Abstract
Free full text

Independent Translocation of Two Micronemal Proteins in Developing Plasmodium falciparum Merozoites
Abstract
Apical membrane antigen 1 of Plasmodium falciparum (PfAMA1) contains an N-terminal propeptide that is removed prior to the translocation of the mature protein onto the merozoite surface. We localized unprocessed PfAMA1 to the microneme organelles of the intraerythrocytic schizont. The results have suggested that the processed form of PfAMA1 translocates from the microneme compartment independently of another microneme protein, EBA175, which is also involved in the invasion of human erythrocytes.
The clinical symptoms of malaria infection result from exponential expansion of parasite numbers during the asexual erythrocytic phase of the Plasmodium life cycle. The precise molecular events mediating erythrocyte invasion are not fully understood, but its rapid nature indicates that it is a tightly controlled process involving specific receptor-ligand interactions between host and parasite (reviewed in reference 7). Molecules located in the apical organelles, as well as those found on the merozoite surface, are believed to play crucial roles.
Extracellular merozoites form an initially reversible attachment to circulating erythrocytes through molecules in the surface coat filaments of the parasite (18). Attached merozoites then reorient to bring the anterior apical pole into alignment with the erythrocyte plasma membrane. Next, an irreversible adhesion, the tight junction, forms between host and parasite membranes, coinciding with an indentation in the erythrocyte membrane and extrusion of the contents of the apical organelles (2, 6). Entry into the host cell is accomplished by posterior movement of the tight junction around the merozoite circumference. When the newly formed parasitophorous vacuole is sealed around the body of the merozoite, invasion is complete.
In Plasmodium falciparum, proteins belonging to the erythrocyte binding protein family have been identified in the micronemes of developing and free merozoites. The best characterized of these is EBA175, which binds to sialic acid residues present on glycophorin A, a highly abundant erythrocyte surface protein (38).
Proteins found in association with the merozoite surface and apical organelles offer considerable potential as components in a malaria vaccine (4). One of the leading candidates for inclusion in a subunit vaccine is apical membrane antigen 1 (AMA1). This molecule has been demonstrated to induce protection against parasite challenge in various animal model systems (3, 12, 15).
P. falciparum AMA1 (PfAMA1) is synthesized in segmenting schizonts as a precursor protein of around 83 kDa, which is then processed N terminally to a 62-kDa form (13). This protein has the structural features of a type 1 integral membrane protein, with short transmembrane and cytoplasmic tail domains. It has previously been localized by immunofluorescence microscopy to the apical organelles and the surface of the merozoite (32), and immunoelectron microscopy (immuno-EM) suggested it to be resident in the rhoptry neck (13). The processed form of AMA1 is thought to translocate to the merozoite surface around the time of schizont rupture (31).
The precise biological role of AMA1 is unknown; however, there is a growing body of evidence suggesting that AMA1 fulfils an essential role during the invasion process (14, 40, 44). AMA1 homologues have been found in all Plasmodium species sequenced to date and also in the related apicomplexan parasite Toxoplasma gondii (17, 21). A comparison of these sequences reveals conservation of all 16 cysteine residues within the ectodomain of the protein, indicating structural constraints on the molecule (23). However, the spacings between the cysteine residues in domain 3 are somewhat different in the plasmodia and Toxoplasma (21). Attempts to delete the gene by targeted disruption have not been successful in either P. falciparum (44) or T. gondii (21). Furthermore, merozoite invasion of erythrocytes is inhibited by both monoclonal antibodies (MAbs) (14, 40) and polyclonal antibodies (22) to AMA1.
In this study, we examine the ultrastructural localization of AMA1 in P. falciparum and show by immunofluorescence microscopy and immuno-EM that the full-length protein is present in the microneme organelles of the developing merozoite.
MATERIALS AND METHODS
Parasites.
P. falciparum clone 3D7, obtained from David Walliker at Edinburgh University, was cultivated in vitro (42) and synchronized (29) according to standard procedures, and it was used in all assays described below. Briefly, ring stage cultures were treated twice with 5% sorbitol 4 h apart; 40 to 48-h-old parasites were used in all assays described below.
Antisera.
Rabbit antisera against EBA175 (34) and AMA1 (22) have been described previously. Briefly, rabbits were immunized with a glutathione S-transferase fusion protein of the region of EBA175 from the F2 domain to the cysteine-rich domain; antibodies were affinity purified using the immunogen coupled to Sepharose beads. Rabbit anti-AMA1 sera were generated against a high-performance liquid chromatography-purified refolded fusion protein comprising amino acids 25 to 472 of the 3D7 sequence (excluding the signal peptide, plus a short N-terminal segment, and the transmembrane and cytoplasmic tail regions) with an N-terminal six-His tag (22). The mouse MAbs 5G8, isotype immunoglobulin G1 (IgG1), with specificity for the AMA1 pro-region, and 1F9, isotype IgG3, which reacts with domain 1 of AMA1, were used in this study (11, 44). These MAbs were raised using refolded Escherichia coli recombinant AMA1 as described above (11).
Western blotting.
Synchronized late schizont stage parasites (40 to 48 h postinvasion) were lysed with 0.15% (wt/vol) saponin and solubilized in sodium dodecyl sulfate-polyacrylamide gel electrophoresis sample buffer with or without 2-mercaptoethanol as a reducing agent (see the legend to Fig. Fig.11 for details) and then fractionated on 8% polyacrylamide gels. Proteins were electrophoretically transferred to nitrocelluose (Schleicher & Schuell, Keene, N.H.), and immunoblotting was performed as previously described (43). Bound antibody was detected with either horseradish peroxidase-coupled sheep anti-mouse or sheep anti-rabbit antibody (Silenus Laboratories, Boronica, Australia) and developed by enhanced chemiluminescence (Amersham International), a luminescence-based method, according to the manufacturer's instructions.

Analysis of recognition patterns of anti-P. falciparum antisera by Western blotting. Saponin-lysed mature 3D7 schizonts were probed with rabbit polyclonal antisera raised against EBA175 (lane 1) and PfAMA1 ectodomain (strain 3D7) (lane 3). The reactivities of mouse MAbs 5G8 (lane 2) against the AMA1 pro-region and 1F9 (lane 4) against AMA1 domain 1 are also shown. The parasite samples in lanes 1 and 2 were treated with 2-mercaptoethanol as a reducing agent, whereas those in lanes 3 and 4 were run under nonreducing conditions, as the epitope for MAb 1F9 has been shown to be reduction sensitive.
Immunofluorescence.
Synchronized late schizont stage parasites were smeared, air dried, and fixed in 100% methanol for 2 min at −20°C. The smears were incubated with a combination of antibodies against P. falciparum proteins. This was followed by incubation with FITC-labeled sheep anti-mouse (Silenus Laboratories) and rhodamine-labeled donkey anti-rabbit IgG (Chemicon, Temecula, Calif.) antibodies. Dual-color fluorescence images were captured using a digital camera and fluorescence microscope (Zeiss, Gottingen, Germany). The DNA stain DAPI (4′,6′-diamidino-2-phenylindole) was included in the second antibody incubation step (8).
Immuno-EM.
Immunolabeling for EM was performed on ultrathin sections of glutaraldehyde-fixed parasites. Mature (40- to 48-h-old) parasites were purified by Percoll density gradient separation, fixed in 1% glutaraldehyde for 1 h at 4°C in RPMI-HEPES buffer, and embedded in White London Resin. Sections were blocked in phosphate-buffered saline containing 0.8% (wt/vol) bovine serum albumin and 0.01% (wt/vol) Tween 80 and then incubated in antiserum diluted in the above-mentioned solution. The antibodies used were rabbit anti-EBA175 and MAb 5G8 against the pro-region of AMA1. Samples were washed and incubated with secondary antibodies conjugated to either 10-, 12-, or 15-nm-diameter gold particles (BioCell). Poststaining with uranyl acetate and lead citrate was followed by observation using a Philips CM120 BioTWIN transmission electron microscope at 120 kV. As a negative control for the immunogold EM, we probed sections with an irrelevant MAb (anti-human serum albumin IgG1; Biotrend, Cologne, Germany) at the same concentration as 5G8 and then reacted sections with anti-mouse secondary antibody conjugated to colloidal gold. No labeling was observed with this negative control.
RESULTS
Unprocessed AMA1 colocalizes with EBA175 in P. falciparum schizonts.
MAb 1F9 is reactive to domain 1 of AMA1 (Fig. (Fig.1,1, lane 4) and recognizes two proteins of 83,000 and 66,000 Da. This agrees with the reported sizes of the full-length and processed forms of AMA1 found in late schizonts (13). MAb 5G8 recognizes the full-length protein and not the processed form of AMA1 (Fig. (Fig.1,1, lane 2), confirming its reactivity to the pro-region of the polypeptide (11). This region is cleaved prior to translocation of the mature form of the protein to the merozoite surface membrane (31). A polyclonal antibody against EBA175 reacted with a single protein in late-stage P. falciparum schizonts, as has been previously shown (Fig. (Fig.1,1, lane 1) (34). A rabbit polyclonal antiserum raised against refolded recombinant AMA1 ectodomain (22) also recognized both full-length and processed forms of parasite-expressed AMA1 (Fig. (Fig.1,1, lane 3).
In immunofluorescence studies of late-stage schizonts, MAb 5G8, which specifically recognizes the unprocessed form of AMA1, showed a punctate pattern characteristic of the apical organelles of merozoites (Fig. (Fig.2a2a and b). Anti-EBA175 antibodies showed the same pattern (Fig. 2c and d), and merging of the images from the FITC and rhodamine channels suggested that these two proteins were colocalized in the same subcellular compartment (Fig. 2e and f). Although the merged images show a very high degree of colocalization, there were still some areas that stained only with FITC or rhodamine. This may reflect some degree of heterogeneity in the amounts of AMA1 and EBA175 present in individual compartments. Nevertheless, this result suggests that full-length AMA1 may be present in the micronemes of P. falciparum schizonts. This would be consistent with the subcellular location of AMA1 in the related apicomplexan parasite T. gondii (17, 21).
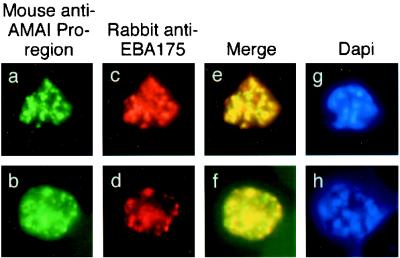
Single and dual indirect immunofluorescence images showing the same subcellular localization of AMA1 and EBA-175 in two mature schizonts of P. falciparum. (a to d) FITC-labeled MAb 5G8 against the PfAMA1 pro-region (a and b) and rhodamine-labeled anti-EBA175 (c and d). (e and f) Merged images show full-length AMA1 colocalized with EBA175. (g and h) DAPI-stained parasites included to show the stage of merozoite development.
Proteolytically cleaved AMA1 translocates within the merozoite before schizont rupture.
When MAb 1F9 was used to detect AMA1 by immunofluorescence (Fig. (Fig.3a3a and b), its staining pattern appeared slightly more diffuse than that of MAb 5G8 (Fig. 2a and b). Staining with 1F9 was also different from that with EBA175 (Fig. 3c and d), and this was confirmed in merged images of the rhodamine and FITC channels, indicating that AMA1 did not completely colocalize with EBA175 in this experiment, although there are some areas of the schizont where both proteins appear in the same compartment (Fig. 3e and f). This suggested that a subpopulation of intraschizont AMA1 was present in a different subcellular compartment than the micronemes, although it is not possible to assign it to a merozoite surface location in these experiments. AMA1 has been previously reported to reside in the neck of the rhoptry (13), and the data presented here may represent the visualization of AMA1 in transit.
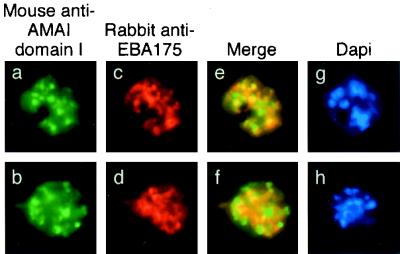
Single and dual indirect immunofluorescence images showing incomplete colocalization of EBA175 and processed AMA1 in mature schizonts of P. falciparum. (a to d) FITC-labeled MAb 1F9 against PfAMA1 domain 1 (a and b) and rhodamine-labeled rabbit anti-EBA175 (c and d). (e and f) Merged images of AMA1 and EBA175 show differential localization of the two proteins. (g and h) DAPI-stained parasites included to show the stage of merozoite development.
To confirm the presence of AMA1 in different subcellular compartments, colocalization immunofluorescence microscopy studies were performed using MAb 5G8 in combination with polyclonal serum recognizing the ectodomain of AMA1 (Fig. (Fig.4).4). Anti-pro-region MAb 5G8 again showed the same punctate staining pattern previously seen (Fig. 4a and b). The polyclonal antibody recognizing the ectodomain of PfAMA1 showed some differences from 5G8 in its staining pattern (Fig. 4c and d), and merged images confirmed partial colocalization of the two AMA1-specific antibodies (Fig. 4e and f). The observation of regions which appeared to stain with only one of the antibodies was unexpected, as most of the pro-region of PfAMA1 was present in the refolded recombinant preparation used to raise the rabbit polyclonal antiserum. This phenomenon may be explicable if the pro-domain is less immunogenic than the mature part of the protein, so that at the titration used in these experiments, the polyclonal serum may not have contained many antibodies which recognized the pro-region. By contrast, MAb 1F9 against AMA1 domain 1 showed almost complete colocalization with the polyclonal anti-AMA1 serum in these assays (Fig. (Fig.5f),5f), an observation which broadly supports the hypothesis that the majority of antibodies in the polyclonal serum are against epitopes outside of the pro-region. However, as in Fig. 2e and f, which show colocalization of full-length AMA1 with EBA175, one of the merged images shows patches of FITC and rhodamine staining (Fig. (Fig.5e).5e). This may indicate that this phenomenon is due to antibody exclusion by steric hindrance, as the two AMA1 antibodies should colocalize completely, as in Fig. Fig.5f.5f. A similar phenomenon has been observed in studies of another merozoite surface protein, MSP1, in which antibodies directed to different regions of the protein can compete with one another for binding (20). Alternatively, it is possible that the polyclonal anti-AMA1 serum does in fact contain antibodies against the pro-domain and that the small patches of green reflect regions of free pro-region where processing has taken place and the mature protein has been translocated (the fate of the short proteolytic cleavage product is unknown). However, this interpretation does not agree with the observations shown in Fig. Fig.4,4, where there was less colocalization between pro-region MAbs and polyclonal anti-ectodomain staining.
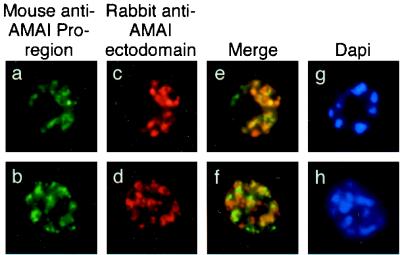
Single and dual indirect immunofluorescence images showing two subpopulations of intramerozoite AMA1 within P. falciparum schizonts. (a and b) FITC-labeled MAb 5G8 against PfAMA1 pro-region showing micronemal localization. (c to f) Rhodamine-labeled rabbit polyclonal serum against AMA1 (c and d) showing apical localization and merged images (e and f) showing incomplete colocalization of the two AMA1 antibodies. (g and h) DAPI-stained parasites included to show the stage of merozoite development.
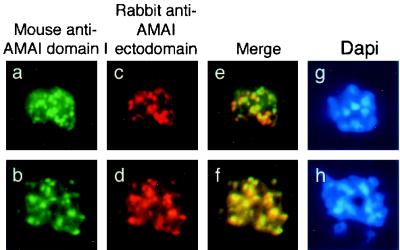
Single and dual indirect immunofluorescence images of two AMA1-specific antibodies in late P. falciparum schizonts. (a and b) FITC-labeled anti-AMA1 domain 1-specific MAb 1F9 showing apical fluorescence. (c and d) Rhodamine-labeled rabbit polyclonal serum against AMA1 showing apical localization. (e and f) Merged images show colocalization of the two AMA1 antibodies. (g and h) DAPI-stained parasites included to show the stage of merozoite development.
In summary, these results suggest that the full-length 83-kDa AMA1 protein bearing the N-terminal pro-region is present within the micronemes, where it is proteolytically processed. The mature 66-kDa protein then moves out of the micronemes following this cleavage event.
AMA1 is present in micronemes prior to proteolytic processing.
In order to determine precisely the ultrastructural location of AMA1, we performed immuno-EM on late-stage P. falciparum schizonts. It had been previously shown by immuno-EM that EBA175 is located in the micronemes of P. falciparum merozoites (37), and this was confirmed in the present study using an independent EBA175-specific antiserum (Fig. (Fig.6c6c and d) (34). EBA175 was not detectable on the surfaces of free merozoites (Fig. (Fig.6d).6d). A previous immuno-EM study of AMA1 reported its presence in the neck of the rhoptry (13). In the present study, using MAb 5G8, specific for the pro-region, AMA1 was detected in a compartment outside of the rhoptry, consistent with the micronemes (Fig. 6a and b). Using this antibody, there was no detectable staining within the neck of the rhoptry in intraschizont merozoites. In double-labeled schizonts, AMA1 and EBA175 colocalized to micronemes clustered around the rhoptries. In the majority of micronemes, only one antigen was detected, and images revealing both antigens within a single microneme were infrequent (Fig. 6e to h). This may be attributable to the small size of micronemes compared to the size of the gold particles, as results of the immunofluorescence experiments suggest that AMA1 and EBA175 are both present within most micronemes, although some micronemes (Fig. 2e and f) appeared to stain only with EBA175 or AMA1.
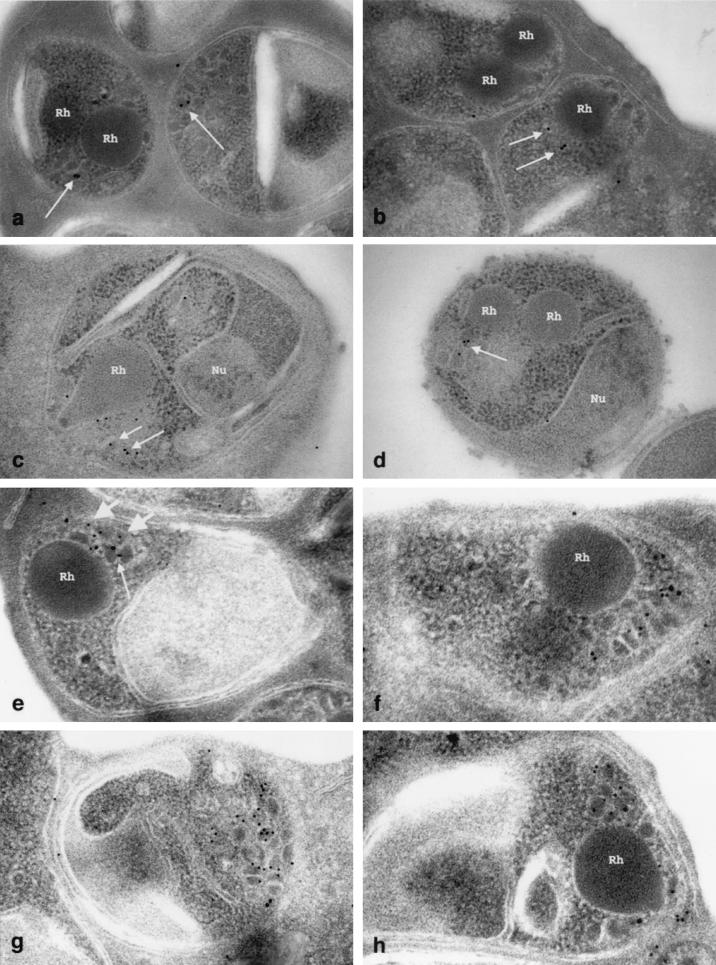
Immunogold localization of AMA1 and EBA175 in late schizonts of P. falciparum 3D7. (a and b) Localization of AMA1 with 15-nm-diameter colloidal gold. Labeling is confined to micronemes (arrows) and absent from rhoptries (Rh). (c and d) Localization of EBA175 with 12-nm-diameter colloidal gold. Labeling is confined to micronemes and absent from rhoptries (Rh), nuclei (Nu), and other structures. (e to h) Double-labeling immunogold for AMA1 (15-nm-diameter gold [small arrow]) and EBA175 (10-nm-diameter gold [large arrows]). Magnification, ×48,000 (a), ×49,000 (b), ×58,000 (c), ×55,000 (d), ×62,000 (e), ×87,000 (f), ×64,000 (g), and ×78,000 (h).
DISCUSSION
PfAMA1 is produced as an 83-kDa polypeptide which undergoes a rapid processing event after synthesis in which a short N-terminal fragment is proteolytically cleaved, giving rise to a 62-kDa mature protein (13, 32). In a previous immuno-EM study, using human serum affinity purified on recombinant fusion protein, AMA1 was detected in the rhoptry necks of merozoites developing within schizonts, although some gold particles also clearly labeled the micronemes (13). In the present study, we used a MAb targeted to the N-terminal pro-region of PfAMA1 to demonstrate conclusively by immunofluorescence microscopy and immuno-EM that unprocessed AMA1 is located in the micronemes. This is consistent with its location in T. gondii (17, 21). No staining of rhoptry necks was evident with the AMA1 pro-region-targeted antibody, suggesting that this portion of the AMA1 protein is retained within the micronemes following proteolytic cleavage. The apparent difference in AMA1 localization between this and the previous study can be explained by the different antibodies used. In the present study, the AMA1 MAb does not recognize processed AMA1, whereas that used in the previous study recognizes both full-length and processed forms of AMA1 (13).
The processed form of PfAMA1 is known to translocate to the merozoite surface, and it has been suggested that this is concurrent with schizont rupture and merozoite release (31, 32). We detected two isoforms of AMA1 by Western blotting of late-stage P. falciparum schizonts, consistent with the reported sizes for full-length and processed AMA1. This confirms previous findings that processing of PfAMA1 occurs prior to schizont rupture (25, 28, 31, 32). Processing has been determined to occur within 15 min of synthesis of the full-length polypeptide (13). Using a MAb specific for unprocessed AMA1, our findings suggest that processing occurs in the micronemes and may be required for initiation of the movement of AMA1 via the rhoptry neck (13) to the merozoite surface.
The results presented here suggest that translocation of N-terminally processed AMA1 may occur independently of that of another microneme protein, EBA175. Two independent studies have demonstrated that expression of these two proteins occurs at the same time in schizont development (41, 44). It has been reported by various authors that AMA1 is detectable on the merozoite surface (22, 31); however, EBA175 has never been conclusively shown to have a merozoite surface location. The differential translocation of two proteins initially found within the micronemes has implications for our understanding of the dynamics of secretion from these organelles and lends credence to the hypothesis that micronemes do not release their contents en masse following cognate binding of the extracellular parasite to erythrocyte membrane receptors (39). If AMA1 moves out of the micronemes independently of EBA175, as our data suggest, it may indicate a level of selectivity of microneme protein transport following the enzymatic cleavage of the AMA1 pro-region, perhaps involving specific signaling through the cytoplasmic-tail region. The cytoplasmic tails of PfAMA1 and its homologues in other species contain the microneme-rhoptry targeting consensus motif YXXΦ, where X is any amino acid and Φ is an amino acid with a bulky hydrophobic side chain (16, 24). It is not known if this motif is necessary or sufficient for correct subcellular localization of this protein. It is also unclear what signaling mechanisms are active in P. falciparum. The cytoplasmic tail of EBA175 also contains this motif, and two independent studies in which the EBA175 protein was truncated short of the 3′ cysteine-rich domain, i.e., lacking transmembrane and cytoplasmic-tail regions, suggest apical localization of C-terminally truncated EBA175 (26, 34), implying that motifs other than those encoded within the cytoplasmic tail may be involved in its subcellular localization.
The role of the N-terminal pro-region of PfAMA1 is still unknown. Plasmodium reichenowi, a close phylogenetic relative of P. falciparum, is the only other species whose AMA1 contains this inserted sequence (27). There is evidence indicating that the sequence is not necessary for trafficking of AMA1, since transspecies complementation studies have demonstrated correct localization of P. falciparum AMA1 in Plasmodium berghei (28) and of Plasmodium chabaudi AMA1 in P. falciparum (44). However, there could be a key motif present in the N-termini of all AMA1 sequences that directs this protein through the micronemes. It is not known whether all AMA1 proteins are N-terminally processed, but it appears that a short N-terminal region of T. gondii AMA1 is removed shortly after its synthesis (17). It has been speculated that all AMA1 proteins may require this processing event prior to their translocation to the parasite surface (25). Removal of the N-terminal region by proteolytic cleavage may be required to allow AMA1 to move out of the micronemes, perhaps by allowing it to bind to an escorter protein (5, 35). Alternatively, a motif present within the N-terminal sequence may act as a microneme retention signal.
Proteolytic processing is a common posttranslational modification of Toxoplasma secretory proteins, usually occurring shortly after synthesis and involving removal of an N-terminal propeptide (9, 10, 17, 33). It is tempting to speculate that one function of the micronemes is to provide an enzymatic milieu in which proteins are proteolytically processed prior to their translocation onto the parasite surface. A recent study identified a subtilisin homologue in the micronemes of T. gondii (30). It will be of interest to determine whether other Plasmodium microneme proteins are processed prior to their secretion and whether the processing event is required for the functioning of these proteins in the invasion process.
In summary, we can now present an updated model of the processing events of AMA1 as it moves from the micronemes to the merozoite surface (25) (Fig. (Fig.7).7). AMA1 is synthesized as an 83-kDa precursor protein that traffics to and is N-terminally processed within the micronemes. The processed AMA1 translocates by an unknown mechanism from the micronemes via the neck of the rhoptry (13) to the surface membrane of the merozoite. In the very elegant early EM studies of apicomplexan parasites, it was observed that the micronemes and rhoptries were sometimes connected (1). This observation was confirmed and extended in another study, which reported branches and connections between these organelles (36). These findings led to the hypothesis that the micronemes and rhoptries were part of a secretory complex of organelles and that the contents of both were excreted through the thin ductules at the neck of the rhoptry (the conoid of the merozoite). Together, our results and those of a previous study (13) suggest that the micronemes may fuse with the rhoptry necks, allowing the transit of AMA1 en route to the merozoite surface.
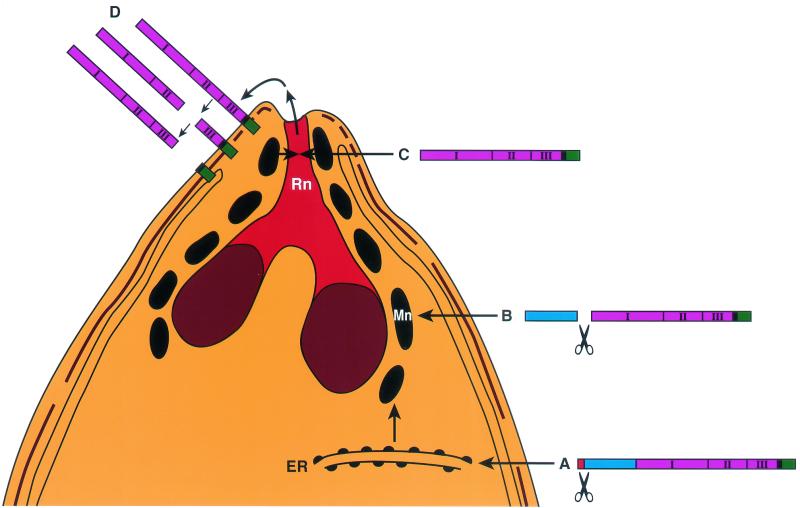
Schematic model of AMA1-processing events in P. falciparum merozoites. AMA1 is synthesized as an 83-kDa precursor protein (A) with signal peptide to allow transport through the endoplasmic reticulum (ER), where removal of the signal peptide presumably occurs. From there it traffics to the micronemes (Mn). The N-terminal pro-region segment is proteolytically cleaved there (B), and the mature 62-kDa polypeptide is translocated out of the micronemes (C) via the neck of the rhoptry (Rn) to the merozoite surface, where it undergoes further C-terminal processing (D), releasing part of the ectodomain off the surface membrane. The domains of AMA1 are represented as follows: signal peptide, red; pro-domain, blue; ectodomains 1, 2, and 3, pink; transmembrane domain, black; and cytoplasmic tail, green.
AMA1 is present over the entire surface of the merozoite but is most densely distributed at the apical tip (22), whereas EBA175 tends to be restricted to the apical end (34) and does not appear to be present on the surfaces of free merozoites. The merozoite surface form of AMA1 is further processed to release two isoforms, PfAMA148 and PfAMA144, into the extracellular milieu (25). Once the merozoite has invaded an erythrocyte, AMA1 is detectable in early ring stages, but only transiently (22). This ring stage AMA1 may represent the small fragment of domain III postcleavage (23).
The function of AMA1 is still unknown, although it is clear that it plays an important role in the merozoite invasion process. A recent study found evidence of erythrocyte binding to Plasmodium yoellii AMA1 domains expressed on Cos cells (19). Elucidation of its subcellular localization and processing events as it moves to the surface of the merozoite will assist in our understanding of the role of this protein in merozoite invasion.
Acknowledgments
We thank Robin Anders and Tony Hodder for AMA1 antibodies used in this study and Tony Hodder for critical appraisal of the manuscript. We acknowledge the Australian Red Cross Blood Bank (Melbourne, Australia) for supplying human erythrocytes and serum.
This work is supported by the National Health and Medical Research Council of Australia. A.F.C. is supported by an International Research Fellowship of the Howard Hughes Medical Institute. J.H. is supported by a Wellcome Trust Travelling Fellowship.
REFERENCES
Articles from Infection and Immunity are provided here courtesy of American Society for Microbiology (ASM)
Full text links
Read article at publisher's site: https://doi.org/10.1128/iai.70.10.5751-5758.2002
Read article for free, from open access legal sources, via Unpaywall:
https://europepmc.org/articles/pmc128307?pdf=render
Citations & impact
Impact metrics
Citations of article over time
Article citations
Toxoplasma FER1 is a versatile and dynamic mediator of differential microneme trafficking and microneme exocytosis.
Sci Rep, 14(1):21819, 18 Sep 2024
Cited by: 3 articles | PMID: 39294204 | PMCID: PMC11410953
Genetic structure of apical membrane antigen-1 in Plasmodium falciparum isolates from Pakistan.
Parasites Hosts Dis, 62(3):302-312, 26 Aug 2024
Cited by: 0 articles | PMID: 39218629 | PMCID: PMC11366544
Atlas of Plasmodium falciparum intraerythrocytic development using expansion microscopy.
Elife, 12:RP88088, 18 Dec 2023
Cited by: 22 articles | PMID: 38108809 | PMCID: PMC10727503
A PPP-type pseudophosphatase is required for the maintenance of basal complex integrity in Plasmodium falciparum.
Nat Commun, 14(1):3916, 03 Jul 2023
Cited by: 4 articles | PMID: 37400439 | PMCID: PMC10317984
Plasmepsin X activates the PCRCR complex of Plasmodium falciparum by processing PfRh5 for erythrocyte invasion.
Nat Commun, 14(1):2219, 19 Apr 2023
Cited by: 3 articles | PMID: 37072430 | PMCID: PMC10113190
Go to all (117) article citations
Data
Similar Articles
To arrive at the top five similar articles we use a word-weighted algorithm to compare words from the Title and Abstract of each citation.
Interaction between Plasmodium falciparum apical membrane antigen 1 and the rhoptry neck protein complex defines a key step in the erythrocyte invasion process of malaria parasites.
J Biol Chem, 285(19):14815-14822, 12 Mar 2010
Cited by: 164 articles | PMID: 20228060 | PMCID: PMC2863225
Plasmodial ortholog of Toxoplasma gondii rhoptry neck protein 3 is localized to the rhoptry body.
Parasitol Int, 60(2):132-138, 13 Jan 2011
Cited by: 24 articles | PMID: 21237287
Rhoptry neck protein RON2 forms a complex with microneme protein AMA1 in Plasmodium falciparum merozoites.
Parasitol Int, 58(1):29-35, 07 Oct 2008
Cited by: 109 articles | PMID: 18952195
Purification of Plasmodium falciparum merozoites for analysis of the processing of merozoite surface protein-1.
Methods Cell Biol, 45:213-220, 01 Jan 1994
Cited by: 61 articles | PMID: 7707987
Review