Abstract
Free full text

Severe Acute Respiratory Syndrome (SARS) Coronavirus-Induced Lung Epithelial Cytokines Exacerbate SARS Pathogenesis by Modulating Intrinsic Functions of Monocyte-Derived Macrophages and Dendritic Cells
Abstract
Severe acute respiratory syndrome (SARS), which is caused by a novel coronavirus (CoV), is a highly communicable disease with the lungs as the major pathological target. Although SARS likely stems from overexuberant host inflammatory responses, the exact mechanism leading to the detrimental outcome in patients remains unknown. Pulmonary macrophages (M), airway epithelium, and dendritic cells (DC) are key cellular elements of the host innate defenses against respiratory infections. While pulmonary M
are situated at the luminal epithelial surface, DC reside abundantly underneath the epithelium. Such strategic locations of these cells within the airways make it relevant to investigate their likely impact on SARS pathogenesis subsequent to their interaction with infected lung epithelial cells. To study this, we established highly polarized human lung epithelial Calu-3 cells by using the Transwell culture system. Here we report that supernatants harvested from the apical and basolateral domains of infected Calu-3 cells are potent in modulating the intrinsic functions of M
and DC, respectively. They prompted the production of cytokines by both M
and DC and selectively induced CD40 and CD86 expression only on DC. However, they compromised the abilities of the DC and M
in priming naïve T cells and phagocytosis, respectively. We also identified interleukin-6 (IL-6) and IL-8 as key SARS-CoV-induced epithelial cytokines capable of inhibiting the T-cell-priming ability of DC. Taken together, our results provide insights into the molecular and cellular bases of the host antiviral innate immunity within the lungs that eventually lead to an exacerbated inflammatory cascades and severe tissue damage in SARS patients.
Severe acute respiratory syndrome (SARS) was originally identified as an atypical pneumonia emerging from southern China in late 2002 (15, 27). This highly contagious respiratory disease, with an 8 to 15% mortality rate, rapidly spread to other countries within Asia and to other continents, causing devastating social, economic, and medical impact worldwide. Individuals severely affected by SARS have had clinical manifestations characterized by fever, dry cough, lymphopenia, various degrees of pancytopenia, arterial hypoxemia, and rapidly progressing changes in chest radiography (15). Through an intense international effort, the causative agent of SARS has been identified as a novel coronavirus (CoV), designated SARS-CoV (7, 15, 27, 34). The transmission of this deadly virus is thought to be mediated primarily through virus-laden droplets but also via either small-particle aerosol or fecal-oral routes, with the lungs as its main pathological target.
The exact mechanism of SARS pathogenesis remains unknown. However, pathological examinations of samples obtained from patients who died of SARS revealed diffuse alveolar damage and morphological changes at various stages and of various degrees of severity, accompanied by prominent hyperplasia of pulmonary epithelial cells and presentation of activated alveolar and interstitial macrophages (M). Strikingly, these pulmonary manifestations were usually found after clearance of viremia and in the absence of other opportunistic infections, findings which suggest that intense local inflammatory responses could be responsible, at least in part, for the profound pulmonary pathology. The likelihood that SARS stems from exuberant inflammatory responses is also supported by the detection of the reactive hemophagocytic syndrome, a disease caused by cytokine dysregulation, in the lungs of severely affected patients (15).
The lungs constitute a portal of entry for various respiratory pathogens, and, fortunately, evolution has equipped this vital organ with elaborate host defense systems to maintain its sterility and normal respiratory functions. Epithelium, pulmonary M, and dendritic cells (DC) are three key cellular elements of the airway innate immune system. In addition to functioning as physical and mechanical barriers that separate and eliminate many inhaled materials, lung epithelial cells can directly respond to respiratory infection by secreting various molecules to initiate and sustain cascades of inflammatory responses that ultimately influence the development of adaptive immune responses required to sterilize the infection (24, 28). Although this early epithelial response is beneficial in facilitating pathogen clearance, an unregulated and excessive epithelial response can also lead to exacerbated inflammatory responses, causing severe tissue damage (37). It is well established that M
and DC not only are critically involved in mediating acute inflammatory responses but also are central players in bridging innate and adaptive immunity against microbial infections (3, 30). Importantly, the majority of M
and DC reside at sites where the tissue and environment interface and most infections occur (3, 30). In this regard, the great majority of pulmonary M
and DC are located abundantly in the alveolar spaces and areas beneath the epithelial lining of the airways, respectively. Such unique anatomic locations within the airway respiratory system, along with their intrinsic functions, i.e., phagocytosis, antigen processing and presentation, and cytokine production, make them, together with epithelial cells, among the first and key responders to respiratory infections.
We have previously characterized the interaction between SARS-CoV and pathologically relevant human lung epithelial cells, primary human M, or DC with regard to the infectivity and polarity of viral infection and their subsequent effect on cellular functions (45, 46). In the present study, we sought to characterize the impact of interplay among these three crucial cellular elements of the airway innate immune system on SARS pathogenesis. Lung epithelial cells are highly specialized cells having well-organized tight-junction complexes on their plasma membrane, thereby separating the surface of the cells into two functionally distinct domains, the apical and basolateral domains, essential for executing their physiological functions (2, 5). Thus, to study this under more physiologically relevant conditions, we first established highly polarized human bronchial epithelial Calu-3 cells by using the Transwell culture system (46) and then followed that process by infection with SARS-CoV. We next determined the profile and the polarity of inflammatory cytokines produced by infected Calu-3 cells and investigated how these virally induced epithelial cytokines would affect the intrinsic functions of M
and DC. Here, we show that Calu-3 cells responded to SARS-CoV infection by secreting several inflammatory cytokines, including interleukin-6 (IL-6), IL-8 and gamma interferon (IFN-γ)-inducible protein 10 (IP-10), in a delayed and dose-dependent manner. We also demonstrate that these SARS-CoV-induced Calu-3 cytokines are potent in modulating various functions of M
and DC, including cytokine production, phagocytosis, and activation of naïve T cells. Finally, we also identify IL-6 and IL-8 as the likely cytokines exerting a negative impact on the ability of DC to priming naïve T cells, thereby providing an intriguing mechanism for SARS-CoV to evade host immune responses by delaying or even diminishing the development of adaptive immune responses. Our results may provide the cellular and molecular bases of the exacerbated inflammatory responses and severe tissue damage within the lungs of SARS patients.
MATERIALS AND METHODS
Cells.
Vero E6 cells were grown in modified Eagle's minimal essential medium supplemented with 10% fetal bovine serum, designated M-10 medium, whereas human lung epithelial Calu-3 cells (ATCC) were grown in modified Eagle's minimal essential medium supplemented with 20% FCS (M-20 medium), as previously described (46).
SARS-CoV.
The Urbani strain of SARS-CoV, kindly provided to us by T. G. Ksiazek, Centers for Disease Control and Prevention (Atlanta, GA), was used throughout this study. The original stock of SARS-CoV was subjected to two additional passages in Vero E6 cells. A viral stock with a titer of 1 × 107 50% tissue culture infectious doses (TCID50)/ml was generated and stored at −80°C. All experiments involving infectious virus were conducted at the University of Texas Medical Branch in an approved biosafety level 3 laboratory.
Viral infection of highly polarized Calu-3 cell cultures.
Highly polarized Calu-3 cells were established by seeding cells onto semipermeable membrane inserts (Transwell no. 3470-clear; Corning Inc., Corning, NY) and culturing for an additional 10 to 12 days after they reached confluence. The latter method ensured the formation of tight junctions between adjacent cells, as evidenced by expression of a relative constant value of transepithelial resistance, which was monitored by an epithelial volt-ohm meter in an electric cell-substrate impedance sensing system (Applied Biophysics, Troy, NY). Only those highly differentiated Calu-3 cell monolayers showing a transepithelial resistance of 1,800 ± 200 ohm-cm2 were used in our studies.
Polarized Calu-3 cell cultures were infected through the apical domain with either gamma-inactivated or live SARS-CoV at a multiplicity of infection (MOI) of 1 by established protocols (45). Supernatants were harvested from both the apical and basolateral sides at day 3 after infection for determining the profile and polarity of the inflammatory cytokines and progeny virus by using the Bio-Plex Cytometric Bead Array (Bio-Rad) or enzyme-linked immunosorbent assay (ELISA) and the TCID50 assay, respectively.
Viral titration.
The infectious viral titers in the cell-free supernatants collected at the indicated time points postinfection were determined by a standard TCID50 assay on permissive Vero E6 cells, as described previously (45). The titer of individual samples was expressed as TCID50 per ml of sample.
M
and DC.
Primary human M and DC were prepared from highly enriched peripheral monocytes (MO). Briefly, peripheral blood mononuclear cells (PBMC) were purified from the peripheral blood of healthy individuals by Ficoll-Paque gradients (American Biosciences). CD14+ MO were purified from PBMC by negative selection by using the combination of an antibody (Ab) mixture for the enrichment of human MO and a magnetic column separation system (StemCell Technologies), as previously described (42). We routinely obtained >95% purity of CD14+ MO, as assessed by flow cytometry, and followed a well-established protocol to generate MO-derived M
and DC, as described elsewhere (1, 33, 49).
Cocultivation of M
and DC with SARS-CoV-infected Calu-3 culture supernatants.
Cell-free supernatants, harvested from either side of highly polarized Calu-3 cells grown in a Transwell system, were subjected to gamma irradiation to inactivate the infectious virus. These gamma-inactivated samples were used for assessing their cytokine contents in a biosafety level 2 laboratory. Primary human M and DC were subsequently incubated with 50% of the basolateral and apical supernatants derived from either uninfected or infected polarized Calu-3 cells, respectively, for 3 days before assessing the potential of Calu-3 epithelial cytokines to modulate their intrinsic functions.
Phenotyping of M
and DC.
The expression of CD40, CD86, CD83, and HLA-DR molecules on the surfaces of M and DC was determined with the standard FACScan and CellQuest software (BD Bioscience). Specific Abs to the aforementioned molecules and their isotype-matched control Abs were obtained from Caltag Laboratories.
Evaluation of DC-mediated proliferation of naïve CD4+ T cells.
The CD4+ T cells were purified by negative selection from PBMC by using a combined mixture of Abs designed for enriching human CD4+ T cells and a magnetic column (StemCell Technology), as previously described (43). DC were cultured with basolateral supernatants derived from either uninfected or SARS-CoV-infected Calu-3 cells for 3 days before assessing their T-cell priming capacity. Additionally, DC stimulated with lipopolysaccharide (LPS) (5 μg/ml) or infected with live SARS-CoV (MOI = 1) for a total of 2 and 3 days, respectively, were included as positive controls (45). In some experiments aliquots of DC were cultured with basolateral supernatants of infected Calu-3 cells containing excess amounts of neutralizing anti-IL-6 (500-P26; PeproTech, Rocky Hill, NJ), anti-IL-8 (500-P28; PeproTech), anti-IP-10 (500-P93; PeproTech), or irrelevant Abs as controls. Alternatively, aliquots of DC were cultured with predetermined concentrations of recombinant human IL-6 (200-06; PeproTech), IL-8 (200-08 M; PeproTech), or IP-10 (300-12, PeproTech) for 3 days before assessing their T-cell-priming capacity. Briefly, a fixed number of allogeneic CD4+ T cells (1 × 105/well) were cocultured with differentially treated DC at various T/DC ratios in 96-well microtiter plates for 6 days. Triplicate cultures of each DC/T ratio were pulsed with 1 μCi/well [H3]thymidine (New England Nuclear) for the last 12 to 16 h before assessing the proliferative T-cell responses. The total incorporation of [H3]thymidine was determined by liquid scintillation counting and expressed as counts per minute.
Cytokine profiling.
The amounts of cytokines in the supernatants from different cultures of Calu-3 cells, M, and DC were assessed by the Bio-Plex Cytometric Bead Array (Bio-Rad), according to the manufacturer's instructions. In some experiments, the contents of IL-6 and IL-8 in the samples were evaluated by using a standard ELISA, as previously described (43, 44). Both capture monoclonal Ab (MAb) and biotinylated detection MAb for human IL-6 and IL-8 were purchased from BD Pharmingen and used at the concentrations recommended by the manufacturer. The concentrations of individual cytokines were expressed as the mean ± standard deviation (SD) from duplicate samples.
Assessment of phagocytosis by flow cytometry.
Pellets of differentially treated M (2 × 105 cells/pellet) were resuspended in 500 μl of R-10 medium in 1.5-ml Eppendorf tubes containing fluorescein isothiocyanate (FITC)-dextran (molecular weight = 40,000, 40 μg/ml; Invitrogen) and incubated at 37°C (or at 4°C for the control) for 1 h. The cells were washed five times with cold R-10 medium and fixed with 2% paraformaldehyde, and the amount of the accumulated intracellular fluorescent probe was determined using a FACScan flow cytometer (BD Bioscience).
Immunoblot analysis.
At various time points postinfection, cells were lysed on ice in a buffer containing 25 mM Tris-HCl (pH 7.5), 150 mM NaCl, 1% Triton X-100, and protease inhibitor cocktail (Sigma). Lysed cells were transferred to microcentrifuge tubes and immediately frozen and stored at −80°C. Cell lysates were gamma irradiated, cleared by centrifugation, and quantified using the bicinchoninic acid protein assay (Bio-Rad). Equal amounts of protein samples were separated by sodium dodecyl sulfate-polyacrylamide gel electrophoresis, transferred to nitrocellulose membranes, and subjected to immunoblot analysis as described previously (6, 20). The following Abs were used: antiactin MAb (Sigma), rabbit anti-IκBα polyclonal Ab (PAb) (Santa Cruz), rabbit anti-SARS-CoV nucleocapsid PAb (Imgenex), and peroxidase-conjugated secondary anti-mouse and anti-rabbit PAbs (Southern Biotech). Protein bands were visualized by incubation with enhanced chemiluminescent substrates (GE Healthcare), followed by exposure to X-ray films.
Statistical analysis.
Data presented are means ± SDs. In addition, wherever appropriate, Student's t test and a one-way analysis of variance with Bonferroni's multiple-comparison test were used, as indicated, to establish statistical significance between groups. A significant difference between two groups was considered to exist when the P value was either <0.05 or <0.01.
RESULTS
Kinetics of NF-κB-mediated IL-6 and IL-8 production by SARS-CoV-infected human epithelial Calu-3 cells.
To initially determine the inflammatory potential of Calu-3 cells in response to SARS-CoV, confluent Calu-3 cells grown in six-well plates were either mock infected or infected with SARS-CoV at MOIs of 5, 1, and 0.1 for 4 days with M-20 medium before assessing the contents of IL-6 and IL-8 in the supernatants by an ELISA. Despite their ability to spontaneously produce inflammatory cytokines (Fig. (Fig.1A),1A), Calu-3 cells responded to SARS-CoV infection by releasing, in a dose-dependent manner, significantly elevated amounts of both IL-6 and IL-8. We next investigated the kinetics of the cytokine responses by infecting Calu-3 cells with medium (as a mock-infected control) or live or gamma-inactivated SARS-CoV at an MOI of 0.1. The levels of IL-6 expression in the cultures were assessed daily for a total of 4 days. As shown in Fig. Fig.1B,1B, Calu-3 cells exposed to inactivated SARS-CoV failed to produce IL-6 beyond the level detected from uninfected Calu-3 cells. Although live SARS-CoV-infected Calu-3 cells could increase IL-6 production, a significantly enhanced production of this cytokine was not detected until day 4 after infection (P < 0.01). We have shown that the yields of infectious progeny SARS-CoV by permissive Calu-3 cells reached a maximum at day 2 after infection (46), and the magnitude of SARS-CoV-induced inflammatory responses was positively correlated with the extent of viral replication (41). Thus, we determined the molecular basis of such a delay in the cytokine responses by investigating the kinetics of NF-κB activation in infected Calu-3 cells. As shown in Fig. Fig.1C,1C, despite the intense expression of SARS-CoV nucleocapsid protein at 24 and 48 h, a significantly activated NF-κB pathway, as indicated by the degradation of IκB, in Calu-3 cells was not detected until 72 h after infection. Taken together, these results strongly suggest that Calu-3 cells are capable of mounting a dose- and time-dependent and NF-κB-mediated production of inflammatory cytokines in response to SARS-CoV infection.
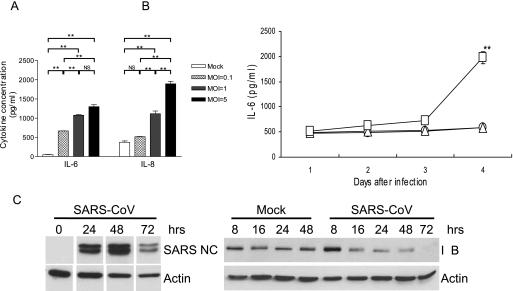
SARS-CoV induces a dose- and time-dependent production of IL-6 and IL-8 by infected human bronchial Calu-3 cells. (A) Confluent Calu-3 cells grown in six-well plates with M-20 medium were infected with live SARS-CoV at MOIs of 5, 1, and 0.1 or, as a control, remained uninfected. The infected cultures were maintained in the M-20 medium before cell-free supernatants were harvested at day 4 after infection for assessing the IL-6 and IL-8 contents by ELISA. (B) Confluent Calu-3 cells were infected with live SARS-CoV (□) or gamma-inactivated (2 × 106 rads) SARS-CoV () at an MOI of 0.1 or remained uninfected (○) as control. The infected cultures were maintained with the M-20 medium and harvested at days 1, 2, 3, and 4 after infection, and their IL-6 content was assessed by ELISA. (C) Confluent CaLu-3 cells were infected with SARS-CoV (MOI = 0.1) for the indicated times before cellular lysates were extracted for immunoblotting for SARS-CoV nucleocapsid (NC) protein, IκB, and actin (as control), as described in Materials and Methods. Results are representative of at least three independent experiments. *, P ≤ 0.05; **, P ≤ 0.01 (Student's t test) compared to different MOIs (A) or gamma-inactivated virus-infected controls (B). Error bars indicate SDs.
Profiling and polarity of inflammatory secretion of SARS-CoV-infected Calu-3 cells.
Lung epithelial cells are structurally and functionally polarized in vivo (10). To investigate the cytokine responses under more physiologically relevant conditions, we characterized the profile and the polarity of SARS-CoV-induced inflammatory responses by infecting polarized Calu-3 cells grown on membrane inserts with gamma-inactivated or live SARS-CoV (MOI = 1) through the apical domain, as we have previously described (46). Supernatants were harvested separately from apical and basolateral chambers at day 4 after infection for assessing the yield of infectious progeny virus and the profile and the polarity of inflammatory responses. While a titer of ~106 TCID50/ml of infectious virus was routinely recovered from the apical supernatants, only very low virus titers (~102 TCID50/ml) were detected in the basolateral supernatants of infected cultures, confirming the preferential apical-to-apical route of SARS-CoV infection and spread in lung epithelial cells (46). Results of Bio-Plex analysis revealed that Calu-3 cells exposed to gamma-inactivated SARS-CoV released small, but readily detectable, amounts of IL-6, IL-8, and IP-10, along with trace levels of IFN-γ and granulocyte colony-stimulating factor (G-CSF), into both the apical and basolateral chambers of the cultures (data not shown). However, the ability of Calu-3 cells to produce inflammatory mediators was significantly enhanced upon infection with live viruses. Table Table11 shows the polarity and the net gain of the aforementioned cytokines produced by live virus-infected Calu-3 cells, which were derived after subtracting those released by inactivated, virus-exposed Calu-3 cells. Interestingly, while IL-6 was preferentially released through the apical surface, IL-8, IP-10, and other cytokines were secreted through either side of the polarized Calu-3 cells. Other cytokines that could be simultaneously measured by Bio-Plex analysis, including IL-2, IL-4, IL-5, IL-7, IL-10, IL-12p70, IL-13, IL-17, granulocyte-macrophage colony-stimulating factor, tumor necrosis factor alpha (TNF-α), monocyte chemoattractant protein 1 (MCP-1), and macrophage inflammatory protein 1B (MIP-1β), could not be detected in either inactivated or live virus-exposed Calu-3 cultures.
TABLE 1.
Polarity of SARS-CoV-induced cytokine secretion by infected Calu-3 cellsa
Cytokine | Mean concn (pg/ml) ± SDb after infection with:
| |||
---|---|---|---|---|
Gamma-inactivated virus
| Live virus
| |||
Apical | Basolateral | Apical | Basolateral | |
IL-6 | 562 ± 188 | 143 ± 40 | 4,710 ± 312 | 392 ± 35 |
IL-8 | 202 ± 181 | 88 ± 15 | 1,363 ± 179 | 792 ± 124 |
IP-10 | 45 ± 23 | 66 ± 35 | 285 ± 38 | 437 ± 29 |
IFN-γ | 3 ± 2 | 2 ± 1 | 24 ± 4 | 37 ± 8 |
G-CSF | <1 | <1 | 4 ± 1 | 7 ± 3 |
SARS-CoV-induced Calu-3 cell cytokines are potent in promoting cytokine production by primary M
and DC.
The deteriorating health that is the outcome of SARS infection likely stems from unregulated and often excessive acute inflammatory responses within the lungs (19, 26). However, neither the cellular nor the molecular basis of such exacerbated inflammatory responses associated with SARS-CoV infection has been characterized. Because pulmonary epithelial cells are considered to be among the earliest targets of SARS-CoV infection, it is likely that acute cytokines elicited by these early target cells might play a pivotal role in initiating inflammatory cascades during the progression of disease. Pulmonary M and DC reside abundantly within the alveoli and in areas beneath the epithelial lining, respectively. Such a close anatomic association with airway epithelium and their well-established roles in bridging innate and adaptive immune responses prompted us to determine whether M
and DC, two of the most prominent innate immune cells, are capable of relaying and/or amplifying the acute inflammatory response initiated by infected lung epithelial cells. We used human MO-derived M
and DC as alveolar M
and pulmonary DC surrogates in this study. Briefly, M
and DC were cultured with 50% of gamma-irradiated apical and basolateral supernatants, respectively, for a total of 3 days before assessing their abilities to produce inflammatory cytokines and chemokines by Bio-Plex analysis. As shown in Fig. Fig.2,2, the apical supernatants harvested from the infected Calu-3 cells were more potent than those derived from mock-infected Calu-3 cells in promoting cytokine production by cultured M
, resulting in a significantly enhanced production of IL-1β, G-CSF, MIP-1α/β, and TNF-α (Fig. (Fig.2A)2A) and IL-6 (Fig. (Fig.2B)2B) (P < 0.05). When DC were cultured with the basolateral supernatants, we found that infected Calu-3 supernatants were also more potent than those derived from uninfected controls in promoting the production of IL-12p40, MIP-1α, and IFN-γ (Fig. (Fig.3A)3A) and IL-6, IL-8, and MCP-1 (Fig. (Fig.3B)3B) by DC.
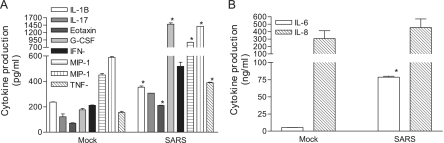
Apical supernatants derived from SARS-CoV-infected Calu-3 cells are potent in promoting cytokine production by M. MO-derived M
were incubated for 3 days with 50% of gamma-inactivated apical supernatants harvested from either mock- or SARS-CoV-infected Calu-3 cells grown on the membrane inserts. The resulting cell-free supernatants were subjected to cytokine profiling by using the Bio-Plex Cytometric Bead Array assay. Data presented are the net values for the indicated cytokines produced by cultured M
, which were calculated by subtracting the baseline values existing in the apical supernatants harvested from those of infected Calu-3 cells. Data presented are means ± SDs. Results are representative of three independent experiments using M
derived from different individuals. *, P ≤ 0.05 (Student's t test) compared to those cultured with mock-infected supernatants.
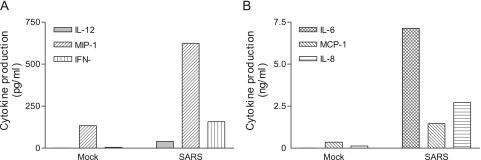
Basolateral supernatants harvested from SARS-CoV-infected Calu-3 cells are potent in stimulating cytokine production by DC. MO-derived DC were cultured with 50% of gamma-inactivated supernatants harvested from the basolateral chambers of either mock- or SARS-CoV-infected Calu-3 cells, as described for Fig. Fig.2,2, for a total of 3 days. The resulting supernatants were subjected to cytokine profiling by Bio-Plex analysis. Data presented are the net values for the indicated cytokines produced by cultured DC, which were calculated by subtracting the baseline values of the cytokines from those existing in the basolateral supernatants of infected Calu-3 cells. Results are representative of three independent experiments using DC derived from different individuals.
Cytokines released by SARS-CoV-infected Calu-3 cells induce the expression of CD40 and CD86 on DC but not M
.
The efficacy of M, and especially that of DC, in mediating host immune responses against invading pathogens relies, in part, on the expression of costimulatory molecules on their surfaces, where an increased expression of these molecules usually correlates well with an enhanced capacity of priming specific cell-mediated immunity (4, 21). We investigated whether SARS-CoV-induced Calu-3 cell cytokines could regulate the expression of costimulatory molecules on M
and DC. For these experiments, primary M
and DC were cultured for three days in medium containing 50% of the supernatants harvested from infected and mock-infected Calu-3 cells, as described above for cytokine profiling. The resulting cells were analyzed by flow cytometry for their expression of CD40, CD86, HLA-DR, and CD83, a marker of mature DC. We found that upon cultivation with the basolateral supernatants of virally infected, but not uninfected, Calu-3 cells, the expression of CD40 and CD86 molecules on the surface of DC was enhanced (Fig. (Fig.4).4). However, no significant change in the expression of HLA-DR and CD83 on DC could be observed (data not shown). We have previously shown that DC exposed to gamma-inactivated SARS-CoV at an MOI of 1 or higher did not reveal any change in the expression of costimulatory molecules on the surface (45). The ability of the basolateral supernatants that contained ~102 TCID50/ml of inactivated SARS-CoV particles to modulate the expression of selected costimulatory molecules on DC is thus likely mediated through SARS-CoV-induced Calu-3 cell cytokines. However, we were unable to detect any significant change in the expression of the aforementioned molecules on the surface of M
cultured with the apical supernatants containing ~5 × 105 TCID50/ml of inactivated SARS-CoV (data not shown). These results suggest that SARS-CoV-induced Calu-3 cell cytokines have the capacity to modulate the expression of costimulatory molecules in a selective and cell type-specific manner.
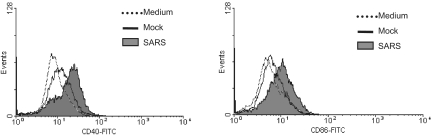
SARS-CoV-induced epithelial Calu-3 cell cytokines enhance the expression of CD40 and CD86 molecules on the surface of cultured DC. MO-derived DC were subjected to incubation with 50% of basolateral supernatants derived from either mock- or SARS-CoV-infected Calu-3 cells for 3 days. DC cultured in RPMI 1640-10% FCS medium were included as an additional control. The expression of costimulatory molecules was evaluated by fluorescence-activated cell sorter analysis. Data presented are the histogram plots from fluorescence-activated cell sorter analysis of cultured DC, showing the enhanced expression of CD40 and CD86. Results presented are representative of four independent experiments using DC derived from different individuals.
SARS-CoV-induced Calu-3 cell cytokines inhibit the receptor-mediated endocytosis and T cell-priming activity of M
and DC, respectively.
The ability to capture, process, and subsequently present antigenic peptides to antigen-specific T cells is another characteristic feature of M and DC (4, 40). To investigate whether SARS-CoV-induced Calu-3 cell cytokines could modify these crucial functions of M
and DC, we evaluated the ability of M
and DC that had been cultured for 3 days with appropriate supernatants derived from uninfected or SARS-CoV-infected Calu-3 cells to take up antigens and prime the proliferation of naïve T-cell responses, respectively. Because receptor-mediated endocytosis is one of the most efficient mechanisms for antigen uptake (17), we compared mannose receptor-mediated uptake of FITC-conjugated dextran (molecular weight = 40,000) in differentially treated M
. As shown in Fig. Fig.5,5, cultivation of M
with apical supernatants derived from infected Calu-3 cells resulted in a complete loss of the ability to take up FITC-dextran sulfates, as judged by the reduced values of the mean fluorescence intensity. Specifically, M
cultured with supernatants of infected and uninfected Calu-3 cells and RPMI 1640-10% FCS medium alone had mean fluorescence intensities of 10.1, 17.2, and 11.9, respectively.
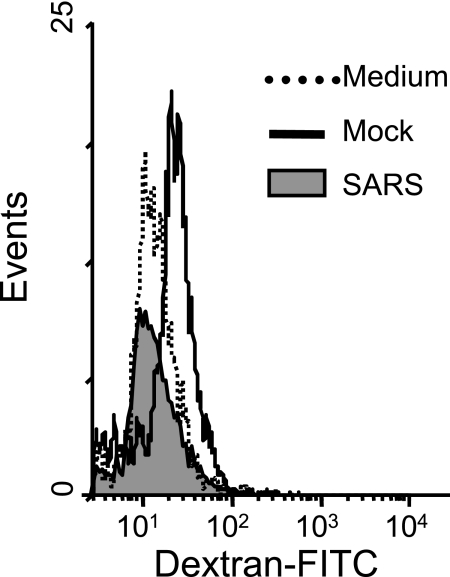
Apical supernatant harvested from SARS-CoV-infected Calu-3 cells reduces the phagocytic activity of M. M
cultured with the apical supernatants derived from mock- or SARS-CoV-infected Calu-3 cells, or RPMI 1640-10% FCS medium, for 3 days, as described for Fig. Fig.2,2, were harvested, and their capacity to take up FITC-conjugated dextran sulfate was analyzed by fluorescence-activated cell sorter analysis as described in Materials and Methods. Results are representative of three independent experiments using M
derived from different individuals.
The capacity to activate naïve T cells is a unique feature of mature DC. To investigate whether this unique ability of DC could be modulated upon cultivation with SARS-CoV-induced Calu-3 cell cytokines released into the basolateral chambers, we compared differentially treated DC populations for their ability to stimulate proliferation of naïve allogeneic CD4+ T cells by using the standard mixed-lymphocyte reaction (MLR) assay. We also included DC that had been either cultured with LPS (2.5 μg/ml) or infected with live SARS-CoV (MOI = 1) as positive controls. As shown in Fig. Fig.6,6, LPS-treated or live SARS-CoV-infected DC appeared to be potent in stimulating the proliferation of naïve T cells compared to untreated controls, an observation consistent with our earlier report (45). In contrast, DC cultured with the basolateral supernatants of infected Calu-3 cells had a severely compromised ability to stimulate naïve T cells compared to those cultured with uninfected basolateral supernatants. Overall, these results suggest that SARS-CoV-induced Calu-3 cytokines could actively downregulate the ability of DC to prime naïve T cells, thereby posing a real threat in delaying and/or diminishing the onset of adaptive immune responses against SARS-CoV infection.
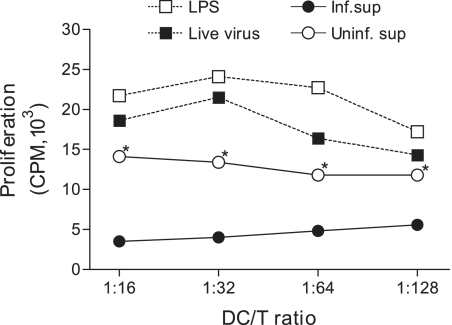
The intrinsic T-cell-priming ability of DC is significantly suppressed subsequent to their cultivation with the basolateral supernatant derived from SARS-CoV-infected, but not mock-infected, Calu-3 cells. Aliquots of MO-derived DC were subjected to activation with LPS (5 μg/ml) or live SARS-CoV (MOI = 1) or cultivation with 50% of the basolateral supernatants harvested from mock- or SARS-CoV-infected Calu-3 cells for a total of 3 days. These differentially treated DC were evaluated for their capacity to prime naïve CD4+ T cells in the standard one-way MLR assay, as described in Materials and Methods. The proliferation of allogeneic T cells was expressed as the average cpm ± SD for triplicate samples. Data shown are representative of three independent experiments using DC and naïve T cells derived from different individuals *, P < 0.05 from Student's t test, in which we compared culture with supernatants derived from infected versus mock-infected Calu-3 cells.
IL-6 and IL-8 are key SARS-CoV-induced epithelial cytokines responsible, in part, for the compromised T-cell-priming ability of DC.
IL-6, IL-8, and IP-10 are the major cytokines elicited by infected Calu-3 cells (Table (Table1).1). While IL-6 has been shown to impair the capacity of DC to activate T-cell responses, likely through the induction of IL-10 and the suppression of NF-κB activation (9), little is known about the role that IL-8 or IP-10 might play in regulating DC functions. Having shown the negative impact of SARS-CoV-induced Calu-3 cell cytokines on the ability of DC to activate naïve T cells, we sought the identity of the responsible cytokines by exploiting the possible role of IL-6, IL-8, and IP-10 in compromising the T-cell-priming activity of DC. Two different, but complementary, strategies were employed by using neutralizing Abs and recombinant cytokines, respectively, as described in Materials and Methods. Briefly, aliquots of DC were incubated with the basolateral supernatants of infected Calu-3 cells in the presence of an excess amount of irrelevant Ab (as a control) or neutralizing anti-IL-6, -IL-8, or -IP-10 Abs. Additionally, aliquots of DC were cultured with M-10 medium alone (as a control) or M-10 medium containing recombinant IL-6, recombinant IL-8, or recombinant IP-10 at concentrations equivalent to those existing in 50% of basolateral supernatants derived from infected Calu-3 cells. DC were harvested at day 3 after cultivation for assessing their capacities to prime naïve T cells by using the standard MLR. The potential role of targeted cytokines in modulating the T-cell-priming ability of DC was assessed by comparing the total uptakes of [3H]thymidine by T cells cocultured with differentially treated DC. To quantitatively compare the impact of these cytokines on this unique function of DC, the averages of [3H]thymidine uptakes by triplicate wells of T cells stimulated with DC cultured with M-10 medium and basolateral supernatants derived from SARS-CoV-infected Calu-3 cells were arbitrarily assigned as 100% and 0% of T-cell proliferation, respectively. As shown in Fig. Fig.7A,7A, incubation of DC with supernatants derived from infected Calu-3 cells in the presence of control Abs resulted in a complete loss of their ability to activate naïve T cells. In contrast, DC cultured with IL-6-, IL-8-, or IP-10-deprived Calu-3 cell supernatants, via incubation with their respective neutralizing Abs, recovered an average of 80% (P < 0.05), 45%, and 18%, respectively, of their capacity to activate T cells. These results suggest that IL-6 and possibly IL-8, but not IP-10, may be responsible, at least in part, for the diminishing ability of DC to activate naïve T cells. This notion was supported by the data from subsequent studies showing that DC cultured in M-10 medium containing recombinant IL-6 or recombinant IL-8, but not recombinant IP-10, had a significantly compromised ability to stimulate the proliferation of T cells (P < 0.05, Fig. Fig.7B7B).
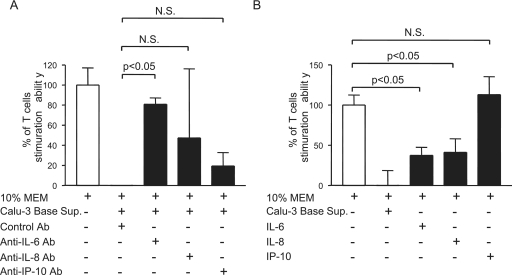
IL-6 and IL-8 are crucial SARS-CoV-induced Calu-3 cell cytokines responsible, in part, for inhibiting the ability of DC to prime naïve T cells. (A) Aliquots of DC were incubated with M-10 medium alone or 50% of SARS-CoV-induced Calu-3 cell cytokines in the presence of control Ab and specific neutralizing Abs against IL-6, IL-8, or IP-10. (B) Additionally, they were incubated with appropriate concentrations of recombinant IL-6, recombinant IL-8, or recombinant IP-10. After cultivation for 3 days, DC were harvested to assess their ability to stimulate proliferation of naïve T cells in a standard MLR. The capacity of differentially treated DC is presented as the percentage of that elicited by M-10 medium-cultured DC. One-way analysis of variance with Bonferroni's multiple-comparison test was used to determine the level of statistical significance. Data presented are representative of at least two independent experiments. Error bars indicate SDs.
DISCUSSION
SARS has established itself as a contagious human respiratory disease. While the extensive pulmonary damage observed in patients severely affected by SARS could be caused directly by viral destruction of permissive alveolar and bronchial epithelial cells, the prominent presentation of pulmonary infiltrates in the acute and/or late phase of SARS-CoV infection suggests that SARS could also stem from an excessive host immune response that eventually leads to diffuse alveolar damage and fibrosis (19, 26). To investigate how the prospective cellular response during the initial phase of host-virus interaction would result in exacerbated inflammatory responses in SARS patients, we first characterized how the permissive human bronchial Calu-3 cells, the only human lung epithelial cell line naturally permissive to SARS-CoV (46), would respond to SARS-CoV infection with regard to the production of cytokines. We found that, despite the absence of type I IFN secretion (unpublished data), SARS-CoV-infected Calu-3 cells are capable of secreting moderate levels of IL-6 and IL-8 in a dose- and time-dependent manner (Fig. (Fig.1).1). The exact mechanism remains to be determined with respect to the delay in the activation of NF-κB and thus of IL-6 and IL-8 responses by infected Calu-3 cells, relative to the kinetics of viral replication. However, virally induced lysis of Calu-3 cells may not be directly responsible for the enhanced cytokine responses at day 4 postinfection, as we have shown that SARS-CoV-infected Calu-3 cells cultured with medium containing 20% FCS failed to show any microscopic lesion or cytopathic effect until day 8 postinfection (46). The early enhanced expression of IL-8 and IP-10 has been associated with an adverse outcome in SARS patients (39). Thus, the ability of permissive cells to produce many inflammatory cytokines but not type I IFNs in response to SARS-CoV infection (18, 36) makes it necessary to investigate what role(s) these cytokines might have in host defense and/or SARS pathogenesis. Because most lung epithelial cells are polarized in vivo to exert their physiological functions (11), we determined the polarity of cytokine secretion by infected Calu-3 cells grown on the membrane inserts. We found that while IL-6 is secreted predominantly through the apical surface, the release of IL-8, IP-10, and other cytokines appears to be bidirectional, i.e., from both the apical and basolateral sides (Table (Table1).1). However, it remains to be seen as to whether such a relatively polarized secretion of IL-6 might be pathologically relevant to SARS.
Pulmonary M and DC are two of the most implicated innate immune cells critically involved in mediating early host defenses that control respiratory viral infection until an adaptive response can be generated (3, 13). The apical surface of highly differentiated lung epithelial cells is known to be exposed to “inhaled” pathogens and pathogens spread from foci of infection in adjacent areas of the lungs, whereas the basal surface is exposed to viremic pathogens through the basement membrane. In addition, the apical and basolateral surfaces of polarized epithelial cells lining the respiratory tracts are accessed by alveolar M
and DC, respectively, making them attractive targets for activation by SARS-CoV-induced lung epithelial cytokines. We used in vitro-derived and cytokine-driven primary human M
and DC from peripheral blood donors for our studies. We found that SARS-CoV-induced Calu-3 cell cytokines are potent in stimulating M
and DC, leading to the profound production of various inflammatory cytokines (Fig. (Fig.22 and and3).3). These results are significant because they provide the likely cellular basis for relaying and amplifying the acute inflammatory responses initiated by SARS-CoV-infected lung epithelial cells. We and others have previously shown that exposure to live, but not gamma-inactivated, SARS-CoV could modulate functions of M
and DC (e.g., the expression of costimulatory molecules, cytokine production, phagocytosis, and T-cell-priming activity) in a dose-dependent manner, in which an MOI of 1.0 was the minimal effective dose (18, 45). We estimated that the MOIs for DC and M
(106 cells) cultured with 50% of inactivated basolateral and apical supernatants containing 102 and 106/ml of inactivated SARS-CoV, respectively, were 0.0001 and 0.5, respectively, which were far less than 1.0. Thus, we believe that virally induced Calu-3 cell cytokines, but not the inactivated SARS-CoV or its encoded proteins, are likely responsible for the altered functions of these two classic innate immune cells.
The exact impact of the inflammatory mediators produced by DC and M stimulated with SARS-CoV-induced Calu-3 cell cytokines on the host defense against and/or the pathogenesis of SARS-CoV in vivo is currently unknown. However, IL-6 plays an important role in regulating not only the host defense by stimulating B-cell differentiation and Ab production but also the optimal development of megakaryocytes and platelets by synergizing with IL-3 (29, 47). In addition, it can induce the expression of acute-phase protein during hepatic injury as well (23). IL-8 is the major chemoattractant for neutrophils, whereas MCP-1 is particularly potent in recruiting activated M
, T cells, basophils, NK cells, and immature DC to the sites of inflammatory infection (32). Importantly, the elevated expression of many of the aforementioned inflammatory mediators, especially IL-6, IL-8, and MCP-1, has also been demonstrated in SARS patients, in which the rapid elevation of IL-8 expression was thought to positively correlate with the progression of pulmonary infiltrates and damage during the acute phase of SARS-CoV infection (27, 28). These observations make our findings clinically and pathologically relevant. While M
and pulmonary epithelial cells of SARS patients were capable of secreting MCP-1 and other inflammatory cytokines (27), DC were shown to be more potent in inducing the expression of MCP-1, IP-10, RANTES, and MIP-1α than that of IL-6 and TNF-α (18). Because the pathogenesis of many respiratory viral infections, including human metapneumovirus and respiratory syncytial virus infections, is believed to stem largely from the pronounced host inflammatory responses (29), it is thus likely that the acute epithelial cytokine-mediated enhancement of cytokine responses via interaction with M
and DC described in this study may play an important role in SARS pathogenesis.
We also revealed that SARS-CoV-induced Calu-3 cell cytokines had a negative impact on other characteristic functions of M and DC, including phagocytosis, as well as antigen processing and presentation to specific T cells, all of which are crucial in the linkage between the innate and the adaptive immune responses against infections. Specifically, we found that the cytokines released into the basolateral chamber of Calu-3 cell cultures could upregulate DC expression of CD40 and CD86 (Fig. (Fig.4)4) but not that of HLA-DR and CD83, a specific marker for the mature DC (data not shown). Interestingly, despite the enhanced expression of CD40 and CD86, these DC were defective in their ability to prime CD4+ T cells (Fig. (Fig.5).5). This is in striking contrast to the case for those DC exposed to live, but not gamma-inactivated, SARS-CoV, in which they became both phenotypically and functionally mature, as revealed by their greatly increased ability to enhance the expression of all of the aforementioned costimulatory molecules and to stimulate the proliferative response of naïve CD4+ T cells (45). We also identified that IL-6 and IL-8, but not IP-10, all of which are prominent cytokines released by SARS-CoV-infected Calu-3 cells, are likely responsible in large part for the significantly reduced T-cell-priming capacity of DC (Fig. (Fig.7).7). However, the contents of infected Calu-3 supernatants are extremely complex, making the involvement of other soluble factors alone or in combination likely. Regardless, our findings indicating a probable role of IL-6 and IL-8 in downregulating the ability of DC to activate T cells provide us the molecular and cellular bases to test the relevance to SARS pathogenesis both in vitro and in animal models, which are currently under investigation.
The transient decrease in the circulating CD4 and CD8 T-cell subsets has been reported to positively correlate with the adverse outcome of SARS-CoV infection (27). Although the exact mechanism(s) underlying this hematological manifestation in SARS patients remains undefined, the sequestration of specific T cells in the lungs or other infected peripheral tissues and apoptosis of uninfected T cells could lead to the transient decrease in circulating T cells. We are currently investigating whether DC cultured with epithelial cytokines derived from infected Calu-3 cells could actively arrest T cells at the G0/G1 phase and/or cause apoptosis of T cells. In addition, DC are the only antigen-presenting cells capable of priming naïve specific T cells within their draining lymph nodes, thereby inducing their expansion and the development of adaptive responses. In this context, the ability of SARS-CoV-induced epithelial cytokines to suppress this crucial activity of DC could be of significance in preventing the expansion of specific T cells that could delay or even abolish the development of the adaptive immune response. The ability of M to take up and digest invading microbes or damaged tissues within inflamed tissues is crucial, not only in host defense against infection, but also in facilitating the repair system of injured tissues to maintain homeostasis of the organism. Thus, the ability of SARS-CoV-induced Calu-3 cell cytokines to inhibit the phagocytic activity of M
could lead to an increased risk of secondary infection, as well as a delay in repairing damaged pulmonary tissues in SARS patients.
Airway epithelial cells are polarized in vivo, with their apical surface constantly exposed to alveolar M. It has been well documented that the apical surface of airway epithelial cells is the most prominent site for entry and release of progeny SARS-CoV (12, 35). In contrast, the basolateral surface of epithelial cells lining the airway has little expression of hACE2, making it an ineffective route for viral entry. This concept that SARS-CoV entry and release are mediated almost exclusively through the apical domain of permissive cells in a tissue culture system has recently been confirmed by using transgenic mice expressing hACE2 (41). Specifically, pulmonary infection and the onset of lung disease could be observed in only mice intranasally challenged, and not in those intraperitoneally challenged, with SARS-CoV. Such characteristic features of SARS-CoV infection within the spatially compartmentalized respiratory tract make our study relevant in terms of dissecting the complex cellular interplays during the acute phase of SARS-CoV infection.
Based on our results, we conclude that M and DC are two crucial innate immune cell types capable of relaying and amplifying the early acute inflammatory response initiated by SARS-CoV-infected lung epithelial cells. Importantly, virally induced epithelial cytokines IL-6 and, particularly, IL-8 could also compromise the ability of M
and DC in the clearance of invading pathogens and the promotion of effective adaptive immune responses, respectively, thereby further contributing to the pathogenesis of SARS. However, airway epithelial IL-6 and IL-8 responses induced by stimuli as diverse as other viruses, bacteria, house mite dust, and chemical and biological allergens (14, 16, 25, 31, 38, 48) rarely cause a life-threatening respiratory disease. This further suggests a complex mechanism of SARS pathogenesis that is far beyond the expression of these two epithelial cytokines. Although the pathogenesis of SARS-CoV infection remains elusive, both viral and host factors likely play key roles in promoting clinical and pathological features characteristic of SARS (8, 22). Specifically, whether SARS-CoV infection could cause cytopathic effects was shown to depend on the cell types and/or the accessibility of ACE2 or other viral receptors, whereas many SARS-CoV-encoded structural and nonstructural proteins appeared to antagonize the host innate immune responses through various strategies. We believe that our in vitro model of SARS-CoV infection using Calu-3 cells provides unique cellular and molecular insights into the intense inflammatory responses associated with SARS. However, further studies using primary airway epithelial cells and suitable animal models are urgently needed to verify our findings and to expand our knowledge on the pathogenesis of SARS.
Acknowledgments
We thank Mardelle Susman for help in preparing the manuscript. We also thank Zihong Chen for technical support in Western blotting.
This work was supported by National Institutes of Health grant R21AI072201, a Career Development Grant award through the Western Regional Center of Excellence for Biodefense and Emerging Infectious Diseases (U54 AI057156), and subcontract awards on SARS from the Viral Respiratory Pathogens Research Unit (NO1 AI3009) (to C.K.T.) and by U.S. Based Collaboration in Emerging Viral and Prior Diseases (NO1 AI25489) (to C.J.P.). T.Y. was supported by the James W. McLaughlin Fellowship Fund.
REFERENCES
Articles from Journal of Virology are provided here courtesy of American Society for Microbiology (ASM)
Full text links
Read article at publisher's site: https://doi.org/10.1128/jvi.01792-08
Read article for free, from open access legal sources, via Unpaywall:
https://europepmc.org/articles/pmc2655569?pdf=render
Free after 4 months at jvi.asm.org
http://jvi.asm.org/cgi/content/full/83/7/3039
Free after 4 months at jvi.asm.org
http://jvi.asm.org/cgi/reprint/83/7/3039
Free to read at jvi.asm.org
http://jvi.asm.org/cgi/content/abstract/83/7/3039
Citations & impact
Impact metrics
Article citations
Regulatory role of microRNAs in virus-mediated inflammation.
J Inflamm (Lond), 21(1):43, 04 Nov 2024
Cited by: 0 articles | PMID: 39497125 | PMCID: PMC11536602
Review Free full text in Europe PMC
Characterization of Unique Pathological Features of COVID-Associated Coagulopathy: Studies with AC70 hACE2 Transgenic Mice Highly Permissive to SARS-CoV-2 Infection.
PLoS Pathog, 20(6):e1011777, 24 Jun 2024
Cited by: 2 articles | PMID: 38913740 | PMCID: PMC11226087
COVID-19-Induced Rheumatoid Arthritis: Case Series and Systematic Review of the Literature.
Mediterr J Rheumatol, 35(2):291-297, 21 May 2024
Cited by: 0 articles | PMID: 39211019 | PMCID: PMC11350421
Treatment of multisystem inflammatory syndrome in children.
World J Pediatr, 20(4):325-339, 21 Mar 2024
Cited by: 1 article | PMID: 38509432
Review
The Therapy of SARS-CoV-2 Infection in Children.
J Clin Med, 13(1):120, 25 Dec 2023
Cited by: 0 articles | PMID: 38202127 | PMCID: PMC10779459
Review Free full text in Europe PMC
Go to all (168) article citations
Similar Articles
To arrive at the top five similar articles we use a word-weighted algorithm to compare words from the Title and Abstract of each citation.
Severe acute respiratory syndrome and the innate immune responses: modulation of effector cell function without productive infection.
J Immunol, 174(12):7977-7985, 01 Jun 2005
Cited by: 104 articles | PMID: 15944304
SARS-CoV-2 Isolates Show Impaired Replication in Human Immune Cells but Differential Ability to Replicate and Induce Innate Immunity in Lung Epithelial Cells.
Microbiol Spectr, 9(1):e0077421, 11 Aug 2021
Cited by: 15 articles | PMID: 34378952 | PMCID: PMC8552721
SARS-CoV replication and pathogenesis in an in vitro model of the human conducting airway epithelium.
Virus Res, 133(1):33-44, 23 Apr 2007
Cited by: 75 articles | PMID: 17451829 | PMCID: PMC2384224
Review Free full text in Europe PMC
Modeling the early events of severe acute respiratory syndrome coronavirus infection in vitro.
J Virol, 80(6):2684-2693, 01 Mar 2006
Cited by: 98 articles | PMID: 16501078 | PMCID: PMC1395447
Funding
Funders who supported this work.
NIAID NIH HHS (6)
Grant ID: N01 AI25489
Grant ID: U54 AI057156
Grant ID: N01 AI3009
Grant ID: R21 AI072201
Grant ID: R21AI072201
Grant ID: N01AI25489