double distilled water for 0.5 h (Fig. 2b). Direct MS analysis of the reaction mixture showed the appearance of a peak at m/z 152.1 after 0.5 h, confirming 5-ASA release (Fig. S2). In simulated gastric fluid (SGF) and simulated intestinal fluid (SIF), P3 micelles remained intact, and no 5-ASA was detected over 3 h by MS (Fig. S2). DLS measurements were consistent with the spectrophotometric and MS data. The mean diameter of P3 micelles remained around 12 nm after incubation in SGF, while 10 nm in SIF, for 24 h each. In contrast, a rapid decrease in size to 5 nm was observed within the first 4 h incubation in SIF containing 25 eq SDT.
After the confirmation of the 5-ASA release mechanism, the in vitro release kinetics from polymeric prodrug P3 versus small molecule sulfasalazine (SSZ), a commercially available azo-based prodrug of 5-ASA, were investigated by HPLC. P3 and SSZ were firstly incubated in gastric contents for 4 h and small intestinal contents for 12 h, representing typical maximum transit times in mice, [56–59] before injected into the size exclusive column. HPLC analysis of P3 conducted under gastric contents and small intestinal contents did not reveal any detectable presence of 5-ASA, as exemplified in Figures S3a and 2d, indicating the excellent stability of P3 in these environments. In contrast, SSZ exhibited no 5-ASA release in gastric contents (Figure S3b) while a partial release under intestinal conditions, with a small peak emerging at the retention time of standard 5-ASA indicating approximately 25% release in 12 h (Figs. 2g and S4a). This release behavior in the small intestine decreases its potential therapeutic effect.
The transformation occurred when P3 was exposed to the colon contents, where the presence of azoreductase activity triggered the selective release of 5-ASA. HPLC quantification of the released 5-ASA over a 24-hour incubation period, representing typical transit time, [60–62] unveiled a controlled and sustained release profile, with approximately 60.0% of the loaded 5-ASA being liberated at a sustained rate(Figs. 2e and f). In stark contrast, SSZ exhibited a significantly different release profile. Within the colon, SSZ released the majority of its 5-ASA payload (82.9%) within a mere 2-hour period and minimal further release beyond 2 h (Figs. 2h, i and S4b), as opposed to the controlled and sustained release observed with P3. Such a robust release profile may not guarantee the maintenance of effective 5-ASA concentrations afterward, potentially resulting in a diminished therapeutic effect. The area under the curve for 5-ASA concentration over time after 2 h was 6.5-fold higher for P3 compared to SSZ, indicating substantially enhanced long-term drug exposure. P3’s sustained release kinetics closely simulate the colonic environment, confirming specificity of sustained 5-ASA release in response to azoreductase. Compared to SSZ’s initial burst release, P3’s sustained release profile enables maintenance of therapeutic 5-ASA concentrations in inflamed colonic tissue for a prolonged duration. Such data prove that our novel drug delivery system presents a promising approach to controlled delivery to optimize ulcerative colitis therapy, addressing the limitations associated with conventional treatments with small molecules, like SSZ.
Retention in colon
Prior to the in vivo test, the toxicity of P2 and P3 was first evaluated in vitro using Caco-2 cells and RAW 264.7 macrophages (Figure S5). Cells were incubated with varying concentrations of polymers P2 and P3 for 24 h before assessing cell viability using the CCK-8 assay. Both polymers exhibited minimal toxicity in either cell line up to the maximum tested concentration of 5 mg
To further validate the electrostatic targeting capabilities of the polymeric prodrug nanoparticles, in vitro adhesion studies were performed using surfaces coated with mucin, a major component of colonic mucus. Freshly cleaved mica sheets were coated with a 1 mg
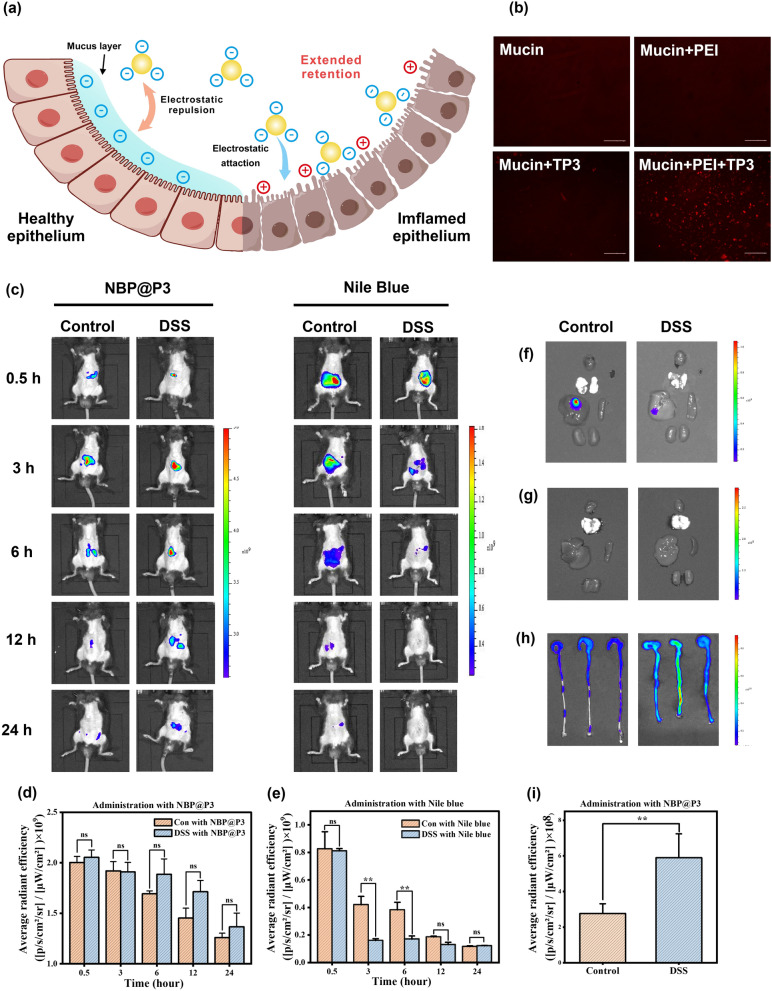
a Proposed mechanism of inflamed colon-targeted delivery for P3. The negative surface charge minimizes mucoadhesion in healthy colon. Electrostatic adhesion to positively charged inflamed colonic tissue enables site-specific retention. Sustained colonic release improves local efficacy. b Evaluation of polymeric prodrug nanoparticle adhesion to surfaces with defined charge. Fluorescence microscopy images showing minimal adhesion of TP3 nanoparticles on mucin protein coatings while increased TP3 adhesion when mucin coatings are treated with the cationic polymer polyetherimide to provide a positively charged surface. Scale bar: 200
In vivo evaluation of P3 and a small molecule dye, Nile Blue (NB), was performed to assess their respective retention profiles in healthy and DSS-induced colitic mice using comprehensive whole-body fluorescence imaging (as depicted in Fig. 3c). Due to the fluorescent quenching effect of azo compound towards most fluorophores, TP3 is not suitable for whole body imaging in this case [63]. Thus we applied a NIR BODIPY (NBP, structure and spectra shown in Figure S1 and S7) to be encapsulated in P3 micelle for tracking [64]. The investigation commenced immediately following oral gavage and entailed tracking at time points of 0.5, 3, 6, 12 and 24 h post-administration. At 0.5 h, both P3 encapsulating NBP (NBP@P3) and NB exhibited pronounced signals within the upper bally region, indicative of their initial gastric retention. By the 3-hour mark, NB fluorescence in DSS-induced colitic mice exhibited a rapid decline, primarily attributed to its swift evacuation from the gastrointestinal tract, while in healthy mice, residual signals had a moderate decrease. As the evaluation continued, the 6-hour time point revealed a remarkable reduction of NB fluorescence by approximately 77% in healthy mice, attributable to processes involving absorption and subsequent systemic clearance. In contrast, within the DSS-induced colitic mice, NB fluorescence became nearly undetectable, aligning with the notable lack of retention.
In sharp contrast to the behavior of NB, NBP@P3 demonstrated a strikingly different retention profile (Fig. 3c–e). At the 6-hour interval, NBP@P3 exhibited intense fluorescence specifically within the lower belly region of DSS-induced colitic mice. This observation underscores the exceptional ability of the polymeric prodrug to achieve prolonged retention and targeted delivery to inflamed colonic tissue. Subsequent measurements at the 12-hour time point unveiled a distinctive outcome: NB fluorescence remained undetectable in both healthy and DSS-induced colitic mice, signifying complete clearance from the system. In contrast, NBP@P3 fluorescence, while exhibiting a reduction compared to the 6-hour signals, remained readily detectable within the colons of both groups. Ultimately, at the 24-hour time point, NBP@P3 fluorescence persisted at detectable levels in DSS-induced colitic mice, further reinforcing its capacity for prolonged retention and targeted delivery to inflamed colonic tissue. In contrast, within the healthy mice, NBP@P3 exhibited a comparable reduction in fluorescence levels, highlighting the similarities in their clearance rates under different physiological conditions. Considering the quicker clearance rates in colitic mice, P3 demonstrated a distinct advantage in terms of retention [65].
Fluorescence imaging of excised organs unveiled distinct biodistribution patterns, providing further insights into the exceptional performance of NBP@P3 in targeted colitis therapy. In contrast to NB-treated mice, where fluorescence signals primarily localized in the livers at the 3-hour mark (Fig. 3f), NBP@P3 exhibited an entirely different distribution profile. Notably, NBP@P3 displayed negligible fluorescence in critical organs such as the heart, liver, spleen, and kidneys (Fig. 3g). The nanoparticle formulation NBP@P3 exhibited remarkable retention and colon-targeting capabilities, evidenced by significantly higher fluorescence intensities observed in the colons of DSS-induced colitic mice compared to their healthy counterparts at the 24-hour time point (Fig. (Fig.3h3h and and3i).3i). This preferential accumulation can be attributed to the electrostatic interactions facilitated by the anionic surface charge of NBP@P3. Notably, the accelerated gastrointestinal transit and emptying typically observed in UC mice would be expected to reduce the retention time of orally administered agents. However, the enhanced retention of NBP@P3 in the DSS-induced colitic mice suggests a prolonged localization within the inflamed colonic tissue. Such enhanced retention is attributed to the electrostatic interactions arising from NBP@P3’s negative surface charge, facilitating its specific adhesion to the positively charged colonic mucosa in the context of colitis.
Therapeutic efficacy
The therapeutic efficacy of P3 was evaluated in vivo using a DSS-induced mouse model of colitis, [66] along with controls including precursor polymer P2 and a physical mixture of P2 + free 5-ASA. Mice were provided 2.5% DSS in drinking water for 7 days to induce colonic inflammation (Fig. 4a). They were then treated by daily oral gavage for 7 days with either PBS, 5-ASA (30 mg
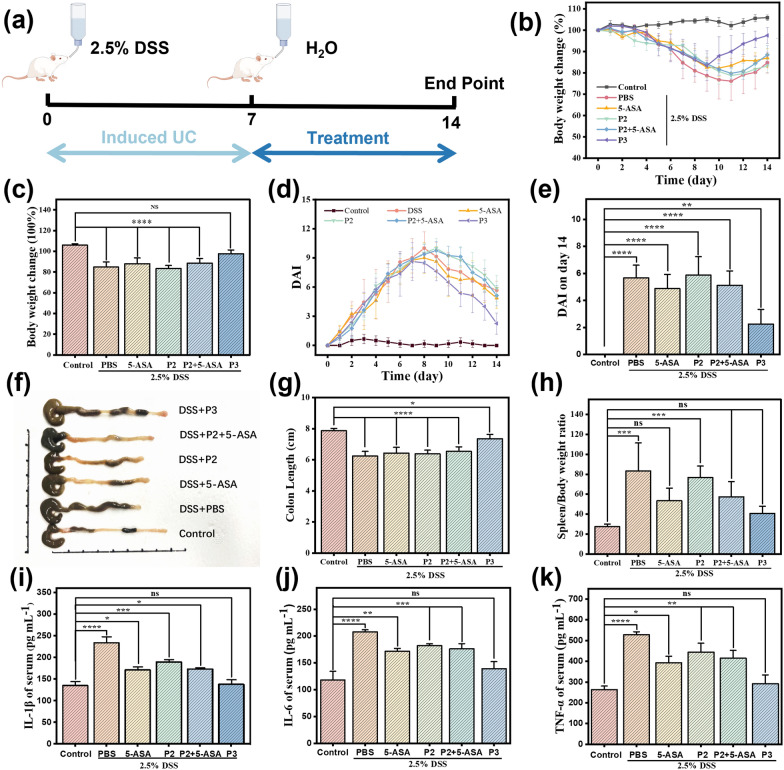
Therapeutic efficacy of P3 compared to controls against DSS-colitis in mice. a Schematic of the experimental design. Mice were randomized into 6 groups (Control: PBS+normal water, PBS+DSS water, 5-ASA+DSS water, P2+DSS water, P2+5-ASA+ DSS water, P3+DSS water). Colitis was induced with 2.5% DSS drinking water for 7 days followed by 7-day treatment with oral gavage of PBS, 5-ASA, P2, P2+5-ASA, or equivalent 5-ASA dose of P3. b Body weight changes from day 1 to day 14 and c the statistical chart of weight change on day 14. d DAI changes from day 0 to day 14 and e the statistical chart of the disease index on day 14. f Schematic diagram of colonic tissue in each group and g the statistical chart Colon length on the day 14. h Spleen/Body weight ratio. i IL-1
A similar trend was seen in changes of the Disease Activity Index (DAI), an integration of weight loss, stool consistency, and fecal blood [67, 68]. In PBS and P2 groups, DAI peaked at 10±1.7 and 10±1 on days 8–9 before recovering slightly (Fig. 4d). However, DAI remained elevated at 5.67±0.94 and 5.87±1.36 on day 14 (both p < 0.0001 vs. control) (Fig. 4e). 5-ASA and P2+5-ASA groups followed similar trends, peaking at day 8-9 and dropping to 4.88±1.05 and 5.13±1.05 by day 14 (p < 0.0001 vs. control). In contrast, the DAI of the P3 treatment group began to decline on day 9 of the experiment, showing an earlier recovery, and dropped to 2.25±1.09 on the fourteenth day of the experiment, which was significantly lower than that of the other groups (p < 0.01). By integrating multiple metrics of disease progression, the DAI results further confirm the enhanced therapeutic efficacy of P3.
In addition to reduced weight loss, P3 treatment markedly attenuated DSS-induced colonic shortening, a marker of inflammation and tissue damage (Fig. 4f and g) [69]. On day 14, the average colon length of PBS and P2-treated mice were 6.23 cm and 6.38 cm respectively, compared to 7.87 cm for healthy controls. 5-ASA and P2+5-ASA partially preserved colon length to 6.43 cm and 6.54 cm respectively (P < 0.0001 vs. control), while P3-treatment resulted in a length of 7.35 cm (P < 0.05 vs. control), very close to healthy mice. The spleen/body weight ratio was also calculated as a metric of systemic inflammation. In healthy mice, the average spleen/body weight ratio was 27.04 (Fig. 4h). This was significantly increased to 83.27 in PBS-treated colitic mice, indicative of splenomegaly. Treatment with standard 5-ASA moderately reduced this ratio to 53.35, while the polymeric prodrug P3 decreased it back to 40.65, similar to that of healthy mice. Mice receiving the precursor polymer P2 alone without 5-ASA conjugation exhibited a ratio of 76.73, no different than PBS controls. The combination of P2+5-ASA resulted in an intermediate ratio of 57.38, comparable to 5-ASA-treated mice but higher than P3-treated ones.
Similarly, serum levels of pro-inflammatory cytokines IL-1
These results suggest that the conjugation of 5-ASA to the polymeric carrier P3 as an azoreductase-activated prodrug resulted in the greatest suppression of systemic and intestinal inflammatory markers as well as superior therapeutic efficacy in DSS-induced colitis. The polymer alone did not confer bioactivity, and the physical combination with 5-ASA had no enhancement compared to 5-ASA alone, highlighting the unique benefits of the prodrug nanoformulation. It is noteworthy to mention that this platform utilized a notably reduced dosage of 5-ASA at 30 mg
Histological analysis
H&E staining was performed on colon sections to assess tissue morphology and inflammation (Fig. 5 and S8-S10). Healthy control mice displayed intact colonic epithelium with abundant goblet cells and no immune cell infiltration. Mice receiving only DSS showed extensive disruption of epithelial architecture, massive loss of goblet cells, submucosal edema, and massive infiltration of immune cells including neutrophils and macrophages. Mice treated with just the precursor polymer P2 after DSS induction showed similar histological features as the DSS alone group, indicating the polymer carrier itself did not confer bioactivity. DSS mice receiving 5-ASA therapy displayed moderate improvement in tissue integrity compared to DSS controls, with some residual goblet cell loss and immune cell infiltration. The combination therapy of P2+5-ASA resulted in an intermediate degree of structural damage and inflammation. In contrast, mice treated with P3 showed remarkably intact colonic morphology comparable to healthy controls, with an intact epithelial barrier, abundant goblet cells, and minimal immune cell infiltration. P3 therapy effectively preserved the colonic epithelial architecture and suppressed inflammation, highlighting its significant therapeutic efficacy compared to standard 5-ASA treatment.
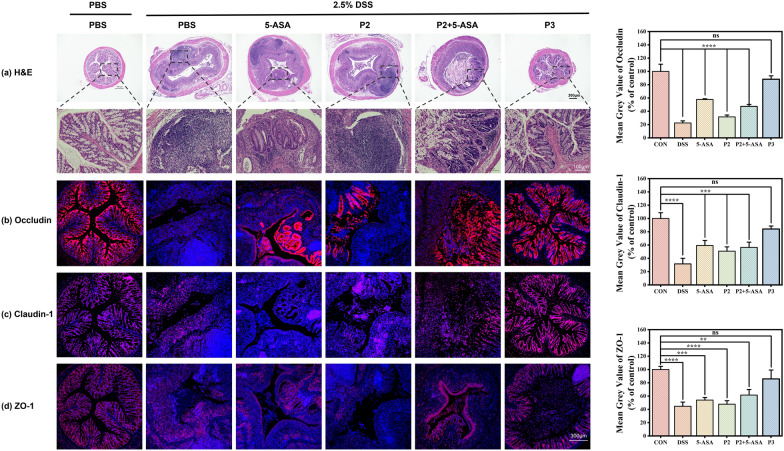
Representative micrographs and quantitative analysis a H&E, b Occludin, c Claudin-1 and d ZO-1 stained colon sections from mice in the various treatment groups. After the 7-day treatment period, colon samples were harvested, fixed in 42% paraformaldehyde, and embedded in paraffin. Sections were cut to 4
To further evaluate the efficacy of polymeric prodrug P3 at a molecular level, immunofluorescence staining was performed to visualize the expression and localization of key tight junction proteins that maintain colonic epithelial barrier integrity (Figs. 5b–d, S7-S9). These included ZO-1, Occludin, and Claudin-1 in colon sections from healthy mice or DSS-colitic mice treated with various regimens described previously. Confocal microscopy revealed intense staining and continuous membrane localization of all three proteins in a healthy control colon. In contrast, mice with untreated colitis showed severely disrupted staining and gaps between epithelial cells, indicating loss of barrier function. Notably, mice receiving P3 therapy displayed a remarkable restoration of tight junction protein expression, organization, and localization profiles matching healthy control. In contrast, only partial recovery was observed after treatment with standard 5-ASA or P2+5-ASA combination, while precursor polymer P2 alone showed no improvement versus untreated colitic mice. Taken together with the H&E analysis, these results confirm that sustained targeted delivery of 5-ASA mediated by the polymeric prodrug nanoformulation effectively preserves colonic barrier integrity on histological and molecular levels in this UC model. The ability to restore tight junction protein function highlights its potential to improve clinical outcomes.
Biocompatibility
To evaluate the potential toxicity of the lead polymeric prodrug P3, major organs including the heart, liver, spleen, lungs, and kidney were harvested from colitic mice treated with P3 and compared to controls (Figure S11). Microscopic examination of H &E stained tissue sections showed no observable differences in morphology or presence of pathologic signs between P3-treated and control mice in any of the analyzed organs. Heart sections displayed normal cardiomyocyte architecture with no inflammatory cell infiltration. Hepatocytes, splenocytes, pulmonary alveoli, renal tubules, and all appeared intact without necrosis or apoptosis. Collectively, these results demonstrate that systemic administration of polymeric prodrug P3 does not induce toxicity or physiologic changes in major organs. The favorable safety profile and biocompatibility of P3 support its potential translation for colitis therapy.
Methods
Chemicals were purchased from commercial sources and used as received.
Synthesis of 3
4-Aminobenzaldehyde and sodium nitrite were dissolved in 50 mL of water in a molar ratio of 1:1.3. To this mixture, concentrated hydrochloric acid in a molar ratio of 1:4 was added. The reaction mixture was stirred at
Synthesis of P1
Polyethylene glycol methyl ether methacrylate (PEGMA, Mn = 950 g
Synthesis of P2
Polymer P1 was stirred in a 4 M solution of HCl in dioxane at room temperature for 2 h. Subsequently, dioxane and HCl were removed from the reaction mixture by vacuum distillation. The resulting polymer was then dried overnight in a vacuum oven to obtain P2 as a colorless, transparent, viscous liquid with a yield of 99%.
Synthesis of P3
Polymer P2 and compound 3 were dissolved in methanol at a molar ratio of 1 (amount of amine): 2. After stirring at room temperature for 1 h, a molar equivalent of sodium cyanoborohydride was added, and the mixture was stirred overnight at room temperature. The methanol in the reaction mixture was then removed by vacuum distillation, and the resulting product was purified using LH-20 gel column chromatography (methanol/dichloromethane = 1:1, v/v). The purified polymer was designated as P3 and appeared as a yellow solid with a yield of 73.6%.
Synthesis of TP3
P3 (50 mg) was dissolved in 5 mL of potassium carbonate buffer (pH 9). TRITC was dissolved in DMSO at 20 mM and added to the polymer solution. The reaction was protected from light and allowed to proceed overnight at room temperature with stirring. The labeled polymer was purified by LH-20 gel column chromatography (methanol/dichloromethane = 1:1, v/v) to obtain TP3 as a yellow solid.
Synthesis of NBP. The synthesis of NBP followed a previous literature [64]. In the presence of piperidine (1.0 mL) and acetic acid (1.0 mL), a solution of BODIPY (1 mmol) and p-tolualdehyde (3 mmol) in toluene (15 mL) was heated at reflux by using a three-necked flask equipped with a Dean-Stark apparatus. The resulting residue was purified by column chromatography on neutral silica gel (hexane/EtOAc = 4 : 1) to give NBP in 76% yield (0.76 mmol).
Preparation of nanoparticle dispersions
A suitable volume of methanol solutions containing P2, P3 or TP3 (100 mg
TEM
0.2 mg
DLS and Zeta potential
0.5 mg
CMC
10
HPLC analysis of compound 3 reacting with SDT
Model compound 3 (0.1 mM) was incubated with sodium dithionite (SDT, 2.5 mM) for 30 min at
HPLC analysis of 5-ASA release from P3
Gastric contents, SI contents and colonic contents were collected from healthy mice. Following the euthanization of mice with CO2 gas, the gastrointestinal contents were gathered and subsequently mixed with PBS to create a 10% (w/v) suspension. P3 was incubated with intestinal contents for 2, 8, and 12 h and colon contents for different time periods at
HPLC analysis of 5-ASA release from SSZ
Gastric contents, SI contents and colonic contents were collected from healthy mice following the same procedure as described above. SSZ was incubated with intestinal contents for 2, 4, 8, and 12 h and colon contents for different time periods at
MS analysis of 5-ASA release from P3
P3 (0.05 mg
Cell cytotoxic assays
The cell viability was evaluated using the standard Cell Counting Kit-8 (CCK-8) method. Caco-2 or RAW 264.7 cells (
DSS induced colitis mouse model
In vivo studies were performed on male C57BL/6J mice (7 weeks old, 22–24 g), provided by Zhejiang Vital River Experimental Animal Technology Co. LTD). Mice received the housing process based on normal condition and were given adlibitum distilled water and food. The UC mice model was established by administering 2.5% (w/v) dextran sulfate sodium (DSS, 36–50 kDa) in drinking water for 7 days and induced colonic inflammation. The appearance of weight loss, loose stool, diarrhea, bloody stool or fecal occult blood, and ulcers were regarded as signs of successful modeling.
Retention of NBP@P3 and NB in vivo
The retention time of NBP@P3 and NB was assessed using whole-body fluorescence imaging experiment. The procedure involved the oral administration of NBP@P3 (30 mg
Treatment of the UC mice
The mice were separated into six groups, namely, healthy control group, DSS model group, 5-ASA-treated DSS group, P2-treated DSS group, 5-ASA and P2-treated DSS group and P3-treated DSS group. Control healthy mice received normal drinking water only. The colitic mice received daily oral gavage for 7 days with 200
Weight loss and Disease Activity Index (DAI)
Body weight loss and DAI were monitored daily as indicators of colitis progression. Body weight was measured daily and percent change from day 0 was calculated. Stool consistency and fecal occult blood were evaluated using the following scoring system: Occult blood - 0, no blood; 1, microscopic bleeding; 2, mild bleeding; 3, obvious bleeding; 4, gross bleeding. Stool consistency - 0, normal; 1, loose stool; 2, mild diarrhea; 3, diarrhea; 4, severe diarrhea. DAI was calculated by combining the occult blood score, stool consistency score, and percent body weight change score, which was assigned as follows: 0, none; 1, 1–5% loss; 2, 5–10% loss; 3, 10–20% loss; 4, >20% loss. Disease activity index (DAI) was obtained based on the summation of stool consistency state (0–4), fecal bleeding (0–4), and body weight loss (0–4).
Spleen index
Spleens were harvested immediately after euthanasia. Wet weight of each spleen was measured, and the spleen index was calculated as: spleen weight (mg) / body weight (10 g). Higher spleen index indicates greater systemic inflammation.
Cytokine analysis
On day 14, whole blood was collected via eye bleed under isoflurane anesthesia. Blood was allowed to clot at room temperature for 30 min before centrifuging at 2500 rpm for 20 min at
Histological analysis
After euthanasia, the colon from cecum to anus was removed and length measured. A 0.5 cm portion of distal colon was fixed in 4% paraformaldehyde for 24 h followed by paraffin embedding and sectioning into 4 μm slices. Hematoxylin and eosin (H&E) staining was performed to assess tissue morphology. The images were taken using an upright microscope. Tight junction proteins ZO-1, Claudin-1, and Occludin were imaged by immunofluorescence staining. The images were taken using fluorescent microscope and Confocal Laser Scanning Microscopy (CLSM).
Biocompatibility
For biocompatibility assessment, heart, liver, spleen, lung and kidney were harvested and fixed in 4% paraformaldehyde. Sections were paraffin-embedded, sliced to 4 μm, and H &E stained to evaluate tissue morphology and identify any signs of toxicity.
Conclusion
In summary, this study represents a significant advancement in the realm of UC therapy through the development of a novel drug delivery system. Our research focuses on the rational design of amphiphilic polymeric prodrugs, specifically tailored to optimize 5-ASA release kinetics for colitis therapy. These prodrugs self-assemble into small micellar nanoparticles, offering several advantages: (1) The incorporation of enzyme-cleavable 5-ASA prodrug units in the micelle corona enables specific drug release at the inflamed colonic site while avoiding systemic exposure. (2) The formation of micelles as well as negatively charged surface promotes the enhancing adhesion to diseased mucosa. (3) Additionally, the architecture of the polymeric design allows for controlled modulation of release kinetics.
Both in vitro and in vivo experiments provide compelling evidence of the efficacy of this drug delivery system. In vitro studies demonstrated selective 5-ASA release in the presence of azoreductase activity and validated stable circulation with negligible premature release followed by sustained colonic delivery, in stark contrast to burst release from sulfasalazine prodrugs. Furthermore, in vivo studies using a DSS-induced mouse model of colitis demonstrated that our negatively charged P3 formulation, with a reduced dosage of 5-ASA at just 30 mg
In conclusion, our polymeric prodrug approach offers a promising avenue for enhanced UC therapy. By addressing the limitations of conventional treatments, such as poor bioavailability, rapid clearance and undesired release profile, our drug delivery system has the potential to significantly improve patient outcomes and quality of life.
Acknowledgements
The authors would like to thank Rongqi Xia from Shiyanjia Lab (www.shiyanjia.com) for the HRMS analysis. Figs. 1a and 3a were created using materials sourced from Figdraw (https://www.figdraw.com).
Author contributions
Sicheng Tang and Wenchao Wang contributed equally to this work. The manuscript was written through contributions of all authors. All authors have given approval to the final version of the manuscript.
Funding
This work was financially supported by startup funding from the Wenzhou Institute of UCAS (WIUCASQD2021046), Wenzhou Science and Technology Project (2023Y0847) and Zhejiang Provincial Natural Science Foundation of China (LQ24H120002).
Declarations
All animal experiments conducted in this study were performed in strict accordance with the guidelines and regulations set forth by the Wenzhou Institute, UCAS. This study was approved by the WIUCAS Animal Ethics Committee, with the approval certificate number WIUCAS23033101.
The authors declare no competing interest.
Footnotes
Publisher's Note
Springer Nature remains neutral with regard to jurisdictional claims in published maps and institutional affiliations.
Sicheng Tang and Wenchao Wang contributed equally to this work.
Contributor Information
Sicheng Tang, Email: nc.ca.sacu@gnatgnehcis.
Limeng Zhu, Email: nc.ca.sacu@gnemiluhz.
Wujun Geng, Email: moc.621@nujuwgneg.
References


Articles from Journal of Nanobiotechnology are provided here courtesy of BMC
Similar Articles
To arrive at the top five similar articles we use a word-weighted algorithm to compare words from the Title and Abstract of each citation.
Discovery and preclinical development of a novel prodrug conjugate of mesalamine with eicosapentaenoic acid and caprylic acid for the treatment of inflammatory bowel diseases.
Int Immunopharmacol, 40:443-451, 04 Oct 2016
Cited by: 3 articles | PMID: 27716592
Evaluation of 5-aminosalicyltaurine as a colon-specific prodrug of 5-aminosalicylic acid for treatment of experimental colitis.
Eur J Pharm Sci, 28(1-2):26-33, 07 Feb 2006
Cited by: 22 articles | PMID: 16455235
5-Aminosalicylic Acid Azo-Linked to Procainamide Acts as an Anticolitic Mutual Prodrug via Additive Inhibition of Nuclear Factor kappaB.
Mol Pharm, 13(6):2126-2135, 05 May 2016
Cited by: 7 articles | PMID: 27112518
Pharmaceutical approaches to colon targeted drug delivery systems.
J Pharm Pharm Sci, 6(1):33-66, 01 Jan 2003
Cited by: 164 articles | PMID: 12753729
Review
Funding
Funders who supported this work.
Science and Technology Plan Project of Wenzhou Municipality (1)
Grant ID: 2023Y0847
University of Chinese Academy of Sciences, Wenzhou Institute (1)
Grant ID: WIUCASQD2021046
Zhejiang Provincial Natural Science Foundation of China (1)
Grant ID: LQ24H120002