Abstract
Free full text

Diabetogenic T cells recognize insulin bound to IAg7 in an unexpected, weakly binding register
Abstract
A peptide derived from the insulin B chain contains a major epitope for diabetogenic CD4+ T cells in the NOD mouse model of type 1 diabetes (T1D). This peptide can fill the binding groove of the NOD MHCII molecule, IAg7, in a number of ways or “registers.” We show here that a diverse set of NOD anti-insulin T cells all recognize this peptide bound in the same register. Surprisingly, this register results in the poorest binding of peptide to IAg7. The poor binding is due to an incompatibility between the p9 amino acid of the peptide and the unique IAg7 p9 pocket polymorphisms that are strongly associated with susceptibility to T1D. Our findings suggest that the association of autoimmunity with particular MHCII alleles may be do to poorer, rather than more favorable, binding of the critical self-epitopes, allowing T-cell escape from thymic deletion.
The development of type 1 diabetes (T1D) in NOD mice and humans is associated with certain alleles of MHCII (1–3). These alleles present sets of peptides distinct from those presented by other MHCII alleles (4, 5), thus their association with T1D may be because they are better than other MHCII alleles at presentation of peptides derived from pancreatic islet β-cell proteins (6). However, tests of this and other hypotheses about the role of MHCII in T1D require a precise identification of the peptides recognized by pathogenic CD4+ T cells.
Peptides bind in an extended conformation to a groove of MHCII. The binding involves a nonamer of the peptide (p), in which the side chains of “anchor” amino acids at positions p1, p4, p6, and p9 interact with corresponding pockets in the groove (7). Polymorphic MHCII residues lining the binding groove influence the preference of each pocket for particular peptide side chains, providing each MHCII allele with a unique preferred peptide-binding motif that dictates the position or “register” of the peptide in the groove. However, the specificities of these pockets are not absolute, and so a peptide may bind in more than one register, with the hierarchy of registers determined by how well the pockets accept the anchor residues. The register of the peptide affects which of its side chains are pointing out of the MHCII groove and hence interaction with T cell receptors. Thus individual peptide-responsive T cells will recognize the peptide bound to MHCII in only one of the possible registers.
The NOD mouse has a single MHCII molecule, IAg7, which is essential for the development of disease (8). Polymorphisms in the IAg7 β-chain (9) shape the p9 binding pocket (10, 11) and contribute to a side chain preference distinct from that of other IA alleles (6). In particular, an Asp-to-Ser alteration at IAg7 β57 disrupts a conserved salt bridge to an Arg at α76. This allows the positively charged Arg to interact with the peptide amino acid side chain in the p9 pocket (11) and confers a unique preference for binding peptide registers with acidic residues at this position (6).
The insulin B-chain peptide (B:12–23) is recognized by diabetogenic CD4+ T cells in the NOD mouse (12–14) and might bind IAg7 in any of four registers. Previous studies have suggested that individual diabetogenic T cells might recognize the peptide in either of two of these registers (15). Of the two remaining registers, one, called register 3 in this study, is predicted to be the least favored of the four possibilities, because it would place an overtly conflicting positively charged arginine side chain in the positively charged p9 pocket.
Here we used an approach we term “register trapping,” in which p1 and p9 were optimized for binding to IAg7, to determine which binding registers of the insulin peptide are recognized by a collection of insulin-specific NOD CD4+ T cells. Surprisingly, these T cells all recognized the insulin peptide bound in register 3. IAg7-binding studies with core nonamer peptides confirmed that register 1 and 2 peptides bound to IAg7 without anchor optimization, but that the register-3 peptide bound very poorly unless the anchors were changed.
Thus, our findings show that the dominant, diabetogenic CD4+ T-cell response in NOD mice targets insulin bound to IAg7 in an unfavorable register. This finding suggests that pathogenic autoreactive CD4+ T cells often target self-antigen epitopes resulting from peptides bound to MHCII in registers with unfavorable binding motifs (16). The combination of poor binding and competition by peptides bound in other registers may prevent sufficient presentation of the critical epitope for thymic deletion, allowing autoreactive T cells targeting these self-antigens to escape negative selection. In the periphery, however, high local expression or modification (17) of the antigen might overcome poor presentation by MHCII, permitting pathogenic T cells to respond to their self-antigen targets and initiate autoimmunity.
Results
Diabetogenic CD4+ T Cell Recognizes Insulin B:12–23 Bound in an Unfavored Register.
The insulin B:12–23 peptide may bind to IAg7 in any of four registers (Fig. 1A). Previous data has suggested that registers 1 and 2 may be most often used by diabetogenic T cells, but that register 3 is disfavored, because of a charge clash between the register 3 arginine at p9 and the positively charged p9 binding pocket of IAg7 (15). To be able to study the registers separately, we optimized the peptide amino acids for binding to IAg7, choosing an Arg and Glu at the predicted p1 and p9 positions, respectively, for each of the four registers (Fig. 1B and Fig. S1)—a process we call register trapping. These choices were based on the solved structures of IAg7 bound to peptides from either hen egg lysozyme (HEL) (10) or glutamic acid decarboxylase (GAD; Fig. S2 A and B) (11). Besides the nine peptide amino acids that occupy the core of the MHCII groove, amino acids at p−1 are also sometimes important, so peptide constructs also included the relevant amino acid at p−1. Additionally, to avoid potential problems with an unpaired Cys, the B:19 Cys of the peptide was altered to Ala. This mutation does not diminish, and may even enhance, recognition by some T cells (18) (Fig. S3).
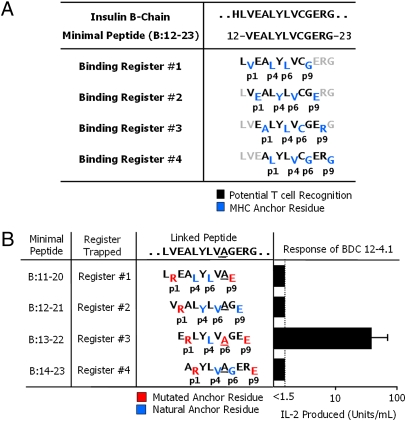
The BDC 12-4.1 T cell responds to register 3 of the insulin B:12–23 peptide. (A) Four possible IAg7-binding registers for the mouse insulin B-chain peptide, B:12–23. Anchor residues are shown in blue, and black residues show the amino acids that potentially contact the T-cell antigen receptor. (B) Minimal 10-mer linked peptide versions of the insulin B:12–23(19A) epitope. Each minimal peptide was trapped in a single binding register by a p1Arg and a p9Glu. The substitution of Ala for Cys at B:19 of the peptide is underlined. Red amino acids are the substituted anchor residues. Blue amino acids are the naturally occurring anchor residues. Black amino acids are the possible T-cell epitope residues. Also shown is the stimulation of the BDC 12-4.1 T-cell hybridoma with each of the individual IAg7-linked peptide complexes expressed on the surface of ICAM/B7+ insect cells, assessed by the production of IL-2. Results are the average ± SEM of triplicate wells from five independent experiments.
We constructed a set of four overlapping 10-mer versions of the insulin B:12–23 minimal peptide, each optimized at p1 and p9 for binding to IAg7, whose C termini were covalently attached via a flexible linker to the N terminus of an insect cell anchored version of IAg7 β-chain (19) (Fig. 1B and Fig. S1). Each construct was cloned into baculovirus, and the viruses were used to infect SF9 insect cells. All of the recombinant IAg7 complexes expressed well on the surface of the infected insect cells (Fig. S4A). We assessed the response of a T-cell hybridoma expressing the TCR of the diabetogenic, IAg7/insulin-specific T cell, BDC 12-4.1, to ICAM/B7+ insect cells (20) bearing the IAg7-linked peptide complexes (Fig. 1B). Unexpectedly, only the complex that trapped the peptide in register 3 stimulated. This result was surprising because, as stated previously, for the natural insulin peptide in this register, the p9 pocket would have to be occupied by a conflicting Arg (6) (Fig. 1A).
Three Other CD4+ T cells Recognize the Insulin Peptide in Register 3.
To find out if BDC 12-4.1 was exceptional in its recognition of IAg7/insulin, we analyzed T-cell hybridomas bearing the TCRs from three other islet-infiltrating insulin-responsive CD4+ T cells, AS91, AS150, and I.29 (15). The TCRs on these three T cells differed in sequence from each other and from BDC 12-4.1 (Fig. S5). Based on mutational and truncational analyses of the insulin peptide, AS91 and AS150 were thought to recognize the insulin peptide bound to IAg7 in register 1 or 2, respectively, whereas the specificity of I.29 had not been previous analyzed. Moreover, unlike BDC 12-4.1, studies with AS91 and AS150 suggested that they required peptides that extended N terminally to B:10 (15). Therefore, we generated IAg7 complexes with the extended B:10–23 peptide trapped in register 1, 2, or 3. Once again, the optimized anchors of Arg at p1 and Glu at p9 were used to trap the peptide in each register, but in this case, the natural Cys at B:19 of the peptide was present (Fig. 2 and Fig. S6). For comparison, we also generated an IAg7 complex linked to the unmodified B:10–23 peptide. As before, all of the recombinant complexes expressed well on the surface of insect cells (Fig. S4B).
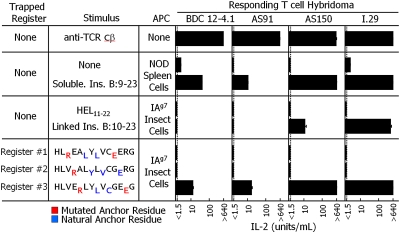
Four independent insulin B-chain responsive T cells recognize the peptide bound to IAg7 in register 3. Triplicate culture wells of the BDC 12-4.1, AS91, AS150, and I.29 insulin B-chain responsive CD4+ T-cell hybridomas were stimulated with an immobilized anti-Cβ Mab (five experiments), NOD spleen cells with or without 50 μg/mL of soluble insulin B:9–23 peptide (two experiments), ICAM/B7+-infected insect cells expressing IAg7 linked to the control hen egg lysozyme (HEL) peptide or to the unmutated B:12–23 peptide (three experiments), or ICAM/B7+-infected insect cells expressing IAg7 linked to each of three trapped versions (Fig. S6) of the insulin B:10–23 peptide (three experiments). The responses of the hybridomas were assessed by IL-2 production, and the average response and SEM is shown.
ICAM/B7+ insect cells expressing the complexes and various controls were assessed for their ability to stimulate the four T-cell hybridomas (Fig. 2). All four hybridomas responded maximally to immobilized anti-Cβ Mab (21). Thus they had approximately the same response to strong TCR cross-linking. Also, all four hybridomas responded to a soluble form of the natural insulin peptide presented by NOD spleen antigen-presenting cells (APCs). BDC 12-4.1 and AS91 responded weakly, whereas AS150 and I.29 responded strongly, suggesting differences in the affinity of these T cells for the IAg7 peptide complex. None of the hybridomas responded to IAg7 linked to an irrelevant HEL peptide. The more strongly reactive AS150 and I.29 responded to insect cells presenting IAg7 linked to the unmutated B:10–23 peptide, but the more weakly reactive BDC 12-4.1 and AS91 did not, presumably reflecting the heterogeneous peptide registers and the better antigen presentation function of the natural splenic APCs compared with the insect cells. Most importantly, when tested with IAg7 linked to each of the three register-trapped versions of the B:10–23 peptide, none of T cells responded to either register 1 or 2, but all responded to the register 3 version just as well as they did to the soluble insulin peptide presented by NOD APCs. Thus all these hybridomas recognized the insulin peptide bound to IAg7 in register 3, even though previous studies have suggested otherwise (15).
Trapping Dramatically Improves Insulin Peptide Binding in Register 3.
Because these findings were unexpected, we tested whether our register-trapping strategy had actually improved peptide binding in register 3. We synthesized soluble minimal-core nonamer insulin peptides corresponding to registers 1, 2, and 3 with either the natural anchors at p1 and p9 or with the p1 and p9 positions optimized to Arg and Glu, respectively. To avoid peptide dimerization, we changed the B19 Cys to Ala in all of the peptides. We accessed the ability of the peptides to bind to IAg7 based on their relative ability to inhibit the binding of a biotinylated HEL peptide to soluble IAg7. The unbiotinylated HEL peptide was used as a positive control and a peptide from moth cytochrome c (MCC) was used as a negative control. The results of a typical experiment are shown in Fig. 3A. The inhibition curves from this and two other experiments were combined to calculate the average inhibitory power of each peptide compared with the HEL peptide (Fig. 3B).
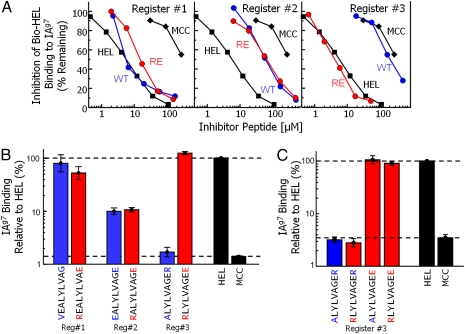
The p9 Arg-to-Glu mutation dramatically improves the binding of a register 3 peptide to IAg7. (A) Various concentrations of either unmutated (blue) or p1Arg/p9Glu (red) versions of the minimal nonamer peptides for registers 1, 2, and 3 of the B:12–23(19A) peptide were compared with a HEL peptide (positive control, black squares) and an MCC peptide (negative control, black diamonds) for the ability to inhibit the binding of a biotinylated HEL peptide to soluble IAg7. The panels show the percent of biotinylated HEL peptide remaining bound to IAg7 vs. the dose of inhibitor. The results are for a representative experiment. (B) The experiment described in A was performed three times. In each experiment the inhibition curve for each peptide was compared with the curve obtained with the HEL peptide to determine its IAg7 binding ability relative to HEL expressed as a percent. The results presented are the average value for the three experiments with the SEM. Blue bars, unmutated peptides; red bars, p1Arg/p9Glu peptides; black bars, control peptides. (C) The relative ability of the unmutated register-3 nonamer peptide to bind to IAg7 (blue bar) was compared with register-3 peptides bearing p1Arg and/or p9Glu (red bars) and to the HEL and MCC control peptides (black bars), as in A and B. The results are the average ± SEM of three experiments.
The HEL peptide was about 50- to 100-fold better than the MCC peptide in binding to IAg7. The register 1 peptide bound IAg7 nearly as well as the HEL peptide, regardless of whether its p1 and p9 anchors were optimized. The register 2 peptide bound to IAg7, but only about 1/10th as well as the register 1 peptide, despite its natural optimal Glu anchor at p9. Its binding was not improved by introducing the p1 Arg anchor. Perhaps this peptide binds suboptimally because, as previously suggested, its Tyr at p4 may fit poorly into the p4 pocket of IAg7 (22). As predicted, the register 3 peptide with its natural anchors bound IAg7 very poorly. Importantly, optimization of the register 3 anchors to p1Arg and p9Glu dramatically improved its binding to IAg7 binding. These results confirmed the conclusions of Levisetti et al. (15) that the preferred binding registers for the insulin peptide are registers 1 and 2, and also demonstrated that the register-trapping strategy converts the register 3 peptide from a very poorly to a very strongly IAg7-binding peptide.
The properties of the IAg7 peptide- binding pockets predict that in the optimized register 3 peptide, the Arg-to-Glu mutation at p9 should be much more important than the Ala-to-Arg mutation at p1. To test this prediction we compared the IAg7 binding of the unmutated and doubly mutated peptides to two additional nonamer peptides, in which only one of the two mutations was introduced. The single mutation of p1 Ala to Arg did not improve binding, but the peptide with just the Arg-to-Glu mutation at p9 bound as well as the doubly mutated peptide, again confirming the rationale for fixing the register-3 binding by the p9 mutation (Fig. 3C).
Trapping Confirmed by Disulfide Bond Formation Between the Peptide and IAg7.
To exclude the possibility that the p1Arg/p9Glu version of the register 3 peptide was bound and presented by IAg7 in some unexpected way, we engineered cysteines both into the peptide, covalently linked to the IAg7 β-chain, and into the IAg7 α-chain such that the two cysteines should form a disulfide only were the peptide trapped in register 3. Based on the solved crystal structure of IAg7 bound to a GAD peptide (10), we identified two different sites for these cysteines. First, for the natural B:10–23 peptide, binding in register 3 would place its cysteine (B:19) in the p6 pocket of IAg7 where it could disulfide bond to a Cys substituted for IAg7 αAsn62 (Fig. 4A). Alternately, a Cys substitution at αAsn72 could form a disulfide with a Cys placed at the p11 position of the peptide/linker (Fig. 4B), as previously described for MHCI plus peptides (23).
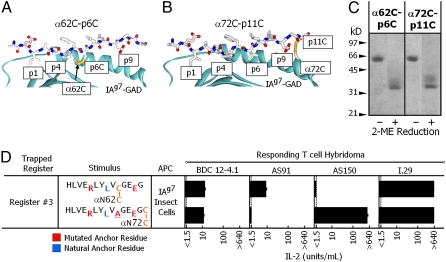
Confirmation by disulfide bond formation that the insulin peptide is trapped in register 3. The structure of IAg7 bound to a GAD peptide (PDB ID code 1ES0) (11) was used to model (Swiss PDB Viewer) (39) cysteines replacing the amino acids either at peptide p6 and IAg7 α62Asn (A) or at peptide p11 and IAg7 α72Ile (B). In both cases, the distance and orientation of the cysteines predict the formation of a disulfide bond. (C) The stimulating register 3 complex in Fig. 2 was altered to remove its baculovirus gp64 transmembrane anchor. The complex was mutated to introduce a Cys into the IAg7 α chain at either 62Asn or 72Ile. The peptide-encoding region of the α72Ile>Cys complex was further mutated to convert the Cys at p6 to Ala and the Ala at p11 to Cys (Fig. S6). Infected insect cells were infected with baculovirus encoding for each complex and the soluble IAg7 purified from the culture supernatant. The soluble IAg7 complexes were analyzed by SDS/PAGE under nonreducing and reducing conditions. The gels were stained with Coomassie blue. (D) The baculovirus gp64 transmembrane anchor was restored to each complex shown in C, allowing expression via baculovirus on ICAM/B7+ insect cells, which were used to stimulate the four T-cell hybridomas. The responses were assessed by IL-2 production, and the average of three experiments with triplicate wells are shown with the SEM.
To find out if the predicted disulfide bonds formed, we modified the register 3-trapped peptide to introduce these pairs of cysteines and analyzed soluble forms of both complexes by SDS/PAGE (Fig. 4C). Normally, the α- and β-chains of soluble MHCII molecules readily dissociate when boiled in SDS/PAGE sample buffer. However, in these complexes, because the peptide is already covalently linked to the IAg7 β-chain, a disulfide bond between the α-chain and the peptide should prevent dissociation unless the complex is intentionally reduced. In the absence of reduction, virtually all of the register 3-trapped IAg7 complexes from both constructions migrated with an apparent mass of ~64 kDa, the predicted MW of the disulfide-linked αβ IAg7 heterodimer. Upon reduction, these complexes separated into individual lower MW chains, proving the higher MW complex was due to the formation of a disulfide bond. These results confirmed that the optimized anchor residues had trapped nearly all of the peptide in register 3.
Finally, we tested the ability of the surface-bound versions of these disulfide-linked complexes to stimulate the four T-cell hybridomas. Both complexes yielded good surface expression of IAg7 on the insect cells (Fig. S4C). All four hybridomas responded to at least one of the complexes, confirming that they indeed recognized the peptide bound in register 3 (Fig. 4D). However, AS150 failed to respond to the p6-α62 disulfide-linked complex, and AS91 failed to respond to the p11-α72 disulfide-linked complex, despite the fact that they both responded to the nondisulfide version. These failures probably occurred because, even when bound in the appropriate register, local constraints in the exact conformation of the peptide backbone affected the fine structure of the surface-exposed portion of the peptide detected by some, but not all, T cells (24).
Discussion
A number of hypotheses have been suggested to account for the linkage between MHCII polymorphisms and autoimmunity in general and between IAg7 and T1D in the NOD mouse in particular. The longest-standing idea is that IAg7 binds and presents the relevant pancreatic antigenic peptides, including those from insulin, better than other MHCII alleles do (6). An alternate hypothesis suggests that IAg7 binds peptides in general less well than other IA alleles do because the structure of its p9 pocket destabilizes binding of many peptides, leading to a general decrease in presentation of self-antigens during thymic negative selection, allowing autoimmune T cells to escape into the periphery (25, 26).
Our data led us to propose a third hypothesis, that rather than having a generalized peptide-presentation defect, IAg7 presents just the relevant autoimmune epitopes poorly. This notion is not tenable if the target of diabetogenic T cells in NOD T1D is the B:9–23 insulin peptide in the favored registers, 1 or 2 (15). However, the results shown here, demonstrating that the target of four unrelated, diabetogenic, insulin-specific T cells, two of which were previously thought to bind B:12–23 in registers 1 and 2, is actually B:12–23 bound in the unfavored register 3, strongly support this third idea. Thus we conclude that T cells recognizing register 1 and 2 actually do not contribute to disease, presumably because they have been effectively deleted by sufficient antigen presentation in the thymus, whereas those recognizing register 3 escape because of poor thymic presentation. Because similar polymorphisms that directly affect peptide binding are also present in human T1D susceptibility MHCII alleles (1, 4, 22), our findings in the NOD mouse raise the possibility that they may play the same role in T1D patients.
The suggestion that poor presentation of particular peptides leads to the escape of autoreactive T cells from the thymus raises the question of how these T cells overcome this problem to cause disease peripherally in the pancreas. There are a number of possibilities. The simplest idea might be that the difference lies in antigen dose. Insulin is expressed in the thymus, regulated in part by the transcriptional regulator Aire (27). Apparently, this level of expression is insufficient for effective tolerance in NOD mice. However, overexpression of proinsulin in NOD mice via a transgene prevents the development of T1D, presumably due to a better level of presentation in the thymus (28). In the pancreas, insulin expression is extremely high. This increased dose alone might push register-3 insulin presentation to the threshold required to activate T cells that have escaped thymic deletion, thus initiating T1D.
Second, some difference in peripheral processing and/or interaction with IAg7 might improve presentation of insulin in register 3 in the pancreas. Such differences could be caused by destruction of registers 1 and/or 2, but not register 3, by differential processing in peripheral tissues, leaving register 3 to bind IAg7 uncompeted. Finally, Unanue and coworkers (29, 30) have reported that even with a given peptide-binding register, peptide conformation is not homogeneous. They describe two peptide/MHCII conformations, A and B, dependent on the pathway of peptide loading, which can be discriminated by individual T cells (29). Their recent paper (30) suggests that pathogenic T cells in NOD T1D recognize the B form of insulin bound to IAg7 and that this form is preferentially generated in the pancreas. Therefore a difference between the ratio of these two forms between thymus and pancreas could explain the peripheral activation of thymic escapees.
There are other examples of self-peptides involved in autoimmunity that bind to MHCII in unusual ways and/or are recognized by CD4+ T cells in an unconventional way. For example, WE14, a 14-aa peptide that results from the natural processing of the β-cell granule protein chromogranin A (31), is bound to IAg7 such that only its first five amino acids lie in the binding groove, filling positions p5–p9. The other nine amino acids extend from the binding groove and contribute to IAg7 binding in some essential, but unknown, fashion, providing an example of an autoantigen that might require tissue-specific processing to generate the relevant epitope. Another example is the myelin basic protein peptide recognized in mouse experimental autoimmune encephalomyelitis in IAu mice (16, 32). Like the WE14 peptide, when recognized by CD4+ T cells, this peptide only partially fills the antigen-binding groove of IAu. Finally, Li et al. (33) have shown the very unusual engagement of a complex of HLA-DR51 and a myelin basic protein peptide by a human TCR. The TCR footprint is extremely tilted toward the N-terminal end of the peptide, interacting with amino acids outside of the binding groove at p−1, p−2, and p−4, and lacking the usual engagement with the MHCII α-helices and center of the peptide. Together, these findings suggest that T-cell thymic tolerance to self-antigens may be in general very efficient, and that only rare antigens that are presented or recognized in unconventional ways allow the development of autoimmunity.
It is not immediately apparent why previous mutational and truncation data indicated register 1 or register 2, rather than register 3, to be the recognized binding registers for the insulin peptide in NOD T1D (15). It is possible that competition among the binding registers obscured the interpretation of the results of the previous experiments. The most surprising data in this previous paper was that some T cells, including AS91 and AS150, responded to some versions of the peptide that lacked the p9Arg of register 3, leaving peptides that would not completely fill the binding groove. However, as discussed previously, there is precedent for autoimmune T-cell recognition of foreshortened self-peptides. Moreover, perhaps more is gained, in terms of register-3 recognition, by eliminating the unfavored p9Arg than is lost by the absence of the p9 anchor altogether.
Finally, our findings illustrate the difficulty in determining the relevant mode of MHCII binding of self-peptides involved in autoimmunity, especially when peptides can shift and bind in multiple overlapping registers. Traditional truncation/mutation approaches may not be sufficient to identify the actual epitopes, because inferior binding registers may have to compete with irrelevant overlapping favorable binding registers (16, 34). In these cases, additional approaches, such as the register-trapping and disulfide formation approaches described herein may be needed to supply more direct evidence for the relevant binding registers recognized by self-reactive T cells. This approach may also be useful in defining the functional register of conventional foreign antigen peptides that are known to bind to an MHCII molecule in multiple registers.
Materials and Methods
T-Cell Hybridomas.
The BDC 12-4.1 T-cell hybridoma was created by fusing BW5147 α/β T cells with BDC 12-4.1 RAG−/− splenocytes (18). The AS91 and AS150 T-cell hybridomas (15), as well as the I.29 T-cell hybridoma, were generous gifts from Matteo Levisetti (University of Washington, St. Louis). T-cell hybridomas were grown in modified SME media (35) (Invitrogen).
IAg7-Linked Peptide Complexes.
The IAg7 α- and β-chains were placed under the p10 and pH promoters in the pBacp10pH baculovirus transfer vector, respectively (19, 20, 36). The α-chain was terminated following residue α182E to remove the transmembrane domain, and attached to a basic leucine zipper (37). The β-chain contained a peptide with a C-terminal flexible linker (GGGSLVPRGSGGGGS) inserted between the signal peptide and the N terminus of the β1 domain. The β2 domain was truncated at residue β190Q and attached to an acid leucine zipper (37) followed by the baculovirus gp64 transmembrane domain. Soluble versions of the complexes were achieved by removal of the gp64 transmembrane domain on the β-chain and the addition of the TCRCα epitope tag (DATLTEKSFETDMNLNFQNLSVN) to the C terminus of the α-chain.
Generation of Recombinant Baculovirus.
IAg7-containing baculoviruses were generated by homologous recombination between the transfer vector and linearized Safire baculovirus DNA (Oribigen) using the manufacturer's calcium phosphate cotransfection protocol and SF9 insect cells. A virus stock was collected after 10 d.
Expression and Purification of IAg7 Complexes.
Soluble IAg7-linked peptide complexes were produced in spinner flasks by infecting 5.2 × 108 Hi-5 cells (MOI ~3) in 250 mL plain Grace's medium. After 2 h, the culture was supplemented with 550 mL of TMN-FH media, incubated overnight at 27 °C, and then moved to 19 °C for 6 d. Supernatant was harvested and cleared of debris by centrifugation. Soluble IAg7 was immunoaffinity purified using Mab, AD0124.5 (anti-TCRCα epitope), coupled Sepharose, eluted in 50 mM carbonate buffer (pH 10.4), and adjusted to a neutral pH using 0.15 vol 1 M Tris (pH 6.9). The protein was buffer exchanged into PBS with 0.1% azide by centrifugation, Amicon Ultra 30 kDa. The resulting protein isolate was subject to FPLC size exclusion Superdex 200 chromatography (GE Healthcare) to remove aggregate or contaminating proteins.
SDS/PAGE Electrophoresis.
SDS/PAGE analysis was carried out using a 12.5% Phastgel (GE Healthcare). An aliquot containing 1 μg/μL of protein was boiled in an equal volume of 2× SDS/PAGE sample buffer with and without the addition of 2-mercaptoethanol. Approximately 0.5 μg of total protein was loaded in each lane.
T-Cell Stimulation Assays.
Viruses expressing IAg7 with a transmembrane domain were used to infect ICAM/B7+-expressing SF9 cells (20). Three days after infection the cells were used as artificial APCs. In 250 μL of complete tumor media, 1 × 105 T-cell hybridomas were cocultured with 3 × 104 infected Sf9 cells (35). After 24 h, the supernatants assayed for IL-2 using IL-2–dependent HT-2 cell line (38).
Binding of Peptides to IAg7.
The binding of peptides to soluble IAg7 was determined in a peptide competition binding assay as previously described (31). Briefly, soluble IAg7 with a covalent hen egg lysozyme peptide (aa11-22, HEL) (Fig. S6) was prepared. The purified protein was treated with thrombin to cleave at a thrombin site in the linker connecting the peptide to the IAg7 β-chain (36). Samples (0.5 μg) were incubated with a limiting amount of a soluble biotinylated version of pHEL11–22, biotin-GGGMKRHGLDNYRGYSL (11 μM), either alone or in the presence of various concentrations of potential competitors peptides in 15 μL of pH 5.3 buffer overnight at room temperature. An unbiotinylated HEL peptide was used as the positive control, and a moth cytochrome c peptide (MCC88–103) peptide was used as the negative control. The sample was diluted to 100 μL PBS in a well of a 96-well ELISA plate coated with an IAg7 monoclonal antibody, OX6 (BD Pharmaceuticals). The captured IAg7 was washed several times with PBS, and the bound bio-pHEL detected with akaline phosphatase-coupled Extravidin (Sigma) and o-nitrophenol phosphate. The binding inhibition curves were compared to calculate the binding ability of the inhibitor peptides relative to the HEL peptide.
Acknowledgments
We thank Drs. M. Levisetti and E. Unanue (Washington University, St. Louis) for generously providing the AS91, AS150, and I.29 insulin-reactive T-cell hybridomas and Drs. B. K. B Stadinski, E. Clambey, and M. Macleod for critical reading and discussions. This work was supported by National Institutes of Health Grants 5 U19-AI05086, AI-17134, AI-18785 AI050864, DK55969, and DK057516 and Juvenile Diabetes Research Foundation Grant 4-2007-1056.
Footnotes
The authors declare no conflict of interest.
This article contains supporting information online at www.pnas.org/lookup/suppl/10.1073/pnas.1006545107/-/DCSupplemental.
References
Articles from Proceedings of the National Academy of Sciences of the United States of America are provided here courtesy of National Academy of Sciences
Full text links
Read article at publisher's site: https://doi.org/10.1073/pnas.1006545107
Read article for free, from open access legal sources, via Unpaywall:
https://europepmc.org/articles/pmc2890771?pdf=render
Free after 6 months at www.pnas.org
http://www.pnas.org/cgi/content/abstract/107/24/10978
Free after 6 months at www.pnas.org
http://www.pnas.org/cgi/content/full/107/24/10978
Free after 6 months at www.pnas.org
http://www.pnas.org/cgi/reprint/107/24/10978.pdf
Citations & impact
Impact metrics
Citations of article over time
Alternative metrics

Discover the attention surrounding your research
https://www.altmetric.com/details/102382834
Smart citations by scite.ai
Explore citation contexts and check if this article has been
supported or disputed.
https://scite.ai/reports/10.1073/pnas.1006545107
Article citations
Antigen-specific T cell responses in autoimmune diabetes.
Front Immunol, 15:1440045, 15 Aug 2024
Cited by: 0 articles | PMID: 39211046 | PMCID: PMC11358097
Review Free full text in Europe PMC
The insulin secretory granule is a hotspot for autoantigen formation in type 1 diabetes.
Diabetologia, 67(8):1507-1516, 29 May 2024
Cited by: 1 article | PMID: 38811417
Review
Aire mediates tolerance to insulin through thymic trimming of high-affinity T cell clones.
Proc Natl Acad Sci U S A, 121(20):e2320268121, 06 May 2024
Cited by: 1 article | PMID: 38709934 | PMCID: PMC11098115
Genetically engineered CD80-pMHC-harboring extracellular vesicles for antigen-specific CD4+ T-cell engagement.
Front Bioeng Biotechnol, 11:1341685, 17 Jan 2024
Cited by: 0 articles | PMID: 38304104 | PMCID: PMC10833362
Tregs with an MHC class II peptide-specific chimeric antigen receptor prevent autoimmune diabetes in mice.
J Clin Invest, 133(18):e168601, 15 Sep 2023
Cited by: 17 articles | PMID: 37561596 | PMCID: PMC10503798
Go to all (149) article citations
Data
Data behind the article
This data has been text mined from the article, or deposited into data resources.
BioStudies: supplemental material and supporting data
Protein structures in PDBe
-
(1 citation)
PDBe - 1ES0View structure
Protocols & materials
Related Immune Epitope Information - Immune Epitope Database and Analysis Resource
Similar Articles
To arrive at the top five similar articles we use a word-weighted algorithm to compare words from the Title and Abstract of each citation.
Specificity and detection of insulin-reactive CD4+ T cells in type 1 diabetes in the nonobese diabetic (NOD) mouse.
Proc Natl Acad Sci U S A, 108(40):16729-16734, 26 Sep 2011
Cited by: 121 articles | PMID: 21949373 | PMCID: PMC3189014
Recognition of Multiple Hybrid Insulin Peptides by a Single Highly Diabetogenic T-Cell Receptor.
Front Immunol, 12:737428, 30 Aug 2021
Cited by: 9 articles | PMID: 34527002 | PMCID: PMC8435627
C-terminal modification of the insulin B:11-23 peptide creates superagonists in mouse and human type 1 diabetes.
Proc Natl Acad Sci U S A, 115(1):162-167, 18 Dec 2017
Cited by: 27 articles | PMID: 29255035 | PMCID: PMC5776820
A novel pathway of presentation by class II-MHC molecules involving peptides or denatured proteins important in autoimmunity.
Mol Immunol, 55(2):166-168, 27 Nov 2012
Cited by: 17 articles | PMID: 23200226 | PMCID: PMC3594457
Review Free full text in Europe PMC
Funding
Funders who supported this work.
Howard Hughes Medical Institute
Intramural NIH HHS (1)
Grant ID: Z01 AI005086
NIAID NIH HHS (10)
Grant ID: AI-17134
Grant ID: R56 AI017134
Grant ID: U19 AI050864
Grant ID: AI-18785
Grant ID: 5 U19-AI05086
Grant ID: P01 AI022295
Grant ID: R01 AI018785
Grant ID: T32 AI007405
Grant ID: AI050864
Grant ID: R01 AI017134
NIDDK NIH HHS (4)
Grant ID: DK55969
Grant ID: R01 DK055969
Grant ID: P30 DK057516
Grant ID: DK057516