Abstract
Free full text

Mammalian glycosylation in immunity
Abstract
Glycosylation produces a diverse and abundant repertoire of glycans, which are collectively known as the glycome. Glycans are one of the four fundamental macromolecular components of all cells, and are highly regulated in the immune system. Their diversity reflects their multiple biological functions that encompass ligands for proteinaceous of receptors known as lectins. Since the discovery that selectins and their glycan ligands are important for the regulation of leukocyte trafficking, it has been shown that additional features of the vertebrate immune system are also controlled by endogenous cellular glycosylation. This Review focuses on the emerging immunological roles of the mammalian glycome.
Glycosylation is the enzymatic process that produces glycosidic linkages of saccharides to other saccharides, proteins and lipids, and is probably as ancient as life itself. Unicellular and multicellular organisms depend on glycosylation to produce monomeric and multimeric glycan linkages that are essential for cell viability and normal function1–4. The resulting glycome encompasses a diverse and abundant repertoire of glycans, which are one of the four fundamental macromolecular components of all cells (together with nucleic acids, proteins and lipids) (FIG. 1). Glycans have important biological functions in protein maturation and turnover, cell adhesion and trafficking, and receptor binding and activation5–8.
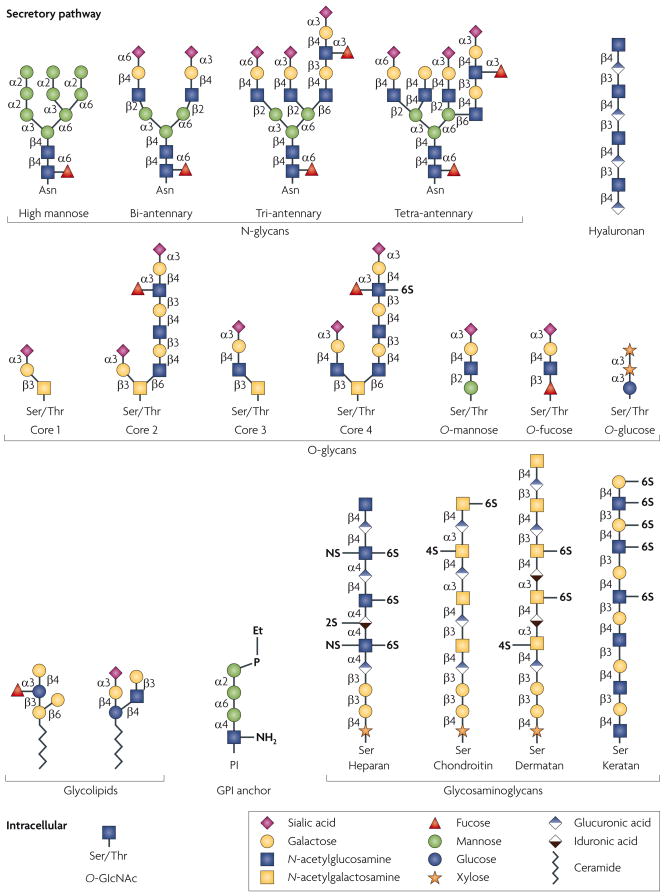
Glycan structures of the six classes of secretory glycan (N-glycans, hyaluronan, O-glycans, glycolipids, glycosylphosphatidylinositol (GPI) anchor and glycosaminoglycans) and the single intracellular glycan O-linked β-N-acetylglucosamine (O-GlcNAc) are shown. Representative examples of each type are indicated using the symbol nomenclature for monosaccharides (see key). Multiple and multi-antennary examples of the predominant mature, complex-type N-glycans are shown, including the high-mannose N-glycan that is found attached to some glycoproteins. Examples of core 1–4 O-glycans are depicted, as well as O-mannose, O-fucose and O-glucose structures. Core 5–7 O-glycans are not shown. The GPI anchor and examples of the glycosaminoglycans and glycolipids are also depicted. In all examples, glycan linkages are identified by the anomeric configuration (α orβ) of the donor saccharide following its linkage to the ring position (1–6) of the glycan acceptor. Et–P denotes a phosphoethanolamine linkage. NS, 2S, 4S and 6S denote the sulphation positions of the glycosaminoglycan chains. Asn, asparagine; Ser, serine; Thr, threonine.
Glycosylation is prominent in the lumen of the endoplasmic reticulum (ER) and in the Golgi apparatus. The cellular repertoire of glycans that are produced by glycosylation in these organelles of the secretory pathway reflects the combinatorial expression of subsets of glycosyltransferase and glycosidase enzymes, of which there are more than 200 in the mammalian genome. The formation and breakdown of glycans are regulated at several levels in the cell. One of the mechanisms involves transcriptional regulation of the genes that encode these enzymes, but others include access to substrates and molecular interactions that alter enzyme localization in the lumen of the ER and Golgi2,9,10. Changes in the glycome can occur in response to environmental and genetic stimuli, and are frequently associated with the acquisition of altered cellular phenotypes1–10.
Glycosylation also occurs among proteins in the cytoplasm and nucleus through the actions of the Ogt glycosyltransferase, which produces a reversible O-linked β-N-acetylglucosamine (O-GlcNAc) post-translational modification11. O-GlcNAc linkages are important in numerous physiological processes and disease. In contrast to this intracellular glycosidic bond that is formed by Ogt, secretory glycosylation in the ER and Golgi produces a large structural repertoire, including oligomeric glycan linkages that are presented at the cell surface and in extracellular compartments. Intracellular glycosylation has been reviewed elsewhere and is not discussed further in this Review12.
Glycosylation can substantially modify the structure and function of proteins by steric influences involving intermolecular and intramolecular interactions and can mediate the production of glycan ligands for lectins (FIG. 2). Lectins are proteins with glycan-binding activity that were first described in plants but subsequently have been found in all cells, from microorganisms to humans13–15. Lectin–glycan binding is a means of molecular recognition, which organisms can use to identify and decode the biological information that is present in their own cellular glycome as well as the glycomes of other organisms.
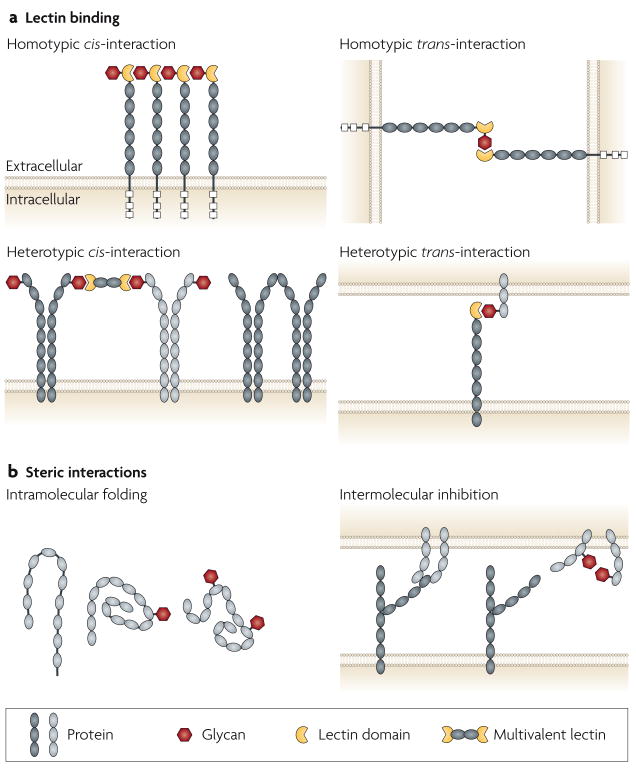
Glycans can function as ligands for lectins (a) and as steric elements that alter molecular interactions at the cell surface and between extracellular compartments (b). Interactions can involve the same cell (cis) or different cells (trans), and can be either homotypic or heterotypic, and intramolecular or intermolecular. It is often difficult to distinguish steric effects of glycans from alterations in lectin–glycan binding. This figure is idealized and not drawn to scale.
The extracellular positioning of the secretory glycome and its phylogenetic variation between organisms (BOX 1) allows glycans to be involved in pathogen–host interactions16. Glycan linkages of the mammalian host are an abundant and attractive target for pathogens to establish an infection through lectin binding. Mammals have developed a phyloglycomic recognition system, which includes a subset of Toll-like receptors (TLRs) and C-type lectins, to detect the glycans of lower organisms as a means of non-self discrimination and immunological activation17. These pattern-recognition receptors and their responses to non-vertebrate glycans are not considered further in this Review.
The development and function of the mammalian immune system are associated with restructuring of the cellular glycome. The immune-cell glycome is altered during cell differentiation, activation and apoptosis, and these alterations are being linked to both homeostatic and disease mechanisms of immune-cell functions, including immune-cell trafficking and differentiation, antigen- and cytokine-receptor activation, auto-immunity, and the induction of leukocyte apoptosis18–21. This Review discusses the roles of mammalian secretory glycosylation in the immune system as established by in vivo studies that combine knowledge of the glycan biosynthetic pathways with genetic and cellular research approaches.
Cell trafficking
Of the immunological functions that have been ascribed to mammalian glycosylation, perhaps the best understood involves the glycan ligands of the lectins E-selectin, L-selectin and P-selectin22,23. The selectins and their ligands form a cell–cell adhesion system that operates mainly between leukocytes and endothelial cells. This system is essential for the tethering and rolling of circulating leukocytes on the vascular cell wall, which promotes subsequent firm binding by the integrins and the transmigration of leukocytes through the endothelium and into the surrounding tissue24–26. This mechanism of cell trafficking is responsible for the colonization of lymphoid organs by lymphocytes during development and allows leukocytes to migrate into lymphoid organs or inflamed tissues during an immune response. Each selectin binds a particular glycan ligand, such as sialyl-Lewis-X (sLex) for E- and P-selectin, and 6-sulpho-sLex for L-selectin. The binding interactions between selectins and their ligands can occur between many different cell types, including between lymphoid and non-lymphoid, as well as non-immune, cells26,27.
Regulation of selectins and their ligands
Various cellular mechanisms regulate selectin function. Selectin expression, for example, is normally restricted to endothelial cells, platelets and leukocytes: E- and P-selectin expression is induced on the vascular endothelium during inflammation, P-selectin expression is also induced on platelets and L-selectin is expressed on the surface of leukocytes28. In addition, expression of the glycoproteins that ‘carry’ (that is, are scaffolds for the construction of ) the selectin ligands sLex and 6-sulpho-sLex can be similarly regulated, thereby altering the abundance and cellular localization of sLex and 6-sulpho-sLex. The glycoproteins that carry selectin ligands continue to be discovered and include P-selectin glycoprotein ligand 1 (PSGL1; also known as SELPG), E-selectin ligand 1 (ESL1), CD34, CD44, glycosylation-dependent cell-adhesion molecule 1 (GlyCAM1) and αMβ2-integrin 23,29,30. Another mechanism that controls selectin function involves the combinatorial enzymatic activities of different Golgi-resident glycosyltransferases and sulphotransferases that contribute to the synthesis of sLex and 6-sulpho-sLex. These enzymes are tightly regulated in different cell types and can therefore contribute to the diverse functions of selectins in the immune system.
The synthesis of selectin ligands requires a set of enzymes, including fucosyltransferases, sialyltransferases, galactosyltransferases, glucosaminyltransferases and sulphotransferases, that act in a specific order on an underlying glycan chain as it transits through the Golgi apparatus. The expression of these enzymes can be regulated in response to intracellular signalling and transcription-factor induction31–33. The sLex and 6-sulpho-sLex glycans exist in vivo mainly on the O-glycan chains of glycoproteins, and their production depends on the expression of the fucosyltransferases Fut4 and Fut7. The absence of Fut7 or Fut4, and in particular of both enzymes together, markedly attenuates lymphocyte homing to peripheral lymph nodes and myeloid-cell trafficking in inflammation34–36.
The α2,3-sialyltransferases ST3Gal4 and ST3Gal6 seem to participate in the formation of selectin ligands in vivo. Studies of mice that lack ST3Gal4 have revealed a partial deficiency in selectin ligands, with a marked effect on leukocyte homing and trafficking in inflammation, which was not observed in mice that lack ST3Gal1, ST3Gal2 or ST3Gal3 (REFS 37–39). The in vivo effect of ST3Gal6 deficiency has yet to be reported.
The contribution of O-glycans in carrying selectin ligands in vivo has been investigated in mice that lack core 2 N-acetylglucosaminyltransferase 1 (GCNT1) activity. GCNT1 is one of three glycosyltransferases that construct the core 2 O-glycan branch, which is a type of O-glycan structure that is commonly found on O-glycoproteins. GCNT1 deficiency strongly attenuated E- and P-selectin-mediated inflammation involving myeloid cells, but had relatively little effect on the lymphoid system, including the formation of secondary lymphoid organs and adaptive immune responses40. Subsequent studies of GCNT1-deficient mice detected a subtle but significant defect in the formation of L-selectin ligands in cells that form the high endothelial venules of some lymph nodes, which was associated with decreased kinetics of peripheral lymphocyte homing41,42. The finding that GCNT1 deficiency did not severely affect L-selectin ligands and lymphoid trafficking was addressed by the discovery of an endogenous β1,3-glucosaminyltransferase. In endothelial cells, this enzyme compensates for the lack of core 2 O-glycan branches and extends the core 1 O-glycan branch to provide a scaffold on which L-selectin ligands can also be produced43. Moreover, with loss of both this core-1-extension enzyme and GCNT1, L-selectin ligands were observed on N-glycan chains, which seem to contribute to a robust lymphocyte homing response in the absence of these two glycosyltransferases44. Compensation was also observed between the glycan sulphotransferases that are required for 6-sulpho-sLex synthesis, namely GlcNAc6ST1 and GlcNAc6ST2. In contrast to deficiency of a single enzyme, deficiency of both sulphotransferases led to almost complete loss of L-selectin-ligand formation on the high endothe-lial venules of lymph nodes, together with a marked decrease in lymphocyte homing and immune function45,46. The different defects of innate and adaptive immune responses that are associated with these profiles of selectin-ligand deficiency reflect the combinatorial and regulated expression of glycosyltransferases and glycan sulphotransferases that construct the E-, L- and P-selectin ligands. This modulation of ligand-expression profiles has progressed our understanding of the complexity of selectin function in the mammalian immune response.
Selectin-targeted therapy
Selectin-mediated binding promotes cell–cell adhesion that induces intracellular signalling and could therefore be exploited in therapeutic approaches. Once engaged by their cognate glycan ligands, the selectins recruit tyrosine kinases, such as FYN and spleen tyrosine kinase (SYK), to their cytoplasmic domains, thereby inducing intracellular tyrosine phosphorylation, activating mitogen-activated protein kinases and promoting integrin activation47–50. Pharmacological intervention that is focused on inhibiting the interactions between leukocytes and endothelial cells might therefore block the intracellular signalling pathways that are induced by selectin binding. In some disease contexts, selectin-mediated cellular trafficking might contribute to tumour-cell adhesion in metastasis and leukocyte infiltration in autoimmune disease. Indeed, blocking selectin binding or inhibiting the synthesis of selectin ligands can attenuate the pathogenesis of inflammatory diseases and decrease the tumorigenicity of cancer cells51–55. In other contexts, the induction of selectin ligands can be beneficial. Glycan remodelling of the mesenchymal stem-cell surface in vitro to express selectin ligands increased cell tropism for the bone marrow after transplantation and resulted in increased bone formation56. Pharmacological alteration of the production of selectin ligands and modulation of the binding of selectins in vivo are active areas of research and development that could ultimately lead to new treatments for the diseases of the immune system that are linked with leukocyte trafficking and activation.
Adaptive immunity and autoimmune disease
During their maturation, the antigen receptors of B and T cells are post-translationally modified with N- and O-glycan chains. The addition of different N-glycan linkages to the B-cell receptor (BCR) and T-cell receptor (TCR) by various glycosyltransferases in the Golgi apparatus seems to alter the molecular interactions at the cell surface that regulate receptor trafficking, association with other glycoproteins on the cell surface, signal transduction and receptor internalization by endocytosis. Moreover, glycans and endogenous multivalent lectins that bind to them might, in some cases, be engaged in a precise spatial and geometrically predictable array on the cell membrane (often termed a lattice) that could control glycoprotein function (see later).
TCR signalling
Glycosylation is involved in the cellular mechanisms that control the threshold of TCR activation, and can therefore have profound effects on the function of the adaptive immune system. The GnT5 glycosyltransferase is encoded by Mgat5 (mannoside acetylglucosaminyl transferase 5) and initiates formation of the β1,6 N-glycan-branch structure on various glycoproteins, including the TCR. This N-glycan branch commonly includes polylactosamine, which consists of N-acetyllactosamine disaccharide repeats and is a ligand for various lectins of the galectin family57. In studies of mice that lack GnT5 function, TCR clustering is significantly increased, which coincides with a decreased threshold of T-cell activation (FIG. 3a). GnT5-deficient mice have increased delayed-type hypersensitivity responses, increased susceptibility to experimental autoimmune encephalomyelitis (EAE) and increased glomerulonephritis that is associated with immune-complex deposition58. The phenotype that is conferred by GnT5 deficiency also includes a TCR trafficking abnormality, and the resulting hyperimmune response might be a contributing factor in some human autoimmune diseases59.
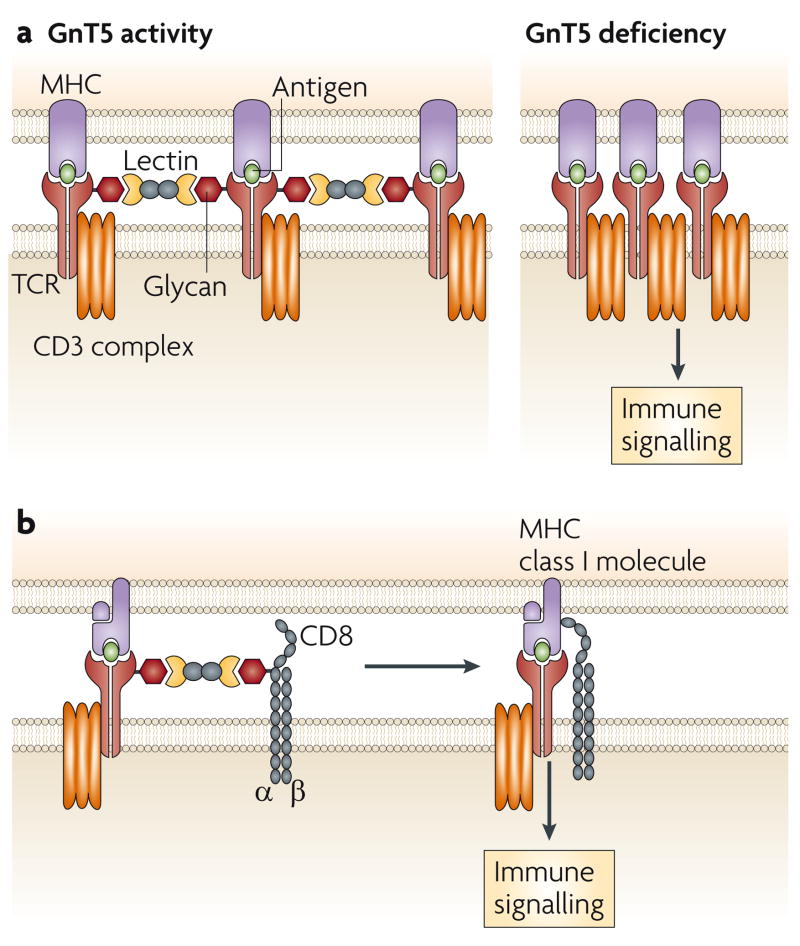
a. Co-clustering of αβ T-cell receptors (TCRs) decreases in the presence of N-glycan branching on the TCR through lectin binding involving one or more of the galectins. Loss of GnT5 glycosyltransferase activity, which mediates N-glycan branching, and therefore lectin binding, increases the propensity for TCR and co-receptor co-clustering and decreases the threshold of TCR activation. b. Lectin binding might also control TCR signalling in cytotoxic T cells by inhibiting the association of the TCR with the CD8 co-receptor.
Endogenous lectins have been implicated in the formation of homotypic and heterotypic TCR interactions that modulate immunological signalling. Galectin-3 is chemically crosslinked with the TCR on naive resting T cells in a GnT5-dependent manner, and disruption of this interaction with glycan-ligand mimetics of galectin-3 also results in increased TCR clustering and a decreased threshold of activation58. By contrast, in post-activated mature cytotoxic T cells, a decrease in effector activity, such as occurs during anergy, can be reversed by ex vivo treatment with glycan-ligand mimetics of galectin-3. Decreased effector activity is associated with decreased co-localization of the TCR and CD8, and decreased binding of the T cells to HLA–peptide tetramers60 (FIG. 3b). Studies of galectin deficiency in mice also indicate that galectin-1 modulates the threshold of TCR signalling during thymocyte development61. Interestingly, treatment of cells or mice with high concentrations of GlcNAc increases GnT5-mediated N-glycan branching and inhibits TCR activation and autoimmune responses in mouse models of EAE and type 1 diabetes62. This is thought to be the result of a higher concentration of the cellular donor UDP (uridine 5′-diphosphate)–GlcNAc in the Golgi apparatus, which might increase the capacity of GnT5 and other closely related glycosyltransferases to produce GlcNAc linkages that contribute to N-glycan-branch formation.
BCR signalling
The BCR forms complexes with immunoregulatory glycoproteins that modulate the threshold of BCR activation, including the lectin CD22 (also known as Siglec-2), a member of the Siglec (sialic-acid-binding immunoglobulin-like lectin) family63 that is expressed exclusively on B cells. The structure of CD22 includes an extracellular lectin domain at the amino terminus of several immunoglobulin-like domains, and an intracellular immunoreceptor tyrosine-based inhibitory motif repeat, which has an inhibitory role as it recruits the phosphatase SHP1 (SRC-homology-2-domain-containing protein tyrosine phosphatase 1) and thereby dampens BCR signalling64. Synthesis of the glycan ligands for CD22 requires the ST6Gal1 sialyltransferase, which adds sialic acid in an α2,6 linkage to the underlying galactose residue of the N-acetyllactosamine disaccharide65. Although this glycan ligand for CD22 is present on many glycoproteins, in addition to CD22 itself, CD22 tends to bind to the glycan ligands of neighbouring CD22 molecules, thereby forming homotypic multimers 66. In the absence of ligands for CD22 owing to ST6Gal1 deficiency, BCR activation and humoral immunity are markedly decreased, and this coincides with increased co-localization of the BCR with CD22, constitutive recruitment of SHP1 to CD22, decreased protein phosphotyrosine signalling, increased BCR trafficking to clathrin-enriched microdomains and increased BCR endocytosis65,67,68. The absence of both CD22 and ST6Gal1 restored normal BCR signalling, microdomain association and rate of endocytosis. Measurements of intracellular signal transduction in response to BCR stimulation in the absence of the ST6Gal1-dependent ligands of CD22 show dominance of negative signalling that suppresses B-cell activation. This negative signalling complex prevents the development of autoimmune disease and markedly improves survival in LYN-tyrosine-kinase-deficient mice68. These findings are consistent with a model of BCR function in which Siglec-dependent binding of CD22 to the BCR dictates the threshold of immunological activation (FIG. 4).
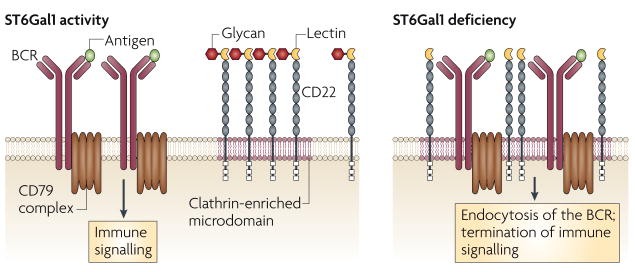
The threshold of B-cell receptor (BCR) activation is modulated by ST6Gal1 and CD22, a member of the Siglec (sialic-acid-binding immunoglobulin-like lectin) family that has a negative role in BCR signalling. CD22 Siglec binding to its glycan ligand promotes homotypic CD22–CD22 interactions, thereby decreasing the frequency of interaction of CD22 with the IgM BCR on mature naive B cells. Loss of CD22 Siglec ligands owing to ST6Gal1 sialyltransferase deficiency markedly increases the co-localization of CD22 with the BCR, thereby increasing the threshold of immune signalling and promoting endocytosis of the BCR in a CD22-dependent manner.
CD22 can bind ligands that are presented in trans and this might modulate glycoprotein complex assembly and its localization at the B-cell surface177. Moreover, the induced co-localization of CD22 with the BCR in cis seems to occur in a Siglec-independent manner that might involve protein–protein interactions that are mediated by the other extracellular (immunoglobulin-like) domains of CD22. Such a mechanism might explain how binding of dendritic cells to CD22 in a Siglec-independent manner inhibits B-cell proliferation69. The biology of CD22 is complex and there is potential for many Siglec-independent functions, some of which could be influenced by the Siglec-binding activity of CD22.
The glycan-dependent immune modulation that has been defined for ST6Gal1 in B cells and GnT5 in T cells indicates that crucial glycoprotein-selective functions can be modulated by single glycosyltransferase genes that contribute to the cellular mechanisms of antigen-receptor activation and the pathogenesis of autoimmune disease.
Antibody function
The secreted immunoglobulins that are produced following B-cell activation and immunoglobulin class switching are glycoproteins with characteristic glycan linkages70. The glycans of IgG are important for binding to Fc receptors and for other effector functions, including binding to complement component 1q (C1q) and complement activation71. Alterations of endogenous IgG glycosylation have been reported in various immune diseases, including rheumatoid arthritis and IgA nephropathy. In patients with rheumatoid arthritis, the N-glycans that are attached to IgG often lack terminal galactose and sialic-acid linkages and therefore bear exposed N-acetylglucosamine72. This terminal linkage is common to non-mammalian vertebrate species as well as various invertebrates and lower organisms, and is a ligand for some endogenous mammalian immune receptors and lectins that might promote inflammatory responses73,74. In patients with IgA nephropathy, the O-glycans that are attached to IgA are similarly truncated to expose N-acetylglucosamine, which correlates with antibody deposition in the inflamed kidney75. Deficiency of the β1,4GalT1 glycosyltransferase (one of several galactosyltransferases) recapitulates the pathology of IgA nephropathy in mice76. By contrast, the extent of IgG sialylation correlates with decreased inflammation following Fc receptor binding77. This seems to be due to the α2,6 sialic acids on IgG that are contributed by ST6Gal1 and are linked to the anti-inflammatory effects of intravenous IgG when used as a therapy for autoimmune diseases78.
Cytokine receptors
The core 1,6 fucose linkage is found on most N-glycans owing to the activity of Fut8 fucosyltransferase, and deficiency of this enzyme results in an emphysema-like disease in mice. Remarkably, this syndrome was linked to impaired binding of the receptor for transforming growth factor β1 (TGFβ1) to its ligand, and could be attenuated by increasing the concentration of TGFβ1 (REFS 79,80). An inherited or sporadic gene mutation that introduces new glycosylation sites into a protein can also produce receptors with ligand-binding defects81. In this study, an N-glycosylation site that was found at a new position in the protein sequence of the interferon-γ receptor 2 (IFNγR2) resulted in the formation of an N-glycan at this location that disrupted the ability of the receptor to bind its ligand. Such pathological changes to protein glycosylation represent another mechanism by which glycosylation can contribute to protein dysfunction and disease aetiology. It seems that glycosylation in the Golgi apparatus can in some cases directly affect the binding of immunomodulatory receptors to cognate ligand.
Innate immunity and autoimmune disease
Glycans might have been used to sense the presence of non-self since the beginning of multicellular life. Glycomic differences in phylogeny and between species can allow for this type of molecular discrimination between self and non-self, and thus glycan structures are activators of the innate immune system and pathogenic triggers for autoimmune diseases. Unlike new-world primates and other vertebrates, old-word primates lack the α1,3 galactosidase (α1,3Gal) galactose-terminated glycan branch linkage owing to a germline mutation of the α1,3 galactosyltransferase. This results in a substantial fraction of circulating immunoglobulins in old-world primates (including humans) that bind the α1,3Gal epitope and contribute to the hyperacute rejection of xenotransplants from other mammals82. Another emerging example concerns endogenous antibodies that are specific for the sialic-acid variant N-glycolylneuraminic acid (NeuGc). Humans seem to be unique in their lack of the enzyme that converts N-acetylneuraminic acid (NeuAc) to NeuGc, and the consumption of NeuGc in the diet could lead to its incorporation into endogenous glycoproteins, resulting in the formation of NeuGc-specific antibodies83. Antibodies specific for glycans are also induced during human Tn syndrome, which is a haematological stem-cell disorder that is associated with thrombocytopaenia and leukocytopaenia and is caused by a mutation of COSMC (also known as C1GALT1C1). This gene normally encodes a protein with chaperone function that is required for β1,3 galactosyltransferase activity in O-glycan biosynthesis84. Mutation of COSMC leads to a truncated O-glycan structure on haematopoietic cells and immunoglobulins (known as the Tn antigen) that functions as an epitope for antibody production and cell lysis and might contribute to IgA nephropathy and Henoch–Schönlein purpura84.
The aberrant synthesis of endogenous glycans can result in the exposure of cryptic epitopes that are perceived by the immune system as non-self and induce an inflammatory condition in the absence of infection that is linked to the pathogenesis of autoimmune disease. This explains how α-mannosidase-II (αM-II; also known as MAN2A1) deficiency causes an autoimmune syndrome in mice that is remarkably similar to systemic lupus erythematosus. Disease pathogenesis in αM-II deficiency is non-haematopoietic in origin (unlike other autoimmune disease models), is independent of both the adaptive immune system and C3 complement activation, can be mitigated by intravenous IgG administration and is linked to chronic activation of the innate immune system85. The block in N-glycan maturation in the Golgi that is caused by αM-II deficiency increases the expression of phylogenetically primitive hybrid N-glycans at the cell surface and among extracellular glycoproteins. The exposed mannose linkages of these primitive hybrid N-glycans on multiple glycoproteins are ligands for endogenous mannose-binding lectin receptors of the innate immune system, and this interaction results in the chronic induction of a pro-inflammatory response that can recruit and activate macrophages. The requirement for complex N-glycan branching to safeguard against this immunological recognition of cryptic mannose ligands reveals a distinct mechanism in the pathogenesis of autoimmune disease that can be termed epitope modification or neo-ligand formation. Mechanisms that impair N-glycan branching could therefore contribute to the pathogenesis of various autoimmune syndromes of presently unknown origin.
The mechanisms that establish and maintain immunological tolerance also involve lectin–glycan ligand interactions that suppress immune-cell activation. For example, galectin-1 is becoming increasingly important in the study of T-cell and dendritic-cell biology and in the pathogenesis of immunological diseases86. Galectins are involved in attenuating graft-versus-host disease, collagen-induced arthritis, type 1 diabetes and T-cell-mediated tumour rejection18,86–91. Galectin-1 seems to bridge innate and adaptive immunity in part by regulating the Fc receptor- and MHC-dependent immune functions of monocytes and macrophages through an extracellular-signal-regulated kinase (ERK) signalling pathway91. The immunomodulatory activity of galectin-1 extends to its involvement in fetal–maternal tolerance during pregnancy. Deficiency of galectin-1 increases the loss of fetuses that have been conceived by the mating of allogeneic mice. In addition, treatment of galectin-1-deficient mice with exogenous galectin-1 restores normal fecundity, and this coincides with the induction of tolerogenic dendritic cells and the proliferation of interleukin-10 (IL-10)-producing regulatory T cells92.
Normal and abnormal catabolism of glycans can also provide signals of immunological importance. Hyaluronan is a glycan that consists of a disaccharide repeat that modulates innate immunity by interacting with TLRs. This glycan is synthesized as a linear chain unattached to either a protein or a lipid and its size seems to be important. In response to tissue injury, endogenous hyaluronidases cleave extracellular hyaluronan into small fragments that induce inflammatory gene expression by binding TLR2 and TLR4 (REFS 93,94). Some pathogens express hyaluronidase as a virulence factor that may cleave hyaluronan into fragments that do not bind TLRs and thereby subvert the immune response. The enzymatic regulation of hyaluronan synthesis and degradation is increasingly recognized as having an important role in innate immunity and the response to infection95.
Immune-cell differentiation
Glycoproteins, such as antigen receptors and MHC molecules, are important regulators of immune-cell differentiation and must be properly folded to function. Without correct folding and maturation in the ER and Golgi apparatus, the expression of improperly folded and dysfunctional glycoproteins on the cell surface and in extracellular compartments can cause defects in the signalling pathways that promote immune-cell differentiation. In addition, acquisition of secondary determinants of the differentiated immune-cell phenotype can also be controlled by glycan-dependent mechanisms. For example, glycosaminoglycan sulphation by the sulphotransferase NDST2 (N-deacetylase/N-sulphotransferase (heparan glucosaminyl) 2) promotes the development of mast-cell secretory granules, which are essential for the function of these immune cells96,97.
T-cell ontogeny and endogenous glycans
The range and structures of peptides that are presented by MHC molecules could be influenced by glycan linkages on native glycoproteins and thus could contribute to the immunological definition of self98. Although peptides that are produced in the cell for presentation on MHC class I molecules are typically deglycosylated during proteolysis in the proteasome, some glycan linkages persist on various peptides and are recognized by cytotoxic T cells99. Peptides that arise from external sources can retain a broader range of glycan linkages, which might influence peptide proteolysis and loading on MHC class II molecules for binding by αβ TCRs. For example, some TCRs from T cells that have been isolated from mice with collagen-induced arthritis recognize an MHC class II-associated peptide of type II collagen that contains galactose linked to hydroxylysine residues of collagen101. Fate decisions in thymic T-cell development can be modulated by galectin-1, which promotes negative selection through ERK signalling100. Altered glycosylation in glycosyltransferase-deficient animals, for example, may influence the formation of the TCR repertoire and thereby have an effect in immunity. The repertoire of T cells in an intact mammal, which is determined by self-antigen presentation, might therefore reflect, in part, the portfolio of endogenous glycopeptides and their presentation by MHC molecules. This possibility could also involve intracellular O-GlcNAc glycan linkages that are attached to many cytoplasmic and nuclear proteins102. Similarly, glycolipids might be involved in development of the natural killer T (NKT)-cell lineage; the presentation of an endogenous glycolipid structure by the CD1 molecule might promote the development of a functional NKT-cell population103.
T-cell repertoire development
Expression of the glycoproteins CD4 and CD8 as TCR co-receptors confers MHC class II and class I restriction of TCR activation, respectively, during T-cell development in the thymus. The sialylation state of glycans of the CD8–α β TCR heterodimer affects co-receptor binding to MHC class I molecules. A low level of O-glycoprotein sialylation in CD4+CD8+ thymocytes is associated with binding to MHC class I tetramers, whereas the increased abundance of sialic-acid linkages on differentiated CD8+ thymic T cells decreases the binding avidity of CD8 for MHC class I molecules, thereby decreasing T-cell adhesion to MHC class I tetramers104–106. This decrease in binding to MHC class I molecules is in part mediated by the sialic acids that are produced by the ST3Gal1 sialyltransferase, as deficiency of this enzyme in mice increases the binding of MHC tetramers to single-positive CD8+ thymic T cells and alters the repertoire of the TCR β-chain variable region (Vβ)105. This mechanism seems to involve a change in the CD8–αβ TCR heterodimer conformation that promotes MHC class I binding in the presence of unsialylated core 1 O-glycans, which lack the steric hindrance and negative charge that are associated with sialic acid105,107. However, another study that used a different MHC–peptide complex showed no significant change in tetramer binding of ST3Gal1-deficient CD8+ thymic T cells108. The reasons for this discrepancy are not clear, but they might relate to the intrinsic binding affinities of the TCR transgenes and MHC tetramer probes that were used in each study. Nevertheless, the expression and deficiency of ST3Gal1 alter the TCR Vβ repertoire in an opposing manner, which indicates that ST3Gal1 has a role in the MHC-restricted thymic differentiation of CD8+ T cells105,109.
Notch ligands and immune-cell development
The binding of Notch ligands to Notch receptors provides another example of how glycosyltransferases can influence intracellular signal transduction and thereby govern the development and function of immune cells. Notch signalling, which controls cell-fate determination in many organisms, dictates lineage commitment in the mammalian lymphoid system and has recently been shown to be involved in myelopoiesis110. The closely related Fringe glycosyltransferases (Manic, Radical and Lunatic in the mammalian genome) were discovered as genes that establish cell-boundary fields in embryogenesis and were later found to intersect with the functions of Notch receptors in early development111–115. The Fringe glycosyltransferases are β1,3 N-acetylglucosaminyltransferases that operate on the O-linked fucose glycans. These glycans are found in the extracellular epidermal growth factor (EGF)-like repeats of Notch receptors in the secretory pathway and control cell-surface Notch signalling116,117. The O-fucose linkage that is the substrate of the Fringe glycosyltransferases is first generated by Pofut1 (protein O-fucosyltransferase). The glycan products of the Fringe glycosyltransferases are then substrates of β1,3 galacto-syltransferases. These glycans provide a physiological mechanism to modulate Notch binding and activation by the extracellular glycoprotein ligands Jagged/Serrate and Delta118–123. In addition to generating the O-fucose linkage that is the substrate of the Fringe glycosyltransferases, Pofut1 provides a chaperone activity that is independent of its glycosyltransferase activity and promotes Notch maturation124. More recently, Notch signalling has been shown to be also influenced by the Rumi glycosyltransferase, which generates O-glucose linkages on the EGF-like repeats of Notch125,126.
Signalling through the Notch axis has profound effects on lymphocyte development and activation in mammals. For example, a constitutively active form of Notch can promote the development of CD8+ T cells in the absence of MHC class I molecules127. The effect of Fringe glycosyltransferases on lymphoid-cell fate includes the suppression of B-cell development from lymphoid progenitors in the thymus128. However, Fringe activity promotes increased Notch binding to Delta-like ligands, which can lead to a block in T-cell development. This might be due to an effect on the access of progenitor cells to intrathymic niches or a direct effect on the induction of T-cell differentiation signals129,130. Moreover, Notch activation leads to the formation of marginal-zone B cells in the spleen and promotes immunoglobulin secretion by a signalling mechanism that involves Notch binding to the Delta-like 1 ligand131–133. Dendritic- and myeloid-cell differentiation also seem to be modulated by Notch signalling in response to the binding of Notch ligands, and the activation of NK-cell cytotoxicity is promoted by the Notch ligand Jagged2 (REFS 134,135). Immune responses can also be inhibited by Notch binding to Fringe-dependent glycan ligands; for example, Delta-like-1 ligand seems to inhibit Notch activation, which skews the outcome in airway inflammation from a T helper 2 (TH2)- to TH1-cell response136. The functional relationships between Notch receptors and their glycan ligands are only partly understood, but already a remarkable complexity in glycan-mediated and Notch-dependent immune-cell differentiation is indicated.
TH-cell polarization
Activated CD4+ effector T cells can differentiate into distinct subsets (TH1, TH2, TH17 and regulatory T cells), each of which produces a unique profile of cytokines that differentially govern immune responses to various stimuli. It seems that mammalian glycosylation has a role in the emergence and phenotypic profiles of these TH-cell subsets, in part by its effect on the production of ligands of various lectins. The Delta-like 1 and Delta-like 4 glycan ligands of Notch promote TH1-cell differentiation of CD4+ T cells in part by suppressing IL-4-mediated signalling, which would otherwise induce TH2-cell differentiation and promote TH2-cell immune responses137,138. By contrast, the GnT5 glycosyltransferase promotes TH2-cell immune responses by inducing differentiation signals that inhibit IFNγ production139. The induced deficiency of galectin-1 restores the TH1–TH2-cell balance of T-cell infiltrates in classical Hodgkin’s lymphoma, apparently by modulating the secretion of TH2-cell cytokines and the proliferation of regulatory T cells140. Differential glycosylation of TH-cell subsets has been linked with their susceptibility to death by galectin-induced apoptosis, which provides an explanation for the increased TH1- and TH17-cell responses that have been observed in galectin-1-deficient mice141. A related mechanism might operate during parasitic infection by which helminth glycans skew CD4+ T-cell immune responses towards the TH2-cell phenotype142.
Apoptosis
Cell death by apoptosis is an important mechanism of immune-system homeostasis during lymphoid development and in the clearance of effector cells after immune activation143. Mammalian glycosylation contributes to this process by generating ligands for endogenous lectins, including galectins57,144,145. These lectins bind to glycans that contain the disaccharide lactose and to polylactosamine, which are present in some cellular glycoproteins and glycolipids.
The T-cell lineage is sensitive to both the endogenous expression and exogenous administration of galectin, and most studies have examined the effects of galectin-1 and galectin-3. Exogenously administered galectin-1 was first reported to induce apoptosis in thymocytes, T-cell lines and activated T cells146. The in vitro methodology used was thought to contribute in part to this result, as a subsequent study reported that activated T cells do not normally undergo apoptosis in response to galectin-1, but become apoptotic after administration of galectin-3 (REFS 147,148). In multiple cell types, including T cells and neutrophils, binding of galectins -1, -2, -3, -4 or -8 to cellular glycans was shown to induce phosphatidyl-serine exposure on the outer leaflet of the plasma membrane, which is associated with cellular apoptosis with phagocytic uptake in vivo, and normal cell viability was retained in vitro147–150. Galectins bind to glycan ligands that are present on various glycoproteins, such as CD2, CD3, CD7 and CD45, thereby forming glycoprotein complexes that induce intracellular signalling cascades and inhibit IFNγ and IL-2 production and IL-2-mediated signalling148,151–154. Among the glycan ligands of the galectins, the polylactosamine moieties that are found in O-glycans are sometimes present on the core 2 branch. T-lymphoma cells that are deficient in core 2 O-glycans as a result of GCNT1 mutation or haploinsufficiency are resistant to galectin-1-induced apoptosis155. In addition, some galectins seem to oppose the functions of others. Galectin-3 binds different glycans and glycoproteins to those that are recognized by galectin-1, and galectin-3 can inhibit galectin-1- but not galectin-9-mediated apoptosis of some human T-cell lines. By contrast, galectin-9 induces T-cell apoptosis that can be inhibited by B-cell lymphoma 2 (BCL-2) (REFS 156–158).
The ST3Gal1 sialyltransferase seems to control the largest and most synchronous apoptotic response in adult mammals, that of eliminating the increase in cytotoxic CD8+ T cells that results from an immune response to infection by various microorganisms and viruses. More than 90% of the clonally expanded CD8+ T-cell population must be rapidly cleared by apoptosis during the resolution of the immune response so that the body is not overcome by lymphocytosis. ST3Gal1 is involved in this process by linking sialic acid in an α2,3 linkage to the core 1 O-glycan of one or more O-glycoproteins. Attenuation of ST3Gal1 function by a post-translational mechanism occurs during the resolution phase of the immune response, and the resulting unsialylated core 1 O-glycan structure is linked to the onset of post-activated CD8+ T-cell apoptosis by a caspase-dependent mechanism that seems to dominate the effects of the intracellular apoptotic modulators BCL-2 and BIM109,159. Remodelling of the glycome in activated CD8+ T cells by downmodulation of ST3Gal1 function induces a signalling pathway that ensures apoptotic cell death in the absence of TCR stimulation and of factors that induce memory-cell differentiation. The lectin (or lectins) that induces post-activated CD8+ T-cell apoptosis in vivo, and the glycoprotein counter-receptor (or receptors) that transmits the apoptotic signal following binding to the unsialylated core 1 O-glycan ligand, are yet to be identified.
Summary and future directions
A rapid expansion of our knowledge of the immunological roles of mammalian glycosylation has resulted from interdisciplinary studies that combine biochemical, enzymatic, structural and genetic techniques. Establishing the relationships between glycan linkages, the enzymes that produce them, and the glycoproteins that are modulated by them remains at the forefront of research (TABLE 1). Studies that bridge immunology and glycobiology, and which link the enzymes of glycosylation with lectin recognition, will continue to provide new insights into the immune system and will reveal new approaches to therapeutic intervention in disease. Studies of glycosylation by the ST3Gal4 sialyltransferase, for example, recently revealed that the Ashwell–Morell receptor of hepatocytes — the main lectin expressed by hepatocytes — mitigates the lethal coagulopathy of sepsis160, exposing an unexpected pathogen–host relationship that might reflect an ancient arm of the innate immune system.
Table 1
Mammalian glycan structures that are linked to immune functions
Glycan* | Enzymes‡ | Function | Recognition§ | |
---|---|---|---|---|
sLex | ![]() | ST3Gal4, ST3Gal6, β1,4GalT1, Fut4 and Fut7 | Trafficking, adhesion and signalling | E- and P-selectin |
6-sulpho-sLex | ![]() | ST3Gal4, GlcNAc6ST1, GlcNAc6ST2, Fut4 and Fut7 | Lymphocyte homing | L-selectin |
Core 1 O-glycan | ![]() | ST3Gal1 | CD8+ T-cell apoptosis | Unknown |
Terminal GlcNAc | ![]() | GnTs | IgA nephropathy and complement activation | Integrins |
N-acetyl-lactosamine and polylactosamine | ![]() | β1,4GalTs and β1,3GnTs | T-cell-mediated tumour rejection, fetal–maternal tolerance and DC function | Galectins |
β1,6 N-glycan branch | ![]() | GnT5 | TCR activation threshold | Galectin-3 |
Sia6LacNAc | ![]() | ST6Gal1 | BCR activation threshold | CD22 |
Hybrid N-glycan | ![]() | αM-II | Deficiency causes SLE-like syndrome in mice | Mannose-binding lectin (or lectins) |
O-fucose glycan | ![]() | β1,3GlcNAcTs and Pofut1 | Lymphocyte development and activation, myeloid and DC development, and NK-cell toxicity | Notch |
O-glucose glycan | ![]() | O-glucosyltransferase (Rumi) | Notch signalling | Notch |
Hyaluronan | ![]() | Hyaluronan synthases | Innate immunity, response to infection | TLR2 and TLR4 |
N-glycan | ![]() | Fut8 | TGFβ1 receptor ligand binding and signalling | TGFβ1 |
The mechanisms by which glycans and lectins contribute to immunity are only now beginning to be understood. Among the potential mechanisms, the nature of multivalent lectin–ligand binding as observed in vitro indicates that higher-order structures might exist among glycoproteins and lectins at the cell surface161. Further study could resolve whether galectins normally operate in vivo to construct a repetitive and spatially precise ultrastructure (a lattice) that thereby modulates the presentation, interaction, function and turnover of glycoproteins161–166. There is increasing evidence that mammalian glycosylation controls the rate of receptor endocytosis as a means to modulate cellular responses through the interaction of lectins and their ligands167–169. It seems possible that what is presently considered basal protein-turnover activity at the cell surface is instead a highly orchestrated process that controls glycoprotein fate and function. Furthermore, it remains probable that more lectins without canonical lectin-like domains exist, which are identifiable as proteins that bind their targets and function in a glycan-dependent manner. It follows that glycan-dependent regulation of glycoprotein and glycolipid interactions might contribute in many contexts and in presently unknown ways to the mechanisms that govern the immune responses of cells to various extracellular stimuli.
The evolutionary success of the selectins and their glycan ligands in regulating immune function by controlling cell–cell adhesion and signalling makes it unlikely that they are the only lectin–ligand system that has evolved to direct immune-cell trafficking. Other glycan structures, such as Lewis X and Lewis Y, for example, have recently been found to participate in the cell–cell recognition that modulates dendritic-cell adhesion and signalling170,171. Moreover, hyaluronan has been recently found to modulate neutrophil adhesion in liver sinusoids172. The extent to which glycosylation contributes to immune-cell recognition is uncertain, but the regulated changes that occur in glycan formation during cell maturation and activation are profound and may alter the production of various lec-tin ligands that might engage various molecules and cell types173. Even the glycosyltransferases that participate in selectin-ligand synthesis have yet to be fully identified, and the regulatory events that control the formation of selectin ligands are incompletely understood. In addition, the mechanisms by which selectin ligands differentially affect the immune response, including their ability to modulate intracellular signalling, are just beginning to be studied.
Glycosylation in mammals constructs a glycome that can be distinguished phylogenetically and immunologically from those of lower and divergent organisms, and encompasses glycan linkages with profound immunoregulatory functions. Improved experimental techniques that can rapidly define glycan structure and can image distinct cellular glycoprotein interactions are increasingly relevant. Antibodies that specifically bind the synthetic and degradative enzymes of glycosylation are for the most part not available today, which makes it difficult to detect the molecular interactions that constitute the regulatory machinery of cellular glyco-sylation. Determining the elements of the mammalian glycome and the mechanisms by which the glycome is constructed and regulated will provide integrative and profound immunological insights into the biological roles of mammalian glycosylation.
Acknowledgments
We apologize to the colleagues and researchers who have made contributions that were not referenced owing to space limitations. J.D.M. is supported by the Howard Hughes Medical Institute and grants from the National Institutes of Health, USA (HL57345, HL78784 and GM62116).
Glossary
- Glycome
- The biological repertoire of glycan structures
- Experimental autoimmune encephalomyelitis
- An experimental model of multiple sclerosis. Autoimmune disease is induced in animals by immunization with myelin or peptides derived from myelin. The animals develop a paralytic disease with inflammation and demyelination in the brain and spinal cord
- Immunoreceptor tyrosine-based inhibitory motif
- (ITIM). A short amino-acid sequence (the consensus sequence of which is V/IxYxxV/L, in which x denotes any amino acid) that is found in the cytoplasmic tail of inhibitory receptors. ITIMs are thought to mediate inhibitory signalling by recruiting phosphatases such as sHP1 (sRC-homology-2-domain-containing protein tyrosine phosphatase 1)
- Clathrin-enriched microdomain
- A region of the plasma membrane that is associated with clathrin-dependent endocytic mechanisms of protein trafficking and turnover
- IgA nephropathy
- An inflammatory disease of the kidney that is associated with the glomerular deposition of IgA
- Old-world primates
- A grouping of primates, which includes humans, that are native to Africa and Asia
- Henoch–Schönlein purpura
- An inflammatory disease that involves the blood vessels and causes vasculitis
- Hodgkin’s lymphoma
- A type of lymphoma that is characterized by the presence of Reed–sternberg cells (derived from B cells) and that spreads between the lymph nodes
Footnotes
DATABASES
Entrez Gene: http://www.ncbi.nlm.nih.gov/entrez/query.fcgi?db=gene
αM-II | CD22 | COSMC | E-selectin | galectin-1 | GCNT1 | L-selectin | Mgat5 | Notch | P-selectin | PSGL1 | ST3Gal4 | ST3Gal6
OMIM: http://www.ncbi.nlm.nih.gov/entrez/query.fcgi?db=OMIM
rheumatoid arthritis | systemic lupus erythematosus | Tn syndrome
FURTHER INFORMATION
Jamey D. Marth’s homepage: http://www.hhmi.org/research/investigators/marth_bio.html
References
Full text links
Read article at publisher's site: https://doi.org/10.1038/nri2417
Read article for free, from open access legal sources, via Unpaywall:
https://www.nature.com/articles/nri2417.pdf
Citations & impact
Impact metrics
Article citations
New insights into allergic rhinitis treatment: MSC nanovesicles targeting dendritic cells.
J Nanobiotechnology, 22(1):575, 19 Sep 2024
Cited by: 0 articles | PMID: 39294599 | PMCID: PMC11411834
Sugar-Coated: Can Multivalent Glycoconjugates Improve upon Nature's Design?
J Am Chem Soc, 146(40):27215-27232, 28 Sep 2024
Cited by: 0 articles | PMID: 39340450 | PMCID: PMC11467903
Review Free full text in Europe PMC
Microbiome-Stealth Regulator of Breast Homeostasis and Cancer Metastasis.
Cancers (Basel), 16(17):3040, 31 Aug 2024
Cited by: 0 articles | PMID: 39272898 | PMCID: PMC11394247
Review Free full text in Europe PMC
The role of galectins in the regulation of autophagy and inflammasome in host immunity.
Semin Immunopathol, 46(3-4):6, 23 Jul 2024
Cited by: 0 articles | PMID: 39042263
Review
Add-On Bifidobacterium Bifidum Supplement in Children with Attention-Deficit/Hyperactivity Disorder: A 12-Week Randomized Double-Blind Placebo-Controlled Clinical Trial.
Nutrients, 16(14):2260, 13 Jul 2024
Cited by: 0 articles | PMID: 39064703 | PMCID: PMC11279422
Go to all (383) article citations
Other citations
Data
Data behind the article
This data has been text mined from the article, or deposited into data resources.
BioStudies: supplemental material and supporting data
Protein structures in PDBe
-
(1 citation)
PDBe - 3GnTView structure
Similar Articles
To arrive at the top five similar articles we use a word-weighted algorithm to compare words from the Title and Abstract of each citation.
Coupling pathogen recognition to innate immunity through glycan-dependent mechanisms.
Int Immunopharmacol, 11(10):1457-1463, 18 May 2011
Cited by: 45 articles | PMID: 21600310
Review
Modulation of cellular tropism and innate antiviral response by viral glycans.
J Innate Immun, 1(5):405-412, 25 Jun 2009
Cited by: 15 articles | PMID: 20375598 | PMCID: PMC3214953
Review Free full text in Europe PMC
Glycosylation in cellular mechanisms of health and disease.
Cell, 126(5):855-867, 01 Sep 2006
Cited by: 1469 articles | PMID: 16959566
Review
Bacterial glycans and their interactions with lectins in the innate immune system.
Biochem Soc Trans, 47(6):1569-1579, 01 Dec 2019
Cited by: 17 articles | PMID: 31724699
Review
Funding
Funders who supported this work.
NHLBI NIH HHS (6)
Grant ID: P01 HL078784
Grant ID: HL57345
Grant ID: HL78784
Grant ID: P01 HL078784-059001
Grant ID: P01 HL057345
Grant ID: P01 HL057345-050001
NIAID NIH HHS (1)
Grant ID: R01 AI050143
NIGMS NIH HHS (4)
Grant ID: R01 GM060938
Grant ID: U54 GM062116
Grant ID: GM62116
Grant ID: U54 GM062116-05