Abstract
Free full text

Evidence for Diversifying Selection at the Pyoverdine Locus of Pseudomonas aeruginosa
Abstract
Pyoverdine is the primary siderophore of the gram-negative bacterium Pseudomonas aeruginosa. The pyoverdine region was recently identified as the most divergent locus alignable between strains in the P. aeruginosa genome. Here we report the nucleotide sequence and analysis of more than 50 kb in the pyoverdine region from nine strains of P. aeruginosa. There are three divergent sequence types in the pyoverdine region, which correspond to the three structural types of pyoverdine. The pyoverdine outer membrane receptor fpvA may be driving diversity at the locus: it is the most divergent alignable gene in the region, is the only gene that showed substantial intratype variation that did not appear to be generated by recombination, and shows evidence of positive selection. The hypothetical membrane protein PA2403 also shows evidence of positive selection; residues on one side of the membrane after protein folding are under positive selection. R′, previously identified as a type IV strain, is clearly derived from a type III strain via a 3.4-kb deletion which removes one amino acid from the pyoverdine side chain peptide. This deletion represents a natural modification of the product of a nonribosomal peptide synthetase enzyme, whose consequences are predictive from the DNA sequence. There is also linkage disequilibrium between the pyoverdine region and pvdY, a pyoverdine gene separated by 30 kb from the pyoverdine region. The pyoverdine region shows evidence of horizontal transfer; we propose that some alleles in the region were introduced from other soil bacteria and have been subsequently maintained by diversifying selection.
Pseudomonas aeruginosa is a gram-negative bacterium commonly isolated from fresh water and soil. The opportunistic pathogen causes a variety of human infections, including infections of the ear canal, lung, eye, and urinary tract. P. aeruginosa is particularly dangerous for immunocompromised patients such as those with human immunodeficiency virus, cancer, or severe burn wounds. Most cystic fibrosis patients have pulmonary P. aeruginosa infections for the majority of their lives. Infection also occurs in many other organisms, including insects (9), nematodes (16), plants (48), and amoebas (52).
Iron is necessary for the growth and survival of almost all organisms, with the exception of Lactobacillus species and some intracellular parasites (3). Iron is incorporated into numerous protein moieties such as iron-sulfur clusters and participates in many cellular redox reactions. Although abundant on the surface of the Earth, iron is not readily accessible because it rapidly oxidizes to insoluble Fe3+ compounds under aerobic conditions. Bacteria obtain iron by a variety of mechanisms, including secretion and subsequent uptake of ferrisiderophores, direct uptake of ferrous iron, uptake of host iron-binding molecules, and proteolytic destruction of host proteins with subsequent iron scavenging (54). Mechanisms for iron acquisition via siderophores are diverse; at least 500 chemically distinct siderophores are known (69). Although almost all organisms require iron, excess iron is highly toxic in aerobic environments, particularly because of the production of hydroxyl radicals in the Fenton reaction.
Pyoverdines are siderophores made by members of the genus Pseudomonas (10, 37). Pyoverdine is the primary siderophore of P. aeruginosa, and pyoverdine mutants have attenuated virulence in multiple infection models (39, 66). Each strain of P. aeruginosa makes one of three pyoverdine types, each type having a distinct peptide side chain that is synthesized nonribosomally (40). The pyoverdine receptor is also type specific, transporting only its corresponding pyoverdine (14).
In a whole-genome-diversity study in which we compared two cystic fibrosis strains and one environmental strain of P. aeruginosa with the PAO1 reference strain, we found the pyoverdine region to be the most divergent alignable locus in the genome (64). Given the divergence in pyoverdine genes and the variety of different pyoverdine molecular structures, the pyoverdine locus appeared to be a possible target of diversifying selection. To determine the degree and patterns of diversity in pyoverdine genes, we sought to clone, sequence, and analyze the pyoverdine region from multiple strains.
MATERIALS AND METHODS
Bacterial strains and growth conditions.
The strains used in this study are listed in Table Table1.1. Strains were grown overnight on L plates at 37°C. Overnight growth at 37°C with solid or liquid Casamino Acids (Difco) medium was used to induce pyoverdine production. L plates containing 12.5 μg of chloramphenicol per ml were used to grow Escherichia coli containing fosmid clones. XL1-Blue MR E. coli cells were used as the host strain for fosmid clones (Stratagene, La Jolla, Calif.). Saccharomyces cerevisiae transformants carrying yeast capture clones were selected on uracil-deficient medium (63) with 2.5 μg of cycloheximide per ml. L plates containing 6 μg of chloramphenicol per ml were used to grow E. coli containing yeast capture clones. E. coli DH10B cells were used as the host cells for yeast capture cloning (24).
TABLE 1.
Strains used in this study
Strain | Pyoverdine type | Origin | Description | Method used to clone pyoverdine region | Reference | GenBank accession no.(s) |
---|---|---|---|---|---|---|
PAO1 | I | Unknown | Wound isolate | 65 | NC_002516 | |
10-15 | I | Seattle, Wash. | Cystic fibrosis isolate | Fosmid library screen | 12 | AY765259, AY766167 |
PA14 | I | Unknown | Wound isolate | 53 | NZ_AABQ00000000 | |
2-164 | II | Seattle, Wash. | Cystic fibrosis isolate | 64 | AF540993, AY766166 | |
1-60 | II | Seattle, Wash. | Cystic fibrosis isolate | 64 | AF540992, AY766165 | |
MSH | II | Mt. St. Helens, Wash. | Environmental isolate | Fosmid library screen and yeast capture cloning | 64 | AY765263, AY766170 |
206-12 | III | Seattle, Wash. | Cystic fibrosis isolate | Fosmid library screen | 12 | AY765261, AY766168 |
ATCC O13 | III | American Type Culture Collection | O-antigen serotype 13 | Yeast capture cloning | 56 | AY765262, AY766169 |
R′ | IV | Thailand | Hospital isolate | Yeast capture cloning | 61 | AY765260, AY766171 |
Pyoverdine typing.
Pyoverdine strain typing was done via crude pyoverdine purification and isoelectric focusing gel electrophoresis as described (40). Once the nucleotide sequences of each pyoverdine sequence type became available, whole-cell PCR with pyoverdine type-specific primers was used to type strains (sequences available from the authors on request).
Fosmid library creation and screening.
P. aeruginosa genomic DNA was prepared by phenol-chloroform extraction and then further purified by ethanol precipitation. Genomic DNA was partially digested with restriction enzyme Sau3AI and size-separated by pulsed-field gel electrophoresis (Bio-Rad CHEF_DRII apparatus, 1% agarose gel, 14°C, 16 h, pulse ramp 1 to 6 s). DNA segments sized 35 to 52 kb were cut from the gel and recovered by electroelution in dialysis bags (SPETRA/POR molecular porous membrane tubing). Fosmid vector pFos1 was prepared as described (30) and genomic DNA fragments were ligated into the prepared vector with T4 DNA ligase (Roche, Basel, Switzerland).
The ligated vector was then packaged with Giga Pack III XL packaging extract (Stratagene), and E. coli cells were infected. Fosmid-containing colonies were picked into 384-well plates and also pooled into 96-well plates at eight colonies per pool. Fosmid-containing colonies were picked to 10-fold coverage of the genome, assuming average insert sizes of 40 kb.
Fosmid pools were screened with PCR at multiple sites upstream and downstream of the pyoverdine region. Primers were designed to amplify approximately 500-bp fragments and amplicons were spaced 1 to 3 kb apart. Once PCR-positive pools were identified, individual fosmid-containing cells were screened with PCR. Positive individual cells were regrown in 3 ml of L broth at 37°C for 18 h, lysed with 0.2 N NaOH with 1% sodium dodecyl sulfate treatment, and DNA was precipitated with isopropanol. Purified fosmids were retested with PCR and by digestion with multiple restriction enzymes.
Yeast capture cloning.
Yeast capture cloning vectors were assembled via yeast recombination (45). PCR with tailed primers carrying homology to both vector and target DNA was used to build target segments. Primers used to amplify upstream of the pyoverdine region included sequences from genes pvdQ and pvdA, while primers used to amplify downstream of the pyoverdine region included sequences from genes PA2405 and PA2406. Targeting plasmid pEHS6 was assembled by transforming into S. cerevisiae the two target segments, a linearized yeast-E. coli shuttle vector, and a central fragment carrying the yeast wild-type CYH2 gene with the Amp/ori stuffer fragment (57).
Genomic DNA was prepared as described (34). After passing genomic DNA 20 times through a 26.5-gauge needle, sheared genomic DNA and the linearized yeast capture cloning vector were transformed into yeast spheroplasts (57). Plasmid DNA was prepared from pooled yeast colonies and transformed into E. coli cells (26). Yeast capture clones were screened by whole-cell PCR with primers specific to vector-insert junctions.
PCR was used to test for the presence of deletions in strains R′ and ATCC O13 by designing primers at the boundaries of each potential breakpoint. Primers located inside putative deletions were designed with sequence from type III strain 206-12.
Multiple complete digest gels and DNA sequencing of fosmid and yeast capture clones.
Multiple complete digest gels were used to verify the 35- to 52-kb size of each isolated fosmid insert or the size of each yeast capture clone (71). Restriction enzymes EcoRI, BstBI, and NcoI were used. When prior sequence data were available, fragment sizes from newly acquired fosmid or yeast capture clones were compared with those predicted from the sequence.
Shotgun libraries of fosmid and yeast capture clones were made by shearing and ligating prepared DNA into a pBluescript vector. Greater than eightfold sequence coverage was obtained for each clone by standard shotgun sequencing methods. Automated sequence finishing was done with Autofinish software (22), manual finishing was performed if necessary, and sequence assemblies were checked against multiple complete digest gel patterns. Sequences for strains PAO1, PA14, 1-60, and 2-164 were obtained from GenBank.
DNA sequence analysis.
All pairwise sequence alignments were performed with cross_match software, a banded implementation of the Smith Waterman algorithm (http://www.phrap.org/). Gene predictions were performed with Genemark (35). Protein domain predictions were performed with PFAM (6) and NCBI's Conserved Domain Database (36). The EMBOSS program lindna was used to generate windowed single nucleotide polymorphism (SNP) plots in Fig. Fig.1,1, and the EMBOSS programs cusp and cai were used to estimate the codon adaptation index of a gene (58). The codon adaptation index of genes was calculated in reference to a codon table of all genes in the PAO1 genome. Nonribosomal peptide synthetase specificity was predicted with software at the website http://raynam.chm.jhu.edu/≈nrps/ (13). PCR primer design was performed with Primer3 software (60). Hydrophobicity plots were generated with the algorithm of Kyte and Doolittle (32) with a window size of 15 amino acids. The program PROF 1.0 was used to predict the secondary structure of proteins (46).
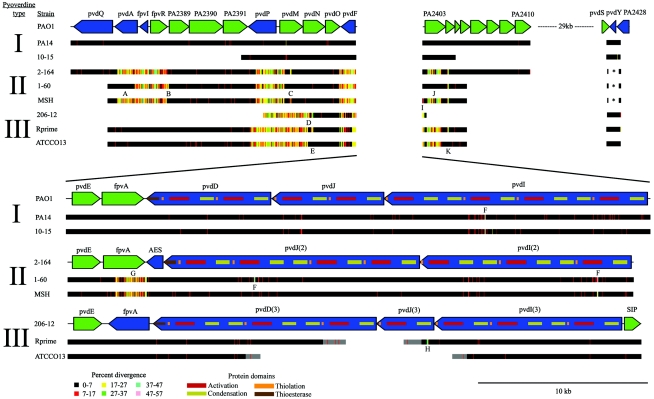
Gene structure and patterns of variation across nine pyoverdine region sequences. Blue and green arrows on the reference sequence line indicate the direction of transcription. In the top panel, the eight other sequences have been aligned to sequence from the reference strain, PAO1; bands of color indicate the local density of single-base-pair differences. In the bottom panel, central-region sequences (each approximately 33 kb) have been aligned with an arbitrarily chosen reference for each type since the central regions are not alignable between types. Protein domains are overlaid on the gene structure of the nonribosomal peptide synthetase genes and are colored as follows: activation domain-red, condensation domain-yellow, thiolation domain-orange, thioesterase domain-brown. Since there is ambiguity in the precise positions of the deletion breakpoints in R′ and ATCC O13, grayed regions show the range of possible breakpoints. While the deletion shown in ATCC O13 is a cloning artifact and that in R′ is a true deletion, both presumably arose by similar mechanisms. Letters A to K indicate regions divergent within pyoverdine sequence types (see Table Table3).3). Two type III genes were predicted to be smaller than colinear genes in other types: pvdP was shorter by more than 250 codons and pvdM was shorter by approximately 50 codons. For simplicity, no effort was made to reflect this length variation in the figure. The scale (in base pairs) is shown in the bottom right of the figure and is consistent throughout the figure. Pyoverdine genes pvdL (PA2424), pvdG (PA2425), and pvdX (PA2428) are not shown in the figure; these genes are not divergent between type I and II strains (no type III sequence is currently available). pvdY has a 468-bp coding region in type I and III strains and a 912-bp coding region in type II strains.
To estimate deviations in tetranucleotide usage in a sequence, we used a Markov maximal-order model (27, 51, 59). This standard model produces an expected count of oligonucleotide usage that accounts for bias in smaller oligonucleotides contained within the oligonucleotide. Let W = (w1, w2, …wm) denote the word formed by m adjacent nucleotides and N(W) denote the observed number of occurrences of the word in a sequence of length n. The expected count E(W) of W is
E(W) = N(w1, w2, …wm−1) N(w2, w3, …wm)/N(w2, w3, …wm−1)
To measure how observed tetranucleotide usage deviated from the expectation, we calculated the frequency of divergence of a word F(W) as the ratio of the observed count N(W) to the expected count E(W). Linear regression analysis was then used to compare F(W) from two sequences, such as the pyoverdine region and the PAO1 genome, and produce an R2 value that estimated the similarity in tetranucleotide usage between the sequences.
Gene names were kept consistent with PAO1 gene naming conventions when predicted protein domains and synteny for genes from different types indicated obvious homologies. The nonribosomal peptide synthetase genes were given gene names consistent with the PAO1 nonribosomal peptide synthetase genes with a number added indicating their pyoverdine type. Type-specific genes were named based on predicted protein domains.
To search for additional type-specific genes outside the divergent pyoverdine region, nucleotide alignments were made between the genomes of type I strains PAO1 and PA14, the unfinished genome of a type II strain (data not shown), and the 0.5X shotgun sequence reads of three type II strains (64). Type III sequence data were not available for comparison outside of the pyoverdine region.
Tests for positive selection.
We used a maximum-likelihood method (73) to estimate whether a gene had experienced positive selection, and if so, we identified the specific codons that were under positive selection. Maximum-likelihood models allow one to test a great number of combinations of parameter values and give a score to each combination according to how well the parameters fit the data; the combination with the best score fits the given data best. Our data were a multiple alignment of nucleotide sequences obtained for each gene in the pyoverdine region. Also input to the models was a phylogenetic tree that was constructed for each gene. We used models whose parameters included the nonsynonymous-to-synonymous ratio ω, the transition-to-transversion ratio κ, and equilibrium codon frequencies π in each codon. ω is a measure of how frequently single-base-pair differences change the amino acid coded for in a codon (as opposed to silent, or synonymous changes): ω < 1 suggests purifying selection, ω = 1 suggests neutral evolution, and ω > 1 suggests positive selection. Values for ω and κ were estimated from the data, and codon frequencies were estimated from nucleotide base frequencies at each of the three codon positions. The genes with the highest scoring combination of parameter values that included a ω greater than 1 were evaluated as being under positive selection.
In practice, maximum likelihood does not test all possible parameters. One must provide a starting point for each parameter, and the software will then incrementally change the parameter values to find a local high score. For each test, we used starting values of 0.1, 1.0, and 3.0 for ω to ensure a broad sampling of likelihood space. When the likelihood scores from each of these starting values agreed, we were confident that we had obtained a true maximum and not a locally maximal score. Below are descriptions of two nested models that have detected positive selection in other systems and which we used to test pyoverdine genes for positive selection.
Model M0 assumed one class of ω for all sites (aligned codons). Model M3 assumed three classes of ω (ω0, ω1, and ω2) each with a respective proportion of sites p (p0, p1, and p2). To compare the models, we calculated twice the log difference between the highest likelihood estimates of models M0 and M3. This difference was compared to a chi square distribution with four degrees of freedom to account for the difference in the number of parameters between the models. Evidence for positive selection in a gene required two conditions: model M3 predict a proportion of codons in a gene to have ω > 1, and the likelihood score of model M3 to be significantly greater than the score of model M0.
To identify specific codons under positive selection, we compared two additional models. Model M7 assumed a beta distribution for values of ω in a gene. Model M8 assumed a beta distribution with an added class of ω for a proportion of sites p1. The beta distribution was used because it allowed for a range of ω values in a gene, but only values of ω between 0 and 1. If some codons in a gene were under positive selection, then model M8 would better fit the data because it allowed a fraction of codons to have ω > 1. Two parameters, p and q, described the shape of the beta distribution. Values for p and q as well as ω and p1 were estimated from the data. We compared twice the log likelihood difference between models M7 and M8 to a chi square distribution with two degrees of freedom. Evidence for positive selection in specific codons required two conditions: model M8 be significantly more likely than model M7, and model M8 predict a fraction of codons with ω > 1. When positive selection was predicted, we could predict specific codons under positive selection by calculating posterior probabilities at each codon with Bayes' theorem. In other words, since we knew that a fraction of codons had a ω value predicted by model M8, we could calculate the probability that each codon belonged to this class of ω.
The program codeml from the PAML software package was used to test genes for positive selection (72). Amino acid multiple alignments were created with ClustalW (67). Alignments were then back-translated to nucleotide sequences and a neighbor-joining tree was generated with the program Neighbor with the phylogenetic software package PHYLIP (18).
To identify the locations of positively selected amino acids in FpvA, we aligned the amino acid sequence of FpvA from PAO1 to sequences from three E. coli proteins of known structure: ferric citrate transporter FecA (19), ferric enterobactin transporter FepA (8), and ferrichrome-iron transporter FhuA (20). Each of these proteins is in the same protein family as FpvA, and contains a TonB-dependent receptor domain (PF00593).
Nucleotide sequence accession number.
Refer to Table Table11 for sequence accession numbers.
RESULTS
Three sequence types at the pyoverdine locus correspond with the three structural types of pyoverdine.
In comparisons of three unfinished P. aeruginosa genomes to reference strain PAO1, we found the pyoverdine region to be the most divergent alignable region in the genome (64). This region of approximately 50 kb in PAO1 is one of the most highly diverged regions in the core P. aeruginosa genome. In the present study we used fosmid cloning and yeast capture cloning to isolate and sequence the pyoverdine region from multiple strains.
The three pyoverdine sequence types correspond to the three known structural types of pyoverdine (see Fig. Fig.1).1). The sequences of the same pyoverdine type have background levels of nucleotide divergence (approximately 0.5%) across the entire region analyzed, while the sequences of different pyoverdine types are highly diverged. The amount of divergence between types is variable in different genes and is highest in the genes located in the center of the region (see Fig. Fig.2).2). The nucleotide sequences from the central part of the region, including pvdE, fpvA, pvdD, pvdI, and pvdJ, are not alignable between pyoverdine types. Figure Figure11 shows a multiple alignment and windowed SNP plot for all sequences, Table Table11 lists the strains used in this study, and Table Table22 lists the functions of individual genes in the region.
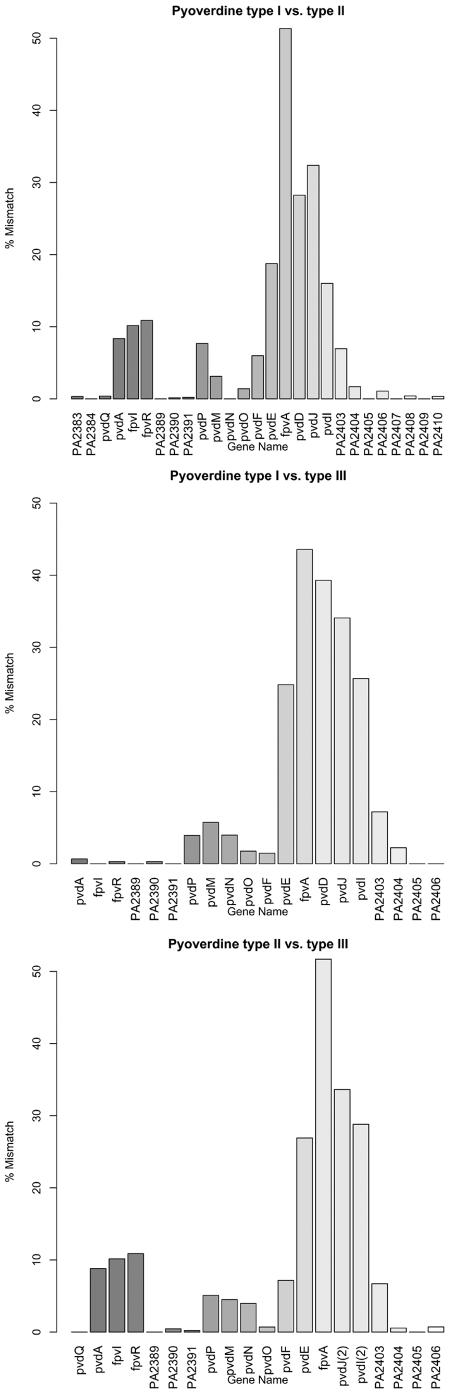
Mismatch in pairwise amino acid alignments between genes of each pyoverdine type. The highest-scoring alignment was used for each gene, and although some predicted gene boundaries differed, alignments spanned the smaller of any two aligned genes. Since the nonribosomal peptide synthetase genes pvdD, pvdJ, and pvdI were highly repetitive and mosaic in structure, the alignments given represent the best match to any nonribosomal peptide synthetase gene in the reference strain for a given type. Strains PAO1, 2-164, and R′ were used as the reference strains for type I, II, and III pyoverdine, respectively.
TABLE 2.
Functions of genes in the pyoverdine region
Gene(s) | Name(s) | Category | Divergence between types | Functiona |
---|---|---|---|---|
PA2385 | pvdQ | Unknown | No | Similarity to aculeacin A acylase |
PA2386 | pvdA | Synthesis | Yes | l-Ornithine-N5-oxygenase |
PA2387 | fpvI | Regulation | Yes | ECF sigma factor |
PA2388 | fpvR | Regulation | Yes | Antisigma transmembrane sensor |
PA2389-PA2391 | opmQ (PA2391) | Unknown | No | Similarity to RND/MFP/OMF efflux pumps |
PA2392 | pvdP | Unknown | Yes | Unknown |
PA2393 | pvdM | Unknown | Yes | Similarity to porcine dipeptidase |
PA2394 | pvdN | Unknown | Yes | Similarity to isopenicillin N-epimerase |
PA2395 | pvdO | Unknown | Yes | Unknown |
PA2396 | pvdF | Synthesis | Yes | N5-hydroxyornithine transformylase |
PA2397 | pvdE | Transport | Yes | ABC transporter |
PA2398 | fpvA | Transport | Yes | Pyoverdine outer membrane receptor |
PA2399-PA2402 | pvdD, pvdJ, pvdI | Synthesis | Yes | Nonribosomal peptide synthetases of the pyoverdine peptide side chain |
PA2403 | Unknown | Yes | Unknown | |
PA2404 | Unknown | Yes | Unknown | |
PA2405-PA2410 | Unknown | No | Membrane proteins and ABC transporter | |
PA2411 | Unknown | No | Similarity to thioesterase | |
PA2412 | Unknown | No | Unknown | |
PA2413 | pvdH | Unknown | No | Similarity to aminotransferase |
PA2424 | pvdL | Synthesis | No | Nonribosomal peptide synthetase of the pyoverdine chromophore |
PA2425 | pvdG | Unknown | No | Probable thioesterase |
PA2426 | pvdS | Regulation | No | ECF sigma factor |
PA2427 | pvdY | Unknown | Yes | Unknown |
PA2428 | pvdX | Unknown | No | Unknown |
The pyoverdine region has unusual codon usage and unusual oligonucleotide usage, both of which suggest a history of horizontal transfer. Codon usage and oligonucleotide usage are phylogenetic signals that reflect the relatedness of bacterial sequences (51, 62). Codon usage in the central type III pyoverdine region is unusual: the codon adaptation index, a measure of synonymous codon bias, of most type III pyoverdine genes is less than 0.55, whereas the genome average is 0.68 (standard deviation = 0.10). Tetranucleotide usage in the region is also unusual; types I and III have a poor fit to the average genome tetranucleotide usage, with R2 = 0.60 and R2 = 0.53, respectively. For comparison, similarly sized sequences from the genome fit the genome average tetranucleotide usage with mean R2 = 0.79 (standard deviation = 0.06). The G+C content of pyoverdine region genes from each type is not significantly different than the genome average of 66.6%. By protein BLAST analysis, the most divergent proteins from each type are similar to pyoverdine proteins from other Pseudomonas species. In PvdE, there is more similarity to a protein from another pseudomonad than to a different P. aeruginosa type. A similar trend occurs in FpvA, except that in two types the highest similarity is with a nonpseudomonal protein; type II FpvA is similar to a receptor from Agrobacterium tumefaciens, and type I FpvA is similar to a receptor from Azotobacter vinelandii.
Approximately 30 kb downstream of the divergent pyoverdine region, pvdY is also divergent between types (Iain Lamont, personal communication). There are two highly diverged alleles of pvdY: type II strains have a 912-bp coding region, and type I and III strains have a 468-bp coding region. The two alleles are not alignable by pairwise nucleotide alignment and have 40% identity at the amino acid level. pvdY from type II strains contains an acetyltransferase protein domain (COG1670, RimL domain, e-value = 7e-06), and both alleles are similar to ribosomal acetyltransferases from other Pseudomonas species. Both alleles of pvdY have unusual codon usage: each allele has a codon adaptation index of less than 0.54, compared to the genome average of 0.68.
Pyoverdine genes adjacent to pvdY, including pvdL (PA2424), pvdG (PA2425), pvdS (PA2426), and pvdX (PA2428), have background levels of divergence between type I and II strains. pvdX, a gene of unknown function that is the only known pyoverdine gene to be expressed in iron-replete conditions, appears to be present in all types and is not divergent between types (68). It is notable that pvdL, a nonribosomal peptide synthetase that synthesizes the pyoverdine chromophore, was not divergent, while the nonribosomal peptide synthetase genes pvdI, pvdJ, and pvdD, which synthesize the pyoverdine side chain, were highly divergent.
We searched for other type-specific genes over an interval from 60 kb upstream to 60 kb downstream of the divergent pyoverdine region. Comparing sequences from strains of types I and II, we found only two candidate genes. Type II strains are missing most or all of gene PA2416. Type II strains also have a predicted gene with a 798-bp coding region between PA2423 and pvdL (PA2424); the gene did not contain a predicted protein domain but encoded a product similar to a hypothetical protein from Chromobacterium violaceum.
Outer membrane receptor gene fpvA and nonribosomal peptide synthetase genes pvdI, pvdJ, and pvdD are the most divergent genes in the pyoverdine region.
Genes that function to synthesize and transport pyoverdine are the most divergent in the region. fpvA, the outer membrane pyoverdine receptor, is the most divergent gene by amino acid alignment (see Fig. Fig.2).2). The next most divergent genes are immediately adjacent to fpvA and include the ABC transporter pvdE and the nonribosomal peptide synthetase genes pvdD, pvdJ, and pvdI. Other genes of moderate divergence between types include pvdP, pvdM, pvdN, pvdO, and PA2403. Type II strains also have moderate divergence in pvdA, fpvI, and fpvR and have a highly divergent downstream gene, pvdY.
Although genes in the central pyoverdine region are highly divergent by amino acid alignment (see Fig. Fig.2),2), most colinear genes probably have related functions. Each type has genes colinear with fpvA, pvdE, and nonribosomal peptide synthetase genes, and the predicted secondary structures of the proteins encoded by both fpvA and pvdE are roughly conserved between types. These genes also share protein domains: fpvA genes have a TonB-dependent receptor domain (Pfam domain PF00593), pvdE genes have ABC transporter (PF00005) and ABC transporter transmembrane region (PF00664) domains, and nonribosomal peptide synthetase genes have activation (AMP-binding, PF00501), thiolation (phosphopantetheine attachment site, PF00550), condensation (PF00668), and thioesterase (PF00975) domains as well as putative epimerization domains. fpvA from each type and the nonribosomal peptide synthetase pvdD have been characterized in detail (1, 17, 50).
Pyoverdine type II and III sequences have type-specific genes.
Type II sequences have a gene with a 966-bp coding region that contains an esterase/lipase protein domain (AES domain, COG0657, e-value = 1e-45) and is located between fpvA and the nonribosomal peptide synthetase pvdJ (2). The AES gene has 69% amino acid similarity and 27% nucleotide divergence from the gene PP4218 in the P. putida genome, which also has an esterase/lipase protein domain and is colinear with esterase/lipase protein domain within the P. putida pyoverdine region (43). There is no significant similarity between the esterase/lipase protein domain and genes in the finished Pseudomonas syringae genome DC3000 (11) or the unfinished Pseudomonas fluorescens genome Pf0-1 (GenBank accession number AAAT03000000).
Type III sequences have a gene with a 954-bp coding region that contains a siderophore-interacting protein domain (SIP domain, COG2375, e-value = 2e-52), and is located between pvdI (3) and PA2403. There is no significant similarity between the siderophore-interacting protein domain and genes in the available Pseudomonas putida, P. syringae, and P. fluorescens genomes.
Most intratype divergence is a result of recombination between types.
There is approximately 0.5% nucleotide divergence between typical pairs of P. aeruginosa strains in most regions of the genome (28, 64). Although sequences of the same pyoverdine type primarily display background levels of divergence, there are 11 instances in the analyzed sequences of dramatically more pronounced divergence within a type (see Table Table3).3). Most intratype differences are approximately 100 bp in length and are located within 400 bp of a region of intertype divergence. Since one allele is typically shared with another type, intratype divergence is the result of recombination between types. Recombination in P. aeruginosa is estimated to be very frequent (28, 47), so this evidence of recombination within the pyoverdine locus is not surprising. It is the swapping of divergent sequence between types that is notable. By shuffling sequences between types at the edges of divergent regions, recombination creates intratype divergence and may simultaneously erode the boundary regions of high intertype divergence.
TABLE 3.
Regions of intratype divergencea
Location in Fig. Fig.11 | Geneb | Length (bp) | Alleles observed in other typesc | Nearest intertype-divergent regiond (bp) |
---|---|---|---|---|
G | fpvA | 1,829 | No | >1,000 |
A | pvdA | 959 | Yes | 0 |
J | PA2403 | 839 | Yes | >1,000 |
E | pvdN | 181 | Yes | 100 |
I | Intergenic between pvdI and PA2403 | 131 | Yes | 133 |
K | PA2404 | 106 | Yes | 350 |
H | pvdD and pvdJ | 95 | Yes | 193 |
F | pvdI and pvdJ | 91 | Yes | 392 |
B | fpvR | 81 | Yes | 0 |
C | pvdM | 75 | Yes | 324 |
D | pvdN | 73 | Yes | 0 |
The outer membrane receptor gene fpvA contains the only example of extensive intratype divergence that cannot be explained in terms of recombination with other known pyoverdine types. Two different alleles exist in type II sequence types: strain 2-164 contains one allele, and strains 1-60 and MSH contain another allele that was recently characterized in detail (17). The alleles are 9% divergent at the nucleotide level and 4% divergent at the amino acid level.
Strain 1-60 contains two examples of an entire gene that is normally type specific that has recombined with one of the other pyoverdine types. Strain 1-60 makes a type II pyoverdine and has predominantly type II sequence in the pyoverdine region. However, the PA2403 and pvdA alleles in 1-60 more closely resemble those in a type I strain. Strain 1-60 appears to be a hybrid, harboring a mixture of alleles typically found in type I or type II strains.
fpvA and PA2403 show evidence of positive selection.
The extensive divergence in the pyoverdine region suggests an unusual evolutionary history. To discover whether positive selection contributed to the divergence, we tested each gene in the region for evidence of positive selection. These tests suggest that fpvA and PA2403, which accumulate nonsynonymous changes at an unusually high rate, are likely to have experienced positive selection (see Table Table44).
TABLE 4.
Evidence for positive selectiona
Gene | No. of sequences | No. of codons | Tree length | Model M0 estimate of ω | Model M8 parameter estimates
| Positively selected sites predicted under model M8 | ||
---|---|---|---|---|---|---|---|---|
p1 | ω | Beta (p,q) | ||||||
fpvA | 9 | 856 | 18.0 | 0.05 | 0.04 | 2.05 | 0.78, 20.20 | 281,316, 440, 485, 501,512, 513, 532,536, 546, 587, 588, 606, 610, 625, 630, 631,659,672, 691 |
PA2403 | 8 | 403 | 1.2 | 0.09 | 0.07 | 2.49 | 0.13, 1.92 | 59, 64, 66, 68, 76, 77, 78, 82, 83,84, 88,95, 102,103, 105, 121, 207, 233, 250, 253, 255, 265, 273,274, 288, 312 |
fpvA contains a TonB-dependent receptor protein domain, consisting of a 22-bp antiparallel-strand β-barrel, a cork domain residing inside the β-barrel, and an N-terminal extension. Using crystal structures from other proteins with this domain, we find that all positively selected residues of FpvA are located in the β-barrel region. Although no proteins are yet known to interact with the β-barrel of FpvA, the β-barrel region of E. coli FhuA may interact with the TonB protein (29).
There is also evidence that positive selection has acted in PA2403. This gene, which is conserved in multiple Pseudomonas species, is of unknown function (55). PA2403 is not required for pyoverdine synthesis, but PA2403 mutants errantly produce pyoverdine in iron-replete conditions (44). With one exception, all of the positively selected amino acids reside outside the four transmembrane domains of the PA2403 protein (see Fig. Fig.4).4). After protein folding, positively selected sites would reside on the same membrane face of the inner membrane, since two transmembrane domains separate the sites.
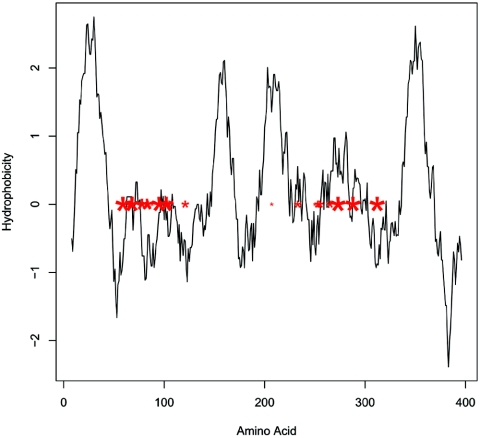
Hydrophobicity plot of PA2403 from PAO1 with codons predicted to be under positive selection superimposed. Four prominent hydrophobic regions (hydrophobicity > 2) likely represent transmembrane domains. Red asterisks represent individual codons predicted to be under positive selection, and asterisk size corresponds to posterior probability of positive selection. With one exception, all amino acids that are predicted to have been under positive selection fall between hydrophobic domains and likely reside on the same side of the membrane after protein folding. Hydrophobicity plots of PA2403 with sequence from pyoverdine type II and III genes give similar results.
The models used to test for positive selection assume that recombination or horizontal transfer did not introduce divergence from another allele (4). Thus, the results of these tests should be viewed with some caution, especially considering the similarity of FpvA to receptors in other species.
Nonribosomal peptide synthetase-predicted amino acid specificities are consistent with pyoverdine structural peptide sequences.
There is excellent correlation between the inferred amino acid sequence made by the nonribosomal peptide synthetase proteins and the primary structure of the pyoverdine that they produce. This correlation is possible because the nonribosomal peptide synthetase proteins are made of a series of cassettes, each of which catalyzes the addition of a particular amino acid to the pyoverdine. A cassette typically contains activation, thiolation, and condensation protein domains, which charge, bind, and connect, respectively, a specific amino acid to a growing peptide. The crystal structure of an activation domain allows homology-based association between cassettes and the amino acid they process during pyoverdine synthesis (13). For each pyoverdine type, the first activation domain in the nonribosomal peptide synthetase operon is specific for the first amino acid in the pyoverdine molecule, the residue closest to the pyoverdine chromophore. Continuing in the order of transcription through the nonribosomal peptide synthetase operon, activation domains are specific to the order of the amino acids in the pyoverdine, proceeding away from the chromophore (see Fig. Fig.33 and and4).4). This order is consistent with the majority of other nonribosomal peptide synthetase enzymes.
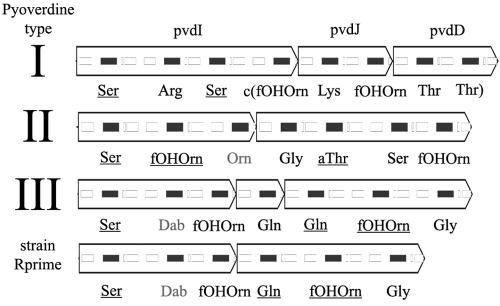
Predicted amino acid specificity of nonribosomal peptide synthetase genes from the pyoverdine region. Protein domains are shown superimposed on genes, solid black rectangles representing activation domains and outlined rectangles representing other nonribosomal peptide synthetase domains. Amino acids in gray were not predicted due to lack of similarity to known sequences. Strain R′ contained an in-frame deletion compared to type III strains that removed one amino acid from the pyoverdine side chain and fused two nonribosomal peptide synthetase genes. Only gene names for pyoverdine type I strains are listed. Underlined residues indicate d-isomers, but specific isomers were not predicted from structural data. fOHOrn, formyl-N5-hydroxyornithine; Dab, diaminobutryic acid; aThr, allo-threonine; c, cyclic.
Previous work showed that R′ synthesizes a rare fourth type of pyoverdine, a type III pyoverdine with a missing glutamine residue (61). Consistent with these findings, R′ has a type III sequence type with a deletion of one nonribosomal peptide synthetase cassette, whose predicted specificity is glutamine.
Nonribosomal peptide synthetase enzymes are studied for their ability to generate many small molecules in a combinatorial fashion (2, 42), although attempts to manipulate the order and specificity of the nonribosomal peptide synthetase enzymes have encountered mixed success. The deletion in R′ represents a natural example of nonribosomal peptide synthetase modification. This event also highlights the arbitrariness of the boundaries between nonribosomal peptide synthetase genes, since the in-frame fusion of two predicted nonribosomal peptide synthetase genes produced a functional product. It is unclear whether the novel peptide sequence in the R′ pyoverdine confers any selective advantage; it is clear that deletion or duplication can rapidly change nonribosomal peptide synthetase genes because of their internally repetitive and highly modular structures.
DISCUSSION
Major genomic differences between strains of P. aeruginosa fall into two broad categories. Some strains contain an insertion of one or more genes that are not present in other strains. Examples include the mobile genetic islands pKLK106 and pKLC102 (31) and pathogenicity islands PAG1 (34), PAG2(C), PAG3(SG) (33), and PAPI-1 and PAPI-2 (25). These insertion-deletion polymorphisms do not occur randomly throughout the genome, but often in hotspots such as specific tRNA genes. Plasmids are similar in that they are present or absent in different strains. In contrast, the pyoverdine region is like the O-antigen biosynthetic region (56), the pilA locus (28), and the flagellar glycosylation region (5): each is present in all strains, but the genes in each locus are highly divergent between strains. This replacement island phenomenon presumably results from diversifying selection, a type of selection that maintains multiple alleles in a population.
It is interesting that alleles of pvdE or fpvA are more similar to genes from other soil bacteria than to other P. aeruginosa alleles. This situation is typical of a region under diversifying selection, where divergent alleles predating a speciation event can be inherited in both new species. However, since there is unusual codon usage and tetranucleotide usage in some pyoverdine types, horizontal transfer seems a more probable explanation for the trans-species polymorphism observed at this locus. Since the pyoverdine region does not show unusual G+C content, perhaps some pyoverdine genes originated in another organism with high G+C content. Other Pseudomonas species are primary candidates for the source of divergent alleles, but high similarity to fpvA was also seen in the high-G+C soil bacteria Agrobacterium tumefaciens and Azotobacter vinelandii.
The pyoverdine outer membrane receptor gene fpvA may drive diversity at the pyoverdine locus. To evolve a new pyoverdine type, both the nonribosomal peptide synthetase and the receptor must coevolve, maintaining their mutual specificity. Receptors are typically specific to a particular pyoverdine structure; however, multiple specificity has been reported, such as in the putative uptake of type II pyoverdine by the type III FpvA receptor (17, 38, 41). Our analysis identifies fpvA as the most divergent gene alignable between types in the region and under positive selection. fpvA is chromosomally located among the most divergent genes in the region and has the only intratype variation that is not a result of recombination between types.
Type II fpvA is an entry site for pyocin S3, a molecule made by some P. aeruginosa strains that is taken up by and lethal to some other strains (7). An alternative type I receptor, fpvB, was recently identified; the receptor is present in strains of each pyoverdine type and is regulated differently than fpvA, suggesting that there is selection to maintain alternative modes of regulation in the receptor (21). Viewed together, these aspects of fpvA function suggest a dynamic evolutionary history, where change in the receptor gene leads to further changes in the system of pyoverdine genes. Adjacent to fpvA, the nonribosomal peptide synthetase genes pvdI, pvdJ, and pvdD and the putative ABC transporter pvdE are also highly divergent and probably coevolve with fpvA. This coevolution would select against recombination events between types that separate these genes, particularly selecting against separation of the receptor gene and the nonribosomal peptide synthetase genes. Repeated rounds of receptor change followed by compensatory mutations elsewhere could result in rapid divergence between pyoverdine sequence types.
Diversifying selection seems to be acting on type-specific genes regardless of their chromosomal location. pvdY is separated by 30 kb from the divergent pyoverdine region but has type-specific divergence. This gene, whose function is currently unknown, presumably has a type-specific function in the pyoverdine system even though it is distantly located from other type-specific genes. It remains possible that there are still other type-specific pyoverdine genes elsewhere in the genome.
One of the most puzzling results of this study is the mixture of alleles in strain 1-60. Two genes in the type II strain have type I alleles, which are significantly divergent from and are present in place of type II alleles. To have become divergent, each allele must have had a selective advantage and must have evolved separately from alleles of other types for a relatively long period of time. Yet we now observe this mixture of alleles functioning together. If the alleles of different types can function together, how have the alleles been able to diverge from one another?
Lastly, we considered possible sources of diversifying selection at the pyoverdine locus of P. aeruginosa. Outer membrane protein genes such as the pyoverdine receptor fpvA are common targets for entry by phage or pyocins, and siderophore diversity may be a resistance mechanism. Siderophore diversity may also be a defense against ferrisiderophore stealing. Siderophore production is a cooperative behavior, since diffusion makes the ferrisiderophore available to any cell with an appropriate receptor. However, all cooperative activities invite cheating (23, 70). Many bacterial genome sequences, including the P. aeruginosa genome, contain numerous putative siderophore receptor genes without the corresponding siderophore synthesis genes (15, 49, 69). In iron-poor environments, it may be beneficial for a bacterial strain to make a siderophore that is distinct in structure from the major varieties present in the environment. This evolutionary dynamic could lead to continual generation of new siderophores whose selective advantage to a particular strain is constantly compromised when other strains acquire a compatible receptor.
Acknowledgments
This work was supported by grant MILLEROOVO (Samuel I. Miller, Principal Investigator) from the Cystic Fibrosis Foundation as well as a Center for Excellence in Genome Sciences grant (P50 HG02351) to Maynard V. Olson.
We thank the staff of the University of Washington Genome Center. We are also grateful to Willie Swanson for advice on testing for positive selection, to Jean-Marie Meyer and Jane Burns for providing strains, to Ruolan Qiu for assistance with fosmid library construction, and to Arnold Kas for software assistance.
REFERENCES
Articles from Journal of Bacteriology are provided here courtesy of American Society for Microbiology (ASM)
Full text links
Read article at publisher's site: https://doi.org/10.1128/jb.187.6.2138-2147.2005
Read article for free, from open access legal sources, via Unpaywall:
https://europepmc.org/articles/pmc1064051?pdf=render
Citations & impact
Impact metrics
Citations of article over time
Alternative metrics
Smart citations by scite.ai
Explore citation contexts and check if this article has been
supported or disputed.
https://scite.ai/reports/10.1128/jb.187.6.2138-2147.2005
Article citations
Feature sequence-based genome mining uncovers the hidden diversity of bacterial siderophore pathways.
Elife, 13:RP96719, 01 Oct 2024
Cited by: 1 article | PMID: 39352117 | PMCID: PMC11444679
Total substitution and partial modification of the set of non-ribosomal peptide synthetases clusters lead to pyoverdine diversity in the Pseudomonas fluorescens complex.
Front Microbiol, 15:1421749, 19 Aug 2024
Cited by: 0 articles | PMID: 39224222 | PMCID: PMC11366639
SOCfinder: a genomic tool for identifying social genes in bacteria.
Microb Genom, 9(12), 01 Dec 2023
Cited by: 1 article | PMID: 38117204 | PMCID: PMC10763506
Spread of Pseudomonas aeruginosa ST274 Clone in Different Niches: Resistome, Virulome, and Phylogenetic Relationship.
Antibiotics (Basel), 12(11):1561, 24 Oct 2023
Cited by: 1 article | PMID: 37998763 | PMCID: PMC10668709
Cold atmospheric pressure plasma-antibiotic synergy in Pseudomonas aeruginosa biofilms is mediated via oxidative stress response.
Biofilm, 5:100122, 07 Apr 2023
Cited by: 9 articles | PMID: 37214348 | PMCID: PMC10196807
Go to all (104) article citations
Data
Data behind the article
This data has been text mined from the article, or deposited into data resources.
BioStudies: supplemental material and supporting data
Nucleotide Sequences (Showing 14 of 14)
- (1 citation) ENA - AF540992
- (1 citation) ENA - AF540993
- (1 citation) ENA - AY766170
- (1 citation) ENA - AY765261
- (1 citation) ENA - AY765260
- (1 citation) ENA - AY766171
- (1 citation) ENA - AY766167
- (1 citation) ENA - AY766166
- (1 citation) ENA - AY766165
- (1 citation) ENA - AY765263
- (1 citation) ENA - AY765262
- (1 citation) ENA - AY766169
- (1 citation) ENA - AY766168
- (1 citation) ENA - AY765259
Show less
Pfam (Showing 7 of 7)
- (2 citations) Pfam - PF00593
- (1 citation) Pfam - PF00501
- (1 citation) Pfam - PF00975
- (1 citation) Pfam - PF00668
- (1 citation) Pfam - PF00550
- (1 citation) Pfam - PF00664
- (1 citation) Pfam - PF00005
Show less
RefSeq - NCBI Reference Sequence Database (2)
- (1 citation) RefSeq - NZ_AABQ00000000
- (1 citation) RefSeq - NC_002516
Similar Articles
To arrive at the top five similar articles we use a word-weighted algorithm to compare words from the Title and Abstract of each citation.
Nucleotide sequence of pvdD, a pyoverdine biosynthetic gene from Pseudomonas aeruginosa: PvdD has similarity to peptide synthetases.
J Bacteriol, 177(1):252-258, 01 Jan 1995
Cited by: 64 articles | PMID: 7798141 | PMCID: PMC176582
The 1.8 A crystal structure of PA2412, an MbtH-like protein from the pyoverdine cluster of Pseudomonas aeruginosa.
J Biol Chem, 282(28):20425-20434, 14 May 2007
Cited by: 50 articles | PMID: 17502378
Subcellular localization of the pyoverdine biogenesis machinery of Pseudomonas aeruginosa: a membrane-associated "siderosome".
FEBS Lett, 587(21):3387-3391, 13 Sep 2013
Cited by: 17 articles | PMID: 24042050
Pyoverdine siderophores: from biogenesis to biosignificance.
Trends Microbiol, 15(1):22-30, 21 Nov 2006
Cited by: 281 articles | PMID: 17118662
Review