Abstract
Free full text

Site-directed disulfide bonding reveals an interaction site between energy-coupling protein TonB and BtuB, the outer membrane cobalamin transporter
Abstract
Transport of vitamin B12 across the outer membrane of Escherichia coli, like that of iron-siderophore complexes, is an active transport process requiring a specific outer membrane transporter BtuB, the proton motive force, and the trans-periplasmic energy coupling protein TonB. Interaction between TonB and two of the TonB-dependent siderophore transporters has been detected previously by formaldehyde crosslinking. Here, site-directed disulfide crosslinking demonstrates contact between a conserved region of BtuB, called the TonB-box, and a portion of TonB, previously implicated as the site of suppressors of TonB-box mutations. The specific pattern of disulfide bonding to alternating residues in the TonB-box allowed deduction of the conformation and parallel orientation of the contact region between these two protein segments. Crosslinking at several positions was increased when BtuB was loaded with substrate, and the crosslinking pattern was altered by the presence of substitutions in BtuB that cause a TonB-uncoupled phenotype. This crosslinking process thus reflects protein interactions that are involved in coupling to active transport.
The outer membrane (OM) is the defining structure unique to Gram-negative bacteria. It has distinctive permeability properties that are unusual for a biological membrane and provides a protective barrier against many deleterious agents (reviewed in ref. 1). The low penetration by nonpolar solutes across the OM of most Gram-negative bacteria is attributed to the presence of the highly crosslinked and rigid lipid A molecules in the outer leaflet. Conversely, the anomalously high penetration by polar solutes across the OM is mediated by general and substrate-preferring porins, which allow downhill diffusion of nutrients or metabolic products. In addition, this membrane possesses active transport systems with high affinity and specificity for binding and transport of scarce nutrients, such as iron complexed to siderophores or host iron-binding proteins, and cobalamins. These active transport systems are called TonB-dependent transporters because of their dependence on the energy-coupling function of the trans-periplasmic protein, TonB, and its accessory proteins, ExbB and ExbD (reviewed in refs. 2–4). Vitamin B12 (CN-Cbl) and other cobalamins are accumulated in Escherichia coli by the sequential action of the TonB-dependent BtuB protein in the OM and by the ATP-dependent periplasmic permease BtuCDF in the cytoplasmic membrane (2). BtuB also serves as receptor for the TonB-independent entry of the E and A colicins and of bacteriophage BF23.
The TonB-dependent transporters are not typical biological transporters, as exemplified by their high content of β-sheet structure and their activation by protein interaction rather than by coupling to ion gradients or phosphate bond hydrolysis. However, they are the only active transport systems for which detailed structural information is currently available in the recently described crystal structures of the ferric enterobactin transporter FepA and the ferric hydroxamate transporter FhuA (5–7). The structures of FhuA and FepA are remarkably similar and provide insights into possible mechanisms of substrate transport and energy coupling. Both proteins span the outer membrane by means of a 22-stranded β-barrel with short loops on the periplasmic face and longer loops on the extracellular face. The barrel is comprised of the residues from the C-terminal end of the polypeptide chain. The N-terminal 150–200 amino acids, which include the most conserved sequences of this family of transporters, form a novel structure that fits into the barrel and is held in place by at least 40 hydrogen bonds with residues in the barrel walls. Although this domain was originally referred to as a “plug” or “cork,” we prefer the term “hatch,” reflecting its active role in the transport process, its probable retention in the barrel during substrate uptake, and its direct participation in substrate and TonB recognition (8). The structure of the ligand-bound form of FhuA confirmed previous suggestions that conformational changes occur on substrate binding (9–12), but these changes were surprisingly subtle. The most dramatic change on substrate binding occurs on the periplasmic face of the hatch domain, far from the ligand-binding site. This change involves the unfolding of helix-1 and the movement of the N-terminal segment of the protein preceding helix-1 by as much as 17 Å relative to the rest of the protein (6, 7).
This N-terminal segment has been implicated in active transport and was called Tonbox-1, here the TonB-box. The TonB-box consists of 7 amino acid residues (Asp6-Thr-Leu-Val-Val-Thr-Ala12 in BtuB), which are highly conserved in the TonB-dependent transporters. In all proteins that were examined, some mutations affecting this region eliminated TonB-dependent transport but not TonB-independent activities or substrate binding (2, 13). Surprisingly, extensive changes in the amino acid sequence of this region in BtuB had little or no effect on CN-Cbl uptake rates (14). The most deleterious changes for CN-Cbl uptake introduced glycine or proline substitutions at positions 8 and 10. These results suggested that the contribution of the TonB-box for transport activity was not strongly dependent on residue-specific interactions, but on polypeptide chain configuration, suggesting that other portions of BtuB also might interact with TonB. The TonB-uncoupled transport phenotype of these mutants was weakly suppressed in an allele-specific manner by tonB mutations that changed glutamine-160 near a highly conserved part of TonB (15, 16). These results suggested the existence of a functional interaction between TonB and the TonB-box of the transporters.
The physical interaction between TonB and two TonB-dependent transporters, FepA and FhuA, has been demonstrated by in vivo formaldehyde crosslinking (17–19). A mutation in the TonB-box of FepA, I14P, which rendered it inactive for transport, prevented crosslinking to TonB (19). This approach identified regions of TonB that were necessary for crosslinking with FepA but could not determine whether these residues were directly involved in complex formation.
In the present study, site-directed disulfide crosslinking was used to study the potential interaction of the TonB-box of BtuB and the portion of TonB that was implicated by the genetic suppression analysis. For this purpose, single cysteine residues were introduced at successive positions in the TonB-box of BtuB and were found to form disulfide bonds with single cysteine residues in TonB at and near residue 160. The fact that different pairs of cysteine residues formed disulfide bonds with different efficiencies provides evidence for the potential structure and orientation of these interacting surfaces. Finally, changes in the pattern of disulfide bonding in the presence of TonB-uncoupling mutations indicated the relevance of the contact surfaces deduced from the cross-linking behavior. This approach demonstrates an interaction between specific residues of TonB and a TonB-dependent transporter.
MATERIALS AND METHODS
Bacterial Strains.
The E. coli K12 strain used for plasmid construction and maintenance was JM109. Other strains used in this study were derived from strain MC4100 (20). Strain RK5016 carries the additional mutations metE70 argH btuB recA; strain RK5043 carries metE70 tonB recA. Plasmid-bearing cells were grown in the presence of ampicillin (100 μg/ml) for the btuB plasmids and chloramphenicol (40 μg/ml) for the tonB plasmids.
Construction of the Plasmids Containing btuB and tonB/exbBD.
All DNA manipulations followed standard protocols (21). Plasmid pAG1 carrying the btuB gene in pBR322 was previously described (14). Plasmids containing the tonB and/or the exbBD genes were constructed by cloning these genes from the chromosome of E. coli strain JM109 using PCR with primers external to each coding sequence that add unique restriction sites. These genes were cloned with their own promoters, tonB as a 988-bp PstI-SalI fragment and exbBD as a 1,321-bp AvaI-SacI fragment, and were inserted into the multiple cloning site of plasmid pSU19 to yield plasmid pNC1.
Construction of BtuB and TonB Mutants.
The single cysteine residue in wild-type TonB was replaced by an alanine residue by using a two-step PCR method to yield TonB-C18A. Single cysteine residues were introduced at positions 160, 162, and 163 of TonB and in BtuB (residues 6–12 in the TonB-box, and residues 25 and 26) by using a two-step PCR method. Similarly, proline substitutions were introduced in BtuB at positions 8 and 10, and double mutants were constructed that combined these prolines with a cysteine at position 6 or 12. All mutagenic changes were confirmed by nucleotide sequencing. The tonB mutant plasmids were transferred by transformation into the tonB strain RK5043, and the btuB mutants were transformed into the btuB strain RK5016 for phenotypic analysis. All tonB and btuB mutant plasmids were introduced in pairwise combinations into RK5016 for crosslinking analysis.
Growth on Vitamin B12.
Strains were tested for growth phenotype on minimal A salts-agar plates supplemented with glucose, arginine, and different concentrations of CN-Cbl (0.1 to 5000 nM) in place of methionine (22).
Cross-Linking Assay.
Strains carrying pairs of plasmids were grown in minimal A salts supplemented with 0.01% methionine and arginine, 0.02% glucose, 10 mM MgSO4, 1 mM CaCl2, 100 μg/ml ampicillin, and 40 μg/ml chloramphenicol. Overnight cultures were diluted 1:9 into the same medium in duplicate 4.5-ml cultures and were incubated for 1.25 hr at 37°C with aeration. CN-Cbl (5 μM final concentration) then was added to one series. Both cultures were incubated for an additional 15 min, were harvested by centrifugation and were washed and suspended in 500 μl of PBS. The OD595 of each suspension was adjusted to 1.0, and 10-μl samples were boiled for 5 min in nonreducing sample buffer containing 50 mM iodoacetamide before analysis by SDS/PAGE and Western immunoblot analysis.
SDS/PAGE and Western Immunoblot Analysis.
The bacterial lysates were resolved by SDS/PAGE at 30 mA on gels with 9.5% (wt/vol) acrylamide by using the discontinuous buffer system of Laemmli (23). The resolved proteins were transferred from the gels to nitrocellulose membranes (Bio-Rad) by transverse electrophoresis for 1.5 hr at 500 mA in buffer consisting of 25 mM TrisHCl (pH 8.3), 192 mM glycine, and 20% (vol/vol) methanol (24). The membranes then were blocked in PBS containing 2.5% dried nonfat milk for >1 hr and reacted for 1 hr at room temperature with monoclonal antibody against either BtuB (4B1; 1:10) or TonB (4H4; 1:20,000), diluted in the same buffer. The α-BtuB mAb was prepared at the University of Virginia Lymphocyte Culture Center by using SDS-denatured, gel-purified BtuB as antigen. The α-TonB mAb was kindly provided by K. Postle (Washington State University). The membranes then were washed 3 times for 10 min in PBS containing 0.02% Tween-20 and then were incubated for 1 hr in affinity-purified horseradish peroxidase-labeled goat anti-mouse-IgG antibodies (Jackson Immunoresearch), diluted 1:5,000 in PBS containing 2.5% dried nonfat milk. After extensive washing, the blots were developed by using the chemiluminescent substrate LumiGlo (Kirkegaard & Perry Laboratories) and were exposed to x-ray film (Kodak XAR).
Two-Dimensional Gel Electrophoresis.
Bacterial lysates first were resolved as described above in duplicate lanes on nonreducing SDS/PAGE (9.5% acrylamide; 0.75 mm thick), but with 5 μl of Rainbow High Molecular Weight Markers (Amersham Pharmacia) added to the samples as guides. One lane was cut from the nonreducing gels, was soaked in reducing sample buffer, and was placed on top of a 1.5-mm-thick 9.5% polyacrylamide gel containing 2-mercaptoethanol. A sample of the original bacterial suspension was boiled in reducing sample buffer and was loaded in an adjacent lane. Proteins were resolved by electrophoresis as above. After resolution, proteins were electrophoretically transferred to a nitrocellulose membrane as described above. A duplicate lane from the first gel was simultaneously transferred by using the colored protein markers for alignment. Western immunoblots were performed as described above.
RESULTS
Monocysteine Derivatives of BtuB and TonB Are Functional. Site-directed disulfide crosslinking analysis was carried out by using monocysteine derivatives of BtuB and TonB expressed from compatible, moderate-copy-number plasmids. Multicopy expression was required to detect the crosslinked products of these minor cellular proteins. Cysteine residues were introduced at successive positions in the TonB-box of BtuB by site-directed mutagenesis, yielding the D6C, T7C, L8C, V9C, V10C, T11C, and A12C variants. Because overexpressed TonB is unstable in the absence of its accessory protein ExbB (25, 26), the tonB-expression plasmid pNC1 also carried the exbBD operon. The cysteine-18 residue in wild-type TonB was changed to alanine, and then cysteine residues were introduced to yield the Q160C, Q162C, and Y163C variants.
Plasmids expressing the wild-type or cysteine-substituted derivatives of BtuB and TonB were introduced by transformation into the btuB mutant RK5016 and the tonB mutant RK5043 for phenotypic analysis. The growth response conferred by each variant protein to CN-Cbl, which is a sensitive assay for CN-Cbl uptake activity, was comparable to wild-type protein (N.C., P. Phan, and R.J.K., unpublished work). As shown below, the monocysteine variant proteins were present at comparable amounts as the wild-type proteins expressed from the plasmids.
Formation of Disulfide Bonds Between BtuB and TonB. Paired combinations of plasmid pAG1 expressing wild-type BtuB or the cysteine substitutions in the TonB-box and plasmid pNC1 expressing wild-type (C18A) or the three cysteine substitutions in TonB were introduced into strain RK5016. Cells in mid-log phase of growth were lysed by the addition of sample buffer containing iodoacetamide to block further disulfide bond formation. Cellular proteins were resolved by SDS/PAGE in the absence of reducing agent and were subjected to immunodetection with monoclonal antibodies to BtuB and to TonB.
Fig. Fig.11 presents the Western immunoblot analysis of proteins from strains expressing the cys-less and Q160C forms of TonB and the cysteine substitutions in the TonB-box of BtuB. Immunodetection with anti-BtuB (Fig. (Fig.11A) revealed that cells expressing the wild-type form of BtuB contained a single immunoreactive species migrating at the expected monomer position of 66 kDa. When BtuB carried a TonB-box cysteine, in combination with cys-less TonB, the monomer species was accompanied by the appearance of minor amounts of a ≈130-kDa species migrating as expected for a BtuB dimer. When TonB-Q160C was expressed in combination with wild-type BtuB, BtuB appeared only as the 66-kDa monomer. However, when TonB-Q160C was expressed together with BtuB variants with TonB-box cysteines, some BtuB variants resulted in the appearance of additional species; particularly prominent were the two bands migrating at ≈100 and ≈90 kDa. Thus, production of these two BtuB-containing species depended on the presence of Cys residues in both TonB and BtuB.
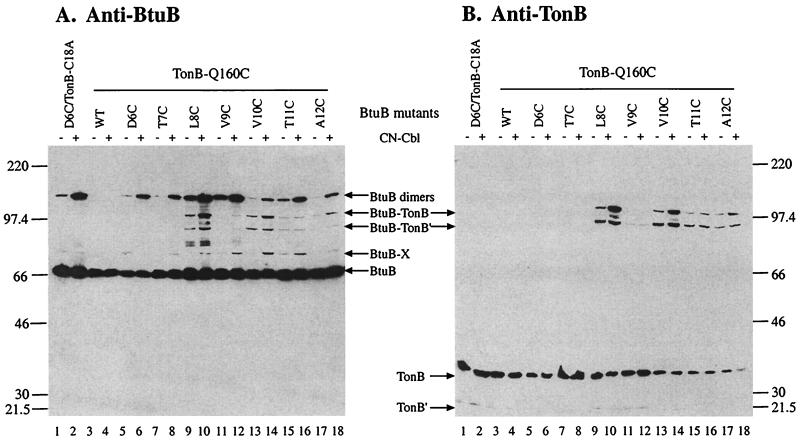
Western immunoblots showing disulfide crosslinking between TonB-Q160C and BtuB variants containing single cysteine residues in the TonB-box region (residues 6 to 12). Cells of strain RK5016 expressing the indicated BtuB and TonB protein variants were grown and treated as described in Materials and Methods. The presence or absence of 5 μM CN-Cbl for 15 min before harvest is indicated on the top. Duplicate gels were analyzed by Western immunoblot analysis using as primary antibodies α-BtuB (A) and α-TonB (B) monoclonal antibodies. The mobilities of molecular weight standards, in kilodaltons, are indicated on the sides. The deduced composition of the immunoreactive species are indicated by arrows.
When a duplicate blot was probed with antibody to TonB (Fig. (Fig.11B), it was seen that both TonB C18A and TonB-Q160C migrated as two species at 35 and 25 kDa. The 35-kDa species corresponds to full-length TonB, and the 25-kDa truncated form of TonB, TonB′, has been described (27, 28). When TonB-Q160C was coexpressed with the BtuB variants with TonB-box cysteines, TonB-containing complexes were observed at 100 and 90 kDa, with the same position and relative distributions as those detected by using the α-BtuB mAb. These are the only species that reacted with antibodies to both BtuB and TonB and demonstrate the formation of disulfide-linked complexes between the TonB-box of BtuB and TonB-Q160C.
Multiple Crosslinked BtuB-TonB Species. The composition of the multiple complexes containing BtuB and TonB was investigated by two-dimensional gel electrophoresis. In the first dimension, proteins were separated by SDS/PAGE under nonreducing conditions, followed by SDS/PAGE in the second dimension under reducing conditions. Fig. Fig.22 shows the results obtained on coexpression of TonB-Q160C and BtuB-V10C; similar results were obtained with other pairs of mutants. All four crosslinked complexes that reacted with α-BtuB mAb liberated full-length 66-kDa BtuB on disulfide bond reduction.
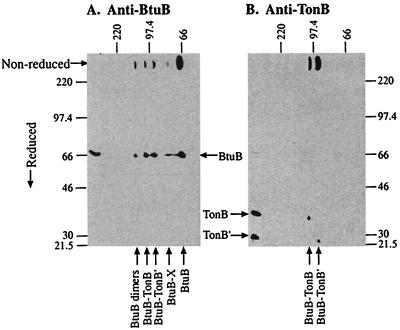
Two-dimensional electrophoretic analysis showing the composition of crosslinked complexes. Proteins from strain RK5016 expressing BtuB-V10C and TonB-Q160C were separated by electrophoresis in the first, horizontal dimension in the absence of 2-mercaptoethanol, followed by electrophoresis in the second, vertical dimension under reducing conditions. Western immunoanalysis used α-BtuB (A) or α-TonB (B) monoclonal antibody for detection of resolved proteins. Along the top and left side of each figure were placed duplicate samples that had been electrophoresed in one dimension in the indicated absence or presence of reducing agent. The mobilities of molecular weight standards are indicated on the sides of each panel, and the deduced identity of each protein species is indicated by arrows.
In contrast, the two crosslinked species that were detected with α-TonB mAb and comigrated with the middle two α-BtuB-reactive complexes were found to contain two TonB polypeptides on reduction of the disulfide bonds. Full-length 35-kDa TonB was present in the 100-kDa BtuB-TonB complex whereas the truncated 25-kDa TonB′ was present in the 90-kDa BtuB-TonB′ complex. The additivity of the apparent molecular weights of the components in these crosslinked complexes indicates that the complexes consist of single molecules of BtuB linked to TonB or TonB′. These results also demonstrated that all of the BtuB- and TonB-containing complexes are dissociated by exposure to 2-mercaptoethanol, conditions that reduce disulfide bonds. Two additional BtuB-containing complexes were observed. The 130-kDa is likely to be a BtuB dimer. The nature of the smallest crosslinked complex, termed BtuB-X, is unknown but could contain a part of TonB lacking the epitope recognized by the α-TonB mAb.
Position Dependence of Crosslinking. There was a marked difference in the level of disulfide crosslinking observed between different pairs of TonB and BtuB proteins. As shown in Fig. Fig.11A and B, TonB-Q160C formed no detectable crosslinks with BtuB-D6C or T7C. Substantial amounts of crosslinking between TonB-Q160C and L8C and V10C were observed whereas crosslinking to T11C and A12C was weaker, and to V9C weaker still.
The ability of two other variants of TonB, TonB-Q162C and TonB-Y163C, to form disulfide-bonded crosslinks to the BtuB TonB-box was determined. Each had a characteristic pattern of bonding that was different from that of TonB-Q160C. TonB-Q162C crosslinked effectively to BtuB-L8C and A12C, less effectively to V10C, even less to T11C, and not at all to D6C, T7C, and V9C (Fig. (Fig.33A). In contrast, TonB-Y163C formed crosslinks only to A12C (Fig. (Fig.33B).
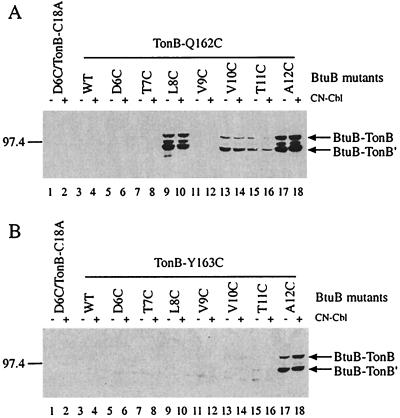
Western immunoblots showing disulfide crosslinking in vivo between BtuB variants containing cysteine in the TonB-box and TonB-Q162C (A) or TonB-Y163C (B). Conditions are as described in the legend to Fig. Fig.1.1. Only the crosslinked species reactive with α-TonB are shown, but comparable results were obtained by using α-BtuB for detection.
As a negative control to test the specificity of the crosslinking reaction, we examined crosslinking of the TonB cysteine-derivatives to the BtuB P25C and T26C variants, located just downstream of the TonB-box. These two BtuB derivatives formed no detectable crosslinks to any TonB variant (data not shown).
Effect of Transport Substrate on Crosslinking.
To test whether the presence of a BtuB transport substrate affected the formation of crosslinks, CN-Cbl was added for 15 min before cell harvest. The presence of CN-Cbl for even this short period strongly increased the production of the putative BtuB dimer complex and the BtuB-X complex (Fig. (Fig.11A). In the presence of TonB-Q160C, CN-Cbl produced a substantial increase in the formation of both BtuB-TonB complexes but had a greater effect on formation of the full-length species (Fig. (Fig.1).1). In contrast, CN-Cbl had a smaller relative effect on crosslinking of TonB-Y163C (Fig. (Fig.33B) and had no obvious effect on crosslinking of TonB-Q162C (Fig. (Fig.33A). Thus, the magnitude of the stimulation of crosslinking by substrate depended on the position of the crosslinked residues.
Change in Crosslinking Specificity by Uncoupling Mutations. The BtuB L8P and V10P mutants exhibit a TonB-uncoupled phenotype, which can be partially suppressed by changes at residue 160 of TonB (15). To examine whether these btuB mutations affect the ability to form crosslinks with TonB, two double mutants were examined. The D6C V10P and L8P A12C BtuB variants contain a single cysteine for crosslinking, along with one of the uncoupling substitutions. These variants were coexpressed with each of the cysteine variants of TonB, and substantial changes in crosslinking were found in comparison to the BtuB cysteine substitutions alone (Fig. (Fig.4).4). In the otherwise wild-type protein, BtuB-D6C did not form crosslinks with any of the TonB derivatives. However, the BtuB-D6C V10P double mutant showed extensive crosslinking to TonB-Q160C, weak linking to TonB-Q162C, and no linking to TonB-Y163C. Likewise, BtuB residue A12C normally forms crosslinks with each of the TonB cysteines. The presence of the L8P substitution resulted in increased crosslinking of A12C to TonB-Q160C and almost complete absence of reaction with TonB-Y163C.
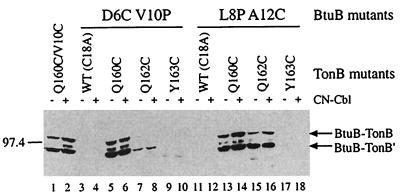
Western immunoblot showing the change in disulfide crosslinking pattern between the TonB cysteine derivatives and the BtuB TonB-box mutants D6C and A12C in the presence of the uncoupling mutations V10P and L8P, respectively. Conditions for bacterial growth in the absence and presence of CN-Cbl and for separation and detection of proteins were as described in the legend to Fig. Fig.1.1. Only the crosslinked species reactive with α-TonB are shown, but comparable results were obtained by using α-BtuB for detection. The mobility of the molecular weight standard and the deduced composition of protein complexes are indicated on the sides of the figure.
These results showed that the presence of the uncoupling mutations in BtuB markedly changed the pattern of crosslinking with the TonB variants. We had initially expected that there would be no crosslinking, based on the hypothesis that the uncoupling mutations prevent or weaken the BtuB-TonB recognition to prevent energy coupling. To ensure that the double BtuB mutants still conferred the uncoupled phenotype, their effect on the growth response to CN-Cbl was examined. It was seen that the double mutants were not as defective as the L8P or V10P substitutions alone. This modulation in phenotype was reminiscent of our previous finding that intragenic suppressors of the defect in the L8P and V10G substitutions arose from changes at alanine-12 and threonine-7, respectively (14). In addition, the TonB cysteine substitutions conferred partial suppressor activity for the BtuB variants L8P and V10P (N.C. and R.J.K., unpublished work). Nonetheless, these results clearly showed that the substitutions that result in the uncoupled phenotype cause a substantial change in the pattern of disulfide crosslinking.
DISCUSSION
The TonB-dependent transporters are currently the only active transport systems of known structure. It is generally accepted that TonB and its accessory proteins serve to couple metabolic energy to the energy-dependent processes of translocation of substrates (siderophores, cobalamins, bacteriocins, or phages) across the OM. The energy-coupling process likely involves specific interactions of TonB and its client transporters. This model is based on numerous observations. TonB activity alters the conformation of the transporters, as shown by changes in binding affinity for TonB-independent substrates (29, 30). Sequence changes in the TonB-box of all TonB-dependent transporters that were examined exhibit the same defect in TonB-dependent energy coupling and can be corrected by changes in a small region of TonB. Formaldehyde-crosslinking of TonB with some TonB-dependent transporters indicates their close proximity at some stage of the transport process. Finally, the structures of FhuA and FepA indicate a dramatic change in the conformation and orientation of the TonB-box in response to substrate binding at the outer face of the OM. The results described here provide more detailed information about the sites of interaction of TonB and BtuB.
We used the technique of site-directed disulfide crosslinking, which is based on the requirement for close proximity and proper orientation of two cysteine residues to allow their formation of a disulfide bond under oxidizing conditions. It was not necessary to add oxidizing agents to obtain BtuB-TonB crosslinking. The periplasmic environment is nonreducing, in part because of the action of the DsbAB redox system (31). The dependence of crosslinking on the positions of the participating cysteine residues in both proteins, and the absence of crosslinking of both proteins to other periplasmic and outer membrane proteins, reveals the high specificity of these interactions and confirms that these portions of BtuB and TonB must encounter each other in a specific manner. The apparent formation of BtuB dimers, and the strong stimulation by CN-Cbl, was surprising because BtuB was not suspected to oligomerize and the TonB-boxes in the crystal structures did not appear to extend into the periplasmic space. The results suggest instead that the TonB-box may extend substantially into the periplasmic space to allow contact to another molecule when substrate is bound.
Our results indicate that the TonB-box region of BtuB contacts the region around residue 160 of TonB, as predicted from the genetic suppressor analysis. Fig. Fig.55 presents a summary of the patterns of crosslinking that were observed. The specificity and the pattern of disulfide bond formation suggests that these sections of both proteins approach each other in a defined orientation. Thus, TonB-Q160C reacted preferentially with BtuB at positions 8 and 10, Q162C reacted preferentially with positions 8 and 12, and position Y163C reacted exclusively with position 12. This pattern is consistent with a parallel, N-to-C alignment of both proteins at this interaction site. The fact that BtuB positions 8, 10, and 12 reacted with all three TonB cysteines to a much greater extent than did positions 7, 9, and 11 suggests that this portion of BtuB folds in a β-sheet structure in which alternating residue side chains face the same direction relative to TonB. In the crystal structures, this region of FhuA and FepA did not assume a fixed secondary structure and was at the edge of the ordered polypeptide structure. There is insufficient information to predict a conformation for this region of TonB, but the ability of positions 160, 162, and 163 to react with the same portions of BtuB is not inconsistent with a helical orientation of this region.
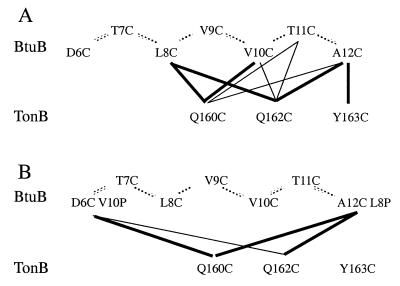
Schematic representation of the disulfide bonds formed between different pairs of BtuB and TonB cysteine derivatives. (A) Pairs of single cysteine substitutions alone. (B) Pairs of cysteine substitutions in the double mutants BtuB D6C V10P and A12C L8P. The thickness of the lines reflects the relative amounts of crosslinks formed.
Although the TonB-box and a region of TonB come into sufficient proximity for efficient disulfide bond formation, it is unlikely that these are the only portions of both proteins that form specific contacts, although no other candidate regions have been identified yet. Because of the very weak sequence requirements for function of the TonB-box, TonB is likely to bind to other parts of the transporter, such as periplasmic loops of the barrel, other parts of the hatch, or both. In our experiments, the presence of the transport substrate, CN-Cbl, had different effects on the formation of crosslinks, depending on the positions involved. When proximity was examined by the formaldehyde crosslinking technique, it was found that the interaction of TonB with FhuA and FepA was strongly stimulated by or dependent on the presence of transport substrate (18, 28). Also, the FepA-I14P change interfered with crosslinking (19). These apparent differences from our results are not contradictory and can be explained if contact of TonB to certain surfaces on the client proteins is affected by the presence of substrate or the conformation of the TonB-box but contact with other surfaces is not. Thus, the apparent degree of dependence of crosslinking on the presence of substrate will depend on which contacts are being detected. Our disulfide crosslinking approach examines very specific contacts. Formaldehyde crosslinking is probably more promiscuous but still requires proximity of reactive residues, mainly lysines. Thus, there is no inherent discrepancy between the different crosslinking studies.
These results lead to a simple model for the action of TonB in transport activity. The presence of bound substrate at the external face of the transporter is marked by an allosteric modification of the structure of the periplasmic face of the hatch domain, in which the TonB-box region swings across the barrel opening. TonB might recognize substrate-loaded receptors by making specific contacts with both the TonB-box and other positions of the barrel. Perhaps the energized form of TonB returns the TonB-box back to its original position. If this displacement is coupled to an allosteric change at the substrate-binding site such that the substrate is released in a direction that is occluded from the exterior of the cell, active transport results. If the released substrate can diffuse only into the periplasm and not into the medium, it will be accumulated in the periplasm at a concentration higher than in the medium.
This simple model might provide an explanation for the peculiar lack of sequence dependence of the conserved TonB-box and for the uncoupling effect of only mutations that disrupt protein secondary structure at certain positions. It is noteworthy to reemphasize that the residues in the TonB-box at which mutations result in the uncoupled phenotype are the only ones that form efficient crosslinks with TonB-Q160. Perhaps the contacts of TonB-Q160 with BtuB positions 8 and 10 signal to TonB whether the transporter has bound substrate. Changes of backbone conformation in the mutants might distort that interaction so that TonB does not recognize that receptor as a suitable target. Alternatively, these amino acid substitutions in the TonB-box might prevent or dissipate the transmission of force from TonB that would normally result in substrate transport. Sequence changes at adjoining residues can shift the conformation back so that TonB can now function. Future studies should address the identification of other contact sites of TonB and the transporters, the structural consequences of the uncoupling mutations, and the structure of the TonB-BtuB complex.
Acknowledgments
The authors are indebted to Cindy Fuller for helpful comments and to Kathleen Postle and Cindy Fuller for their generous provision of the monoclonal antibodies used in this study. This work was supported by research grant GM19078 from the National Institute of General Medical Sciences.
References
Articles from Proceedings of the National Academy of Sciences of the United States of America are provided here courtesy of National Academy of Sciences
Full text links
Read article at publisher's site: https://doi.org/10.1073/pnas.96.19.10673
Read article for free, from open access legal sources, via Unpaywall:
https://europepmc.org/articles/pmc17941?pdf=render
Citations & impact
Impact metrics
Citations of article over time
Smart citations by scite.ai
Explore citation contexts and check if this article has been
supported or disputed.
https://scite.ai/reports/10.1073/pnas.96.19.10673
Article citations
Leptothrix ochracea genomes reveal potential for mixotrophic growth on Fe(II) and organic carbon.
Appl Environ Microbiol, 90(9):e0059924, 12 Aug 2024
Cited by: 0 articles | PMID: 39133000 | PMCID: PMC11412304
Multiple TonB homologs are important for carbohydrate utilization by Bacteroides thetaiotaomicron.
J Bacteriol, 205(11):e0021823, 24 Oct 2023
Cited by: 2 articles | PMID: 37874167 | PMCID: PMC10662123
Multi-environment ecogenomics analysis of the cosmopolitan phylum Gemmatimonadota.
Microbiol Spectr, e0111223, 21 Sep 2023
Cited by: 7 articles | PMID: 37732776 | PMCID: PMC10581226
HDX-MS performed on BtuB in E. coli outer membranes delineates the luminal domain's allostery and unfolding upon B12 and TonB binding.
Proc Natl Acad Sci U S A, 119(20):e2119436119, 12 May 2022
Cited by: 13 articles | PMID: 35549554 | PMCID: PMC9171809
Functional Diversity of TonB-Like Proteins in the Heterocyst-Forming Cyanobacterium Anabaena sp. PCC 7120.
mSphere, 6(6):e0021421, 17 Nov 2021
Cited by: 4 articles | PMID: 34787445 | PMCID: PMC8597729
Go to all (106) article citations
Data
Similar Articles
To arrive at the top five similar articles we use a word-weighted algorithm to compare words from the Title and Abstract of each citation.
Sequence changes in the ton box region of BtuB affect its transport activities and interaction with TonB protein.
J Bacteriol, 182(21):5954-5961, 01 Nov 2000
Cited by: 51 articles | PMID: 11029413 | PMCID: PMC94727
Mutual inhibition of cobalamin and siderophore uptake systems suggests their competition for TonB function.
J Bacteriol, 177(17):4829-4835, 01 Sep 1995
Cited by: 46 articles | PMID: 7665457 | PMCID: PMC177254
Substrate-induced exposure of an energy-coupling motif of a membrane transporter.
Nat Struct Biol, 7(3):205-209, 01 Mar 2000
Cited by: 62 articles | PMID: 10700278
Vitamin B12 transport in Escherichia coli: energy coupling between membranes.
Mol Microbiol, 4(12):2027-2033, 01 Dec 1990
Cited by: 148 articles | PMID: 2089218
Review
Funding
Funders who supported this work.
NIGMS NIH HHS (3)
Grant ID: R01 GM019078
Grant ID: F32 GM019078
Grant ID: GM19078