Abstract
Free full text

Analysis of the IKKβ/NF-κB Signaling Pathway during Embryonic Angiogenesis
Abstract
The NF-κB signaling pathway regulates cellular growth, survival, differentiation and development. In this study, the functions of IκB kinase (IKK)β in angiogenesis during mouse development were examined. Conditional disruption of the Ikkβ locus in endothelial cells using the well-characterized Tie2-Cre transgene resulted in embryonic lethality between E13.5-15.5. Examination of the mutant embryos revealed that while deletion of Ikkβ occurred in endothelial cells throughout the embryo, only the vascular network in the fetal liver was affected. Disruption of the fetal liver vasculature was accompanied by decreased cell proliferation and increased apoptosis of hepatocytes, but hematopoiesis was not affected. Increased apoptosis was not observed outside of fetal liver in the mutant embryos. These results indicate that the IKKβ/NF-κB pathway plays a previously unappreciated role in development of the sinusoidal vasculature in the fetal liver and additionally that this pathway is critical in the crosstalk between endothelial cells and hepatocytes during mouse development.
INTRODUCTION
Blood vessels develop through two consecutive processes, vasculogenesis and angiogenesis. Vasculogenesis occurs via differentiation of endothelial cells from hemangioblasts in the blood island of the extra embryonic mesoderm. Angiogenesis is a physiological process involving the growth of new blood vessels from the endothelial cells of existing blood vessels beginning at embryonic day 8.5 in the mouse (Risau and Flamme, 1995; Risau, 1997; Mojzis et al., 2008). Vasculogenesis and angiogenesis are regulated differently. Although the exact mechanisms are still not entirely defined, both vasculogenesis and angiogenesis are driven by growth factors like vascular endothelial growth factor (VEGF) and angiopoeitins, and additionally involve cell-cell and cell-matrix interactions (Tanjore et al., 2008).
Nuclear factor-κB (NF-κB) regulates expression of a number of genes involved in immune and inflammatory responses, cellular growth, survival, differentiation and development (Chen and Castranova, 2007; Hayden and Ghosh, 2008). There are five NF-κB family members in mammals: p50 (NF-κB1), p52 (NF-κB2), p65 (RelA), c-Rel and RelB. These factors share an N-terminal Rel homology domain (RHD) responsible for both DNA binding and homo- and heterodimerization (Hayden and Ghosh, 2008). In resting cells, NF-κB complexes are retained in the cytoplasm by interaction with IκB inhibitory proteins. Upon stimulation by inflammatory signals, such as TNF-α, IL-1 or LPS, IκB proteins become phosphorylated and ubiquitinated and are subsequently degraded by the proteasome. This results in the translocation of NF-κB proteins to the nucleus where they regulate expression of target genes (Hayden and Ghosh, 2008). Phosphorylation of IκB proteins is mediated by IκB kinase (IKK). The IKK complex contains two highly homologous kinase subunits, IKKα/IKK1 and IKKβ/IKK2, and a regulatory subunit NEMO/IKKγ (Hacker and Karin, 2006). IKKβ is involved in canonical NF-κB signaling in response to inflammatory signals, while the noncanonical pathway depends on the IKKα subunit that phosphorylates NF-κB2/p100 leading to its processing to the p52 protein (Bonizzi and Karin, 2004).
The NF-κB pathway has been previously implicated in angiogenesis. VEGF gene expression can be regulated by NF-κB (Chilov et al., 1997; Ishdorj et al., 2008). Other genes that are important for angiogenesis, such as MMPs, uPA, VCAM-1, ICAM-1, COX-2, GRO-1, iNOS, jagged-1, HMGB1 receptor, and HIF-1α are also regulated by NF-κB (Koch et al., 1995; Taylor et al., 1998; Stetler-Stevenson, 1999; Yasuda et al., 2002; Zhou et al., 2003; Rius et al., 2008; Sainson et al., 2008; van Beijnum et al., 2008). Ikkβ deficient mice died between E12.5 and E14.5 because of severe liver defects (Li et al., 1999a; Li et al., 1999b; Tanaka et al., 1999). Thus, it is not possibloe to study the role of the NF-κB pathway in endothelial cells using this model.
In order to directly address the potential roles of the NF-κB pathway in angiogenesis, we took an in vivo genetic approach using the Cre/LoxP system to specifically disrupt Ikkβ in Tie2-Cre positive endothelial cells. Conditional Ikkβ ablation in endothelial cells led to embryonic lethality between E13.5 and E15.5. The blood vessel network in the embryonic liver was specifically affected in the mice with endothelial cell deletion of Ikkβ. This led to apoptosis of hepatocytes and liver degeneration. Our results demonstrate that IKKβ is critical for fetal liver angiogenesis in vivo.
RESULTS
Ablation of Ikkβ in Tie2-positive endothelial cells leads to embryonic lethality between E13.5 and E15.5
Conditional deletion of Ikkβ using Tie2-Cre (Kisanuki et al., 2001) was used to study the function of the canonical NF-κB pathway in endothelial cells, as previous work has demonstrated that targeting this kinase leads to ablation of the canonical NF-κB pathway (Li et al., 1999a; Li et al., 1999b; Tanaka et al., 1999). Initially mice homozygous for the conditional Ikkβ floxed allele (IkkβF/F/Tie2-Cre) were studied. The majority of IkkβF/F/Tie2-Cre embryos died between E13.5 and E17.5, but infrequently mice with this genotype were born and grew to adulthood (e.g., 25 expected of genotype IkkβF/F/Tie2-Cre, but only 2 survived; supplementary data, Table 1). Endothelial cells were isolated from the aorta of the atypical mice that survived to adulthood. PCR genotyping of the aortic endothelial cells demonstrated that the null Ikkβ allele was not present (Supplementary Figure 1A). In addition, analysis of IKKβ by Western blot analysis revealed that IKKβ was expressed at near normal levels in endothelial cells from the mice that survived to adulthood (Supplementary Figure 1B).
Because these results indicated that the Tie2-Cre-mediated deletion of the Ikkβ conditional allele was incomplete, we adopted the strategy of studying mice with one conventional knockout allele and one conditional allele, Ikkβ−/F, so that only one allele needed to be deleted in endothelial cells through the action of Tie2-Cre. The strategy used to generate mice of genotype of Ikkβ−/F/Tie2-Cre is outlined in Figure 1A. Briefly, a conventional knockout allele was created by breeding IkkβF/+ mice with Sox2-Cre mice (Hayashi et al., 2002). Mice with the knockout allele were bred with Tie2-Cre mice and Ikkβ−/+/Tie2-Cre male mice were identified. The Ikkβ−/+/Tie2-Cre males were bred to IkkβF/F female mice to generate the experimental and control genotypes.
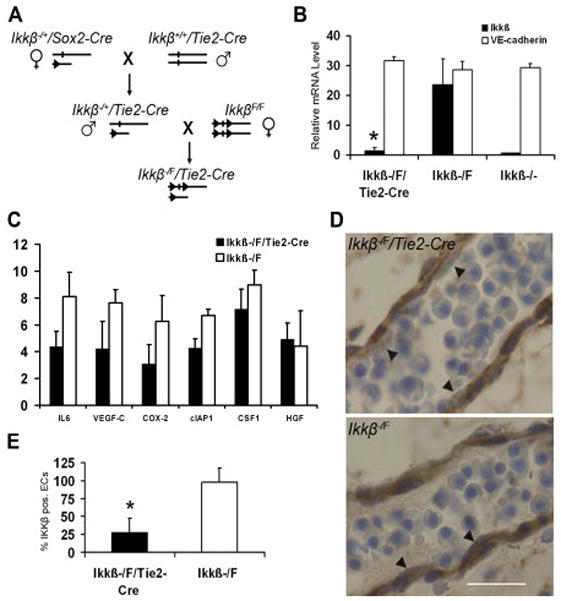
A. Generation Ikkβ−/F/Tie2-Cre mice. A conventional Ikkβ knockout allele was generated with Sox2-Cre and Ikkβ−/+/Tie2-Cre male mice were crossed with IkkβF/F female mice. B. IKKβ and VE-cadherin mRNA expression levels in PECAM-1 positive embryonic endothelial cells isolated by FACS from Ikkβ−/F/Tie2-Cre, Ikkβ−/F and Ikkβ−/− embryos. Cells from four individual embryos for each genotype were analyzed in duplicate experiments. Error bars indicate standard deviation. * indicates that the difference between Ikkβ−/F/Tie2-Cre and Ikkβ−/F embryos is significant as determined by student t-test (p<0.01). C. NF-κB target gene mRNA expression levels in PECAM-1 positive endothelial cells from Ikkβ−/F/Tie2-Cre and Ikkβ−/F embryos. Three individual embryos for each genotype were analyzed in duplicate experiments. Error bars indicate standard deviation. D. IKKβ expression in endothelial cells was examined with immunohistochemistry staining. Endothelial cells in Ikkβ−/F/Tie2-Cre embryos showed less IKKβ staining compared to Ikkβ−/F embryos (arrows). 100X, scale bar 50 μm. E. Quantification of IKKβ positive cells in mutant versus control embryonic blood vessels studied. * indicates significance (P<0.01). Total 50 ECs were counted.
Mice of Ikkβ−/F/Tie2-Cre genotype, expected at a Mendelian frequency of 25%, were not observed beginning at E15.5 (Table 1). Further analysis indicated that Ikkβ−/F/Tie2-Cre mice were present at expected frequencies at E12.5. However, embryonic lethality was observed beginning at E13.5 (Table 1). All other possible genotypes from this genetic cross were observed at expected frequencies (not shown). These results indicated that embryonic lethality occurred in the period between E13.5 and E15.5.
Table 1
Lethality of Ikkβ−/F/Tie2-Cre mice happened between E13.5 and E15.5 in FVB/N background.
Embryonic Day | Total embryos | Ikkβ−/F/Tie2-Cre embryos | Expected Ikkβ−/F/Tie2-Cre embryos |
---|---|---|---|
E11.5 | 57 | 14 | 14.25 |
E12.5 | 54 | 15 | 13.5 |
E13.5 | 63 | 5 | 15.75 |
E14.5 | 40 | 3 | 10 |
E15.5 | 76 | 0 | 19 |
Expected Menderian ratio is 25%.
Viable: heartbeat can be detected at dissection.
To confirm that IKKβ was absent in endothelial cells, PECAM/CD31 positive cells were isolated from E12.5 embryos, before embryonic lethality occurred, by high speed fluorescence activated cell sorting (FACS). The PECAM-1 positive cells constituted approximately 5% of the total cells isolated from each individual embryo (Supplementary Figure 2). Total RNA was extracted from the enriched PECAM-1-positive cells and subjected to qRT-PCR analysis. The level of Ikkβ mRNA in Ikkβ−/F/Tie2-Cre endothelial cells was reduced by 16-fold compared to controls, similar to levels observed in conventional knockout mice (Figure 1B). VE-cadherin, another endothelial cell marker, was expressed at the similar level in Ikkβ−/F/Tie2-Cre, Ikkβ−/F and Ikkβ−/− embryos (Figure 1B). Additionally, the expression level of six NF-κB target genes in the PECAM-1 positive cells was also studied by qRT-PCR (Figure 1C). The mRNA levels of three target genes, IL-6, VEGF-C and COX-2, were reduced 2-fold in Ikkβ−/F/Tie2-Cre endothelial cells compared to Ikkβ−/F control cells (Figure 1C). The relatively small effect on expression of these NF-κB target genes may reflect that basal levels and not cytokine induced levels of expression were determined. The data are consistent with a deletion of the Ikkβ gene and downregulation of the NF-κB pathway in endothelial cells isolated for Ikkβ−/F/Tie2-Cre embryos.
As a complementary approach to confirm Ikkβ deletion in endothelial cells, IKKβ expression was studied by immunohistochemistry in experimental and control mice (Figure 1D). Significantly reduced IKKβ expression was detected in the majority of endothelial cells of Ikkβ−/F/Tie2-Cre embryos compared to controls (arrows in Figure 1D; quantified in Figure 1E). This commercial antibody did not work in our hands for indirect immunofluorescence using frozen sections (data not shown).The results support the conclusion that Ikkβ was deleted in Tie2-positive endothelial cells throughout the embryo.
Angiogenesis in livers of Ikkβ−/F/Tie2-Cre embryos is impaired
Ikkβ−/F/Tie2-Cre embryos at E13.5 had normal appearance at dissection (Figure 2A). Histological analysis of sagittal sections of viable E13.5 Ikkβ−/F/Tie2-Cre embryos revealed specific abnormalities in the livers of these mutant embryos compared to controls (Figure 2B). The architecture of the liver was disrupted and there were regions of apparent cell death. This phenotype was very similar to Ikkβ−/− embryos, but cell death occurred regionally in the livers of Ikkβ−/F/Tie2-Cre embryos compared to through the entire livers in Ikkβ−/− embryos at the same stage. No obvious defects were detected in other organs or regions of the embryos (Figure 2C).
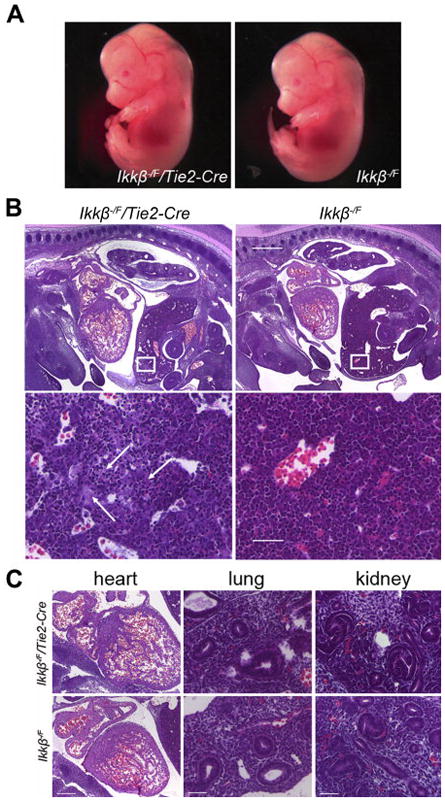
A. Ikkβ−/F/Tie2-Cre embryos are normal by their gross appearance (E13.5). B. H&E staining of sagittal sections. Ikkβ−/F/Tie2-Cre embryos show obvious liver defects. 4X, scale bar 500 μm. Boxed areas are showed in higher magnification at the bottom panel. 40X, scale bar 50 μm. Liver structure is disorganized and arrows highlight areas of cell death. C. H&E staining of other organs. Heart 10X, scale bar 200 μm. Lung and kidney 40X, scale bar 50 μm.
To determine if angiogenesis was affected in Ikkβ−/F/Tie2-Cre embryonic livers, PECAM-1 antibody was used to visualize endothelial cells by indirect immunofluorescence (Figure 3A). The density of blood vessels was reduced by 2.6-fold in Ikkβ−/F/Tie2-Cre embryonic livers (Figure 3B). This decrease in blood vessel density was seen throughout the entire embryonic liver. In contrast, angiogenesis through the remainder of the embryonic body was not affected in Ikkβ−/F/Tie2-Cre embryos, as determined by whole mount PECAM-1 immunohistochemistry staining (Figure 3C). Blood vessels are composed of endothelial cells and supporting cells, such as vascular smooth muscle cells and pericytes (Cleaver and Melton, 2003). To study the blood vessel integrity in Ikkβ−/F/Tie2-Cre embryos, PECAM-1 and smooth muscle α-actin double immunofluorescence staining was performed on frozen sections (Figure 3D). The analysis indicated that there was no obvious blood vessel disorganization in the embryonic vasculature in Ikkβ−/F/Tie2-Cre embryos.
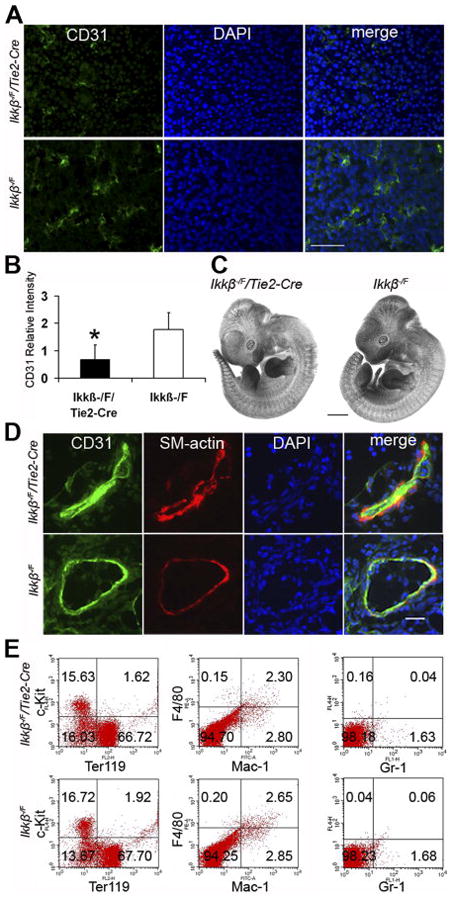
A. PECAM-1 (green) immunofluorescence staining of frozen transverse section of embryonic livers at E13.5. 40X, scale bar 50 μm. B. Relative PECAM-1 intensity was quantified with Image J software. Four individual embryonic liver sections with three fields for each sample were studied. * indicates that the difference is significant (p<0.01). C. Angiogenesis is intact in whole embryos. PECAM-1 IHC staining in whole mount. Scale bar 1 mm. Six individual embryos were studied. D. Cryosections of embryos were stained with anti-PECAM-1 antibody and anti-smooth muscle α-actin antibody. 40X, scale bar 20 μm. Data are representative examples for five individual embryos. E. FACS analysis of hematopoietic differentiation in livers for cell type markers c-Kit, Ter119, F4/80, Mac-1 and Gr-1. The percentage of cells in each quadrant is indicated. Data are representative examples for three individual embryonic livers studied.
Hematopoiesis occurs in the liver beginning at E10 (Godin and Cumano, 2005). Since liver degeneration was observed in Ikkβ−/F/Tie2-Cre embryos, and because Tie2-Cre was also active in hematopoietic stem cells (Kisanuki et al., 2001), the status of fetal liver hematopoiesis was examined at E12.5 by performing flow cytometry with different hematopoietic cell lineage markers. Populations of c-Kit+, Ter119+, F4/80+, Mac-1+ and Gr-1+ cells were intact in Ikkβ−/F/Tie2-Cre fetal livers (Figure 3E). These results indicated that hematopoiesis in mutant livers was not impaired. Immunostaining with F4/80 indicated no significant differences in the Kupffer cells populations between mutant and control embryos (data not shown).
Decreased proliferation and increased apoptosis in livers of Ikkβ−/F/Tie2-Cre embryos
Cell proliferation was measured in sagittal sections of Ikkβ−/F/Tie2-Cre embryos using a BrdU incorporation assay (Figure 4). BrdU positive cells were reduced by 5-fold at E13.5 in Ikkβ−/F/Tie2-Cre embryonic livers compared to Ikkβ−/F controls (Figure 4A top panels, quantification in 4B), indicating that the number of cells in S phase was significantly reduced at E13.5 in Ikkβ−/F/Tie2-Cre embryonic livers. In contrast, no obvious difference between mutants and controls was detected at E12.5 (Figure 4A bottom panels, panels,4C4C).

A. Proliferation was measured by BrdU incorporation. BrdU immunohistochemistry staining of embryonic livers at E13.5 and E12.5. 40X, scale bar 20 μm. B. and C. Percentage of BrdU positive cells were measured at E13.5 (B) and E12.5 (C). Three individuals were analyzed. * indicates that difference is significant measured by student t-test (p<0.01).
To further characterize the phenotype of Ikkβ−/F/Tie2-Cre embryonic livers, immunohistochemistry with cleaved Caspase-3 (Asp175) antibody was performed on both Ikkβ−/F/Tie2-Cre and Ikkβ−/F sections at E13.5 (Figure 5A) and E12.5 (Supplementary Figure 4) to analyze potential changes in apoptosis. Cells that were undergoing apoptosis increased 10-fold in Ikkβ−/F/Tie2-Cre embryonic livers at E13.5 compared to controls. This level of apoptosis was approximately 3.5-fold lower than in the conventional Ikkβ knockout mice (Figure 5B). Apoptosis in the liver at E13.5 was regional and occurred in an apparently random fashion. This was in contrast to the changes in blood vessel density which occurred throughout the fetal liver (Figure 3A). At E12.5, before embryonic lethality was first detected, Ikkβ−/F/Tie2-Cre embryonic livers showed only a 2-fold increase in apoptosis compared to controls (Supplementary Figure 4; Figure 5B). There was no increased apoptosis detected in the heart, lung and kidney (Figure 5C). Apoptosis was also verified by TUNEL staining in an independent set of embryos (data not shown).
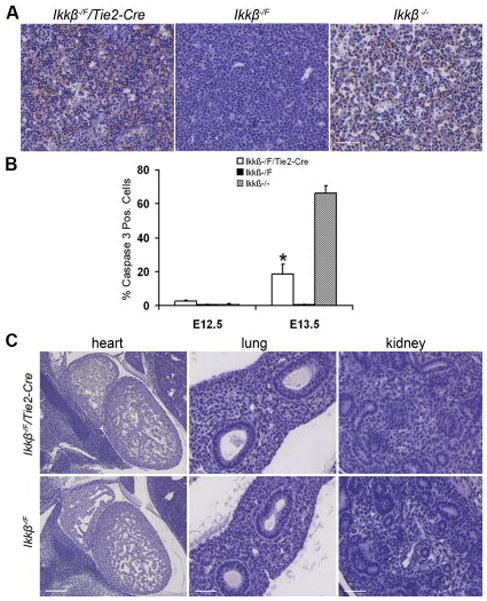
A. Regional apoptosis was detected in Ikkβ−/F/Tie2-Cre embryonic livers. Cleaved Caspase-3 (Asp175) antibody IHC staining of paraffin-embedded sections at E13.5. 40X, scale bar 50 μm. B. Ratio of apoptotic cells to total liver cells at E12.5 and E13.5. Five individual embryos for each genotype were analyzed. * indicates that the difference between Ikkβ−/F/Tie2-Cre and Ikkβ−/F embryonic livers at E13.5 is significant as determined by student t-test (p<0.01). C. Apoptosis was not increased in heart, lung and kidney at E13.5. Heart 10X, scale bar 200 μm. Lung and kidney 40X, scale bar 50 μm.
Two-color immunofluorescence with the cleaved Caspase-3 antibody and either PECAM-1 antibody staining to detect endothelial cells or alpha-fetoprotein antibody staining to detect embryonic hepatocytes, was performed to identify the cell type undergoing apoptosis. The experiments revealed that apoptosis was significantly increased in alpha-fetoprotein positive hepatocytes in mutant versus control embryos (Figure 6A). However, there was no significant increase in apoptosis in cells that were PECAM-1 positive (Figure 6B). These results indicated that hepatocytes were the major cell type undergoing apoptosis in Ikkβ−/F/Tie2-Cre embryos.
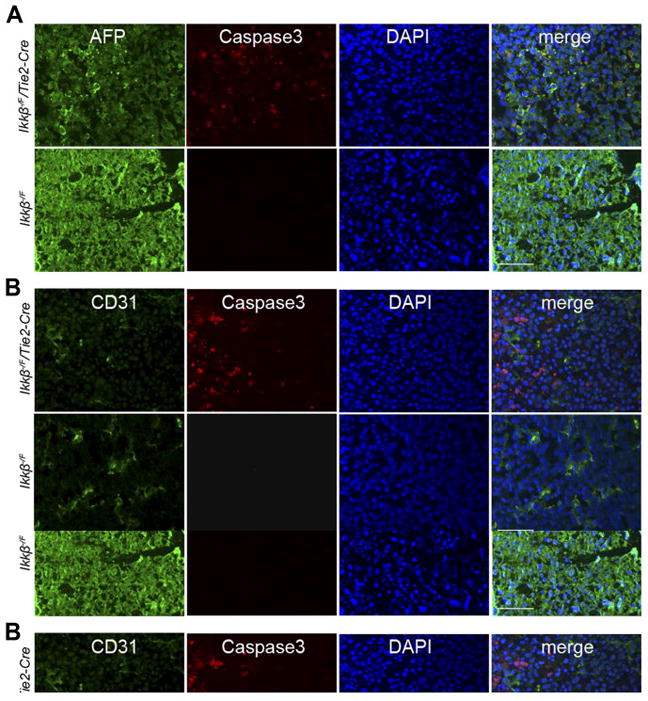
A. and B. AFP (green, A) or PECAM-1 (green, B) and cleaved Caspase-3 (red) double antibody staining of frozen sections from E13.5 embryonic livers. 40X, scale bar 50 μm. C. IKKβ immunohistochemistry staining in Ikkβ−/F/Tie2-Cre and Ikkβ−/− embryonic livers. 40X, scale bar 50 μm. D. TNF-α mRNA expression levels in Ikkβ−/F/Tie2-Cre and Ikkβ−/F whole embryonic livers.
To rule out the possibility that Tie2-Cre may have non-specifically deleted Ikkβ from hepatocytes leading to cell death, immunohistochemistry with IKKβ antibody was performed on transverse sections of Ikkβ−/F/Tie2-Cre embryonic livers. Robust IKKβ staining was detected in Ikkβ−/F/Tie2-Cre embryonic livers (Figure 6C), consistent with the expression of IKKβ in hepatocytes. In a control experiment, tissue from Ikkβ−/−embryos was also studied, demonstrating the absence of IKKβ staining in these embryos (Figure 6C). In conventional Ikkβ knockout mice, apoptosis in the liver occurs due to increased expression and sensitivity to TNF-α (Li et al., 1999a; Li et al., 1999b; Tanaka et al., 1999). However, TNF-α RNA levels were no different in Ikkβ−/F/Tie2-Cre compared to controls (Figure 6D).
DISCUSSION
Conditional deletion of Ikkβ using Tie2-Cre resulted in embryonic lethality with obvious defects in fetal liver angiogenesis and liver degeneration. The restricted phenotype was not due to liver-specific ablation of Ikkβ as deletion of the gene was pan-endothelial. An inductive role for endothelial cells in liver development beginning at E8.5 has been previously established (Matsumoto et al., 2001). However, based on the later onset of the phenotype in the Ikkβ−/F/Tie2-Cre mice, a role for the IKKβ/NF-κB pathway in early endothelial inductive events appears unlikely. The results presented here indicate that the IKKβ/NF-κB pathway plays a novel role in liver homeostasis during embryonic development. The sinusoidal endothelium of the liver is structurally and functionally distinct from the general endothelium of the embryo (Couvelard et al., 1996; Braet and Wisse, 2002) and these differences may explain the apparent lack of phenotype in other tissues and organs.
The phenotype of the Ikkβ−/F/Tie2-Cre embryos was unexpectedly similar to the phenotype observed in the conventional knockout, albeit not as severe (Li et al., 1999a; Li et al., 1999b; Tanaka et al., 1999). Hepatocyte apoptosis occurs later in the Ikkβ−/F/Tie2-Cre mice and hepatocyte apoptosis was initially more regional, limited to a few lobes at E13.5, compared to the Ikkβ deficient mice at the same or earlier stages. In contrast, hepatocyte-specific knockout of Ikkβ using the Alfp-Cre transgene did not result in significant embryonic lethality, despite the activity of this Cre-driver beginning around E10.5 (Kellendonk et al., 2000; Luedde et al., 2005). Taken together, this information suggests that ablation of Ikkβ in endothelial cells could contribute to the conventional knockout phenotype. One caveat concerning this possible interpretation is that a different targeted allele of Ikkβ, a construct that targeted exons 6–7, was utilized in the Alfp-Cre experiments (Luedde et al., 2005). It is possible this allele is hypomorphic, or that Alfp-Cre is not expressed early enough in embryonic development to duplicate the results with the conventional knockout allele. A second caveat is that in the results reported here, the one copy of Ikkβ deleted in hepatocytes may be necessary for the phenotype seen with Tie2-Cre ablation of Ikkβ in endothelial cells. However, hepatocyte apoptosis was also observed in IkkβF/F/Tie2-Cre studied in embryos at E15.5 (data not shown), indicating that the phenotype observed does not depend on deletion of one allele of Ikkβ in hepatocytes.
The decreased cell proliferation and increased cell death observed in hepatocytes in Ikkβ−/F/Tie2-Cre embryos is non-cell autonomous, dependent apparently on the deletion of the Ikkβ gene in endothelial cells. It is well appreciated that communication between the distinct cell types present in liver is required to affect organ structure and functions. For example, the endothelium has been demonstrated to protect hepatocytes independent of its evident role in supplying nutrients and oxygen (LeCouter et al., 2003). Similarly, co-cultivation of hepatocytes with endothelial cells in vitro can potentiate expression of hepatocyte-specific genes, such as albumin and ApoA-I (Harimoto et al., 2002; Kurosawa et al., 2005). Additionally, crosstalk between hepatocytes and closely juxtaposed endothelial cells mediated via vascular endothelial growth factor is essential for sinusoidal endothelial growth and differentiation (Edwards et al., 2005). Our results define the IKKβ/NF-κB pathway as a key element in this intercellular communication during embryonic development. The exact molecular nature of the intercellular dialogue, and how its disruption results in the phenotype observed in these studies, remains to be determined. One obvious candidate, TNF-α does not seem to be involved, since hepatocytes still expressed IKKβ and TNF-α RNA Levels were not altered. Which factors or cytokines are responsible for this crosstalk deserves further investigation.
EXPERIMENTAL PROCEDURES
Mice
Floxed mice (IkkβF/F) were described previously (Li et al., 2003). Ikkβ conventional knockout mice were generated by crossing IkkβF/F mice with Sox2-Cre mice (Hayashi et al., 2002). Tie2-Cre mice were described previously (Kisanuki et al., 2001). All animals were maintained on a FVB/N background (>10 generations). Animals were housed in the animal facility at The Ohio StateUniversity Biomedical Research Tower under sterile conditionsmaintaining constant temperature and humidity and were fed astandard diet. Mice were genotyped by PCR analysis from preparedtail DNA. All protocols for the use of mice were approved by the OSU Institutional Animal Use and Care Committee.
Quantitative real-time PCR
Total RNA was extracted from CD31 sorted endothelial cells by Trizol (Invitrogen, Carlsbad, CA) according to the manufacturer’s recommendations. RNA was purified using the TURBO DNA-free kit (Ambion, Austin, TX). Total RNA was reverse transcribed by Superscript III reverse transcriptase (Invitrogen, Carlsbad, CA) with random hexamer primers. qPCR was performed as previously described (Wei et al., 2004). All PCR reactions were performed in duplicate. Primer sequences are available upon request.
Histology and Immunohistochemistry
Whole-mount embryo immunohistochemistry was performed as described (Suri et al., 1996). Tissue was fixed in 10% buffered formalin (Fisher, Middletown, VA). After routine processing, paraffin-embedded sections (5 μm thick) were stained with hematoxylin and eosin (H&E) for histological analysis. Immunohistochemistry was performed on paraffin-embeddedsections (5 μm thick), and immunofluorescence studies were performed on cryo-preserved sections (7 μm thick). Paraffin sections were dewaxed and rehydrated through a graded series of ethanol washes, and maintained in phosphate buffered saline (pH 7.4; PBS). Frozen sections were air dried for 30 minutes and then placed in PBS. Sections were treated with 3% H2O2 for 10 minutes to quench endogenous peroxidase activity and placed in Dako target retrieval solution at sub-boiling temperature for 60 minutes to unmask the antigens. Protein Block (Dako, Carpinteria, CA) was applied for 30 minutes to reduce non-specific binding of the antibodies. Sections were treated with primary antibody, followed by biotin conjugated secondary antibody (Rockland, Gilbertsville, PA). Alexa Fluro dye 488 or 594 streptavidin (Molecular Probes, Eugene, OR) were used for immunofluorescence. Vectorstain ABC kit (Vector Laboratories, Burlingame, CA) was used for immunohistochemistry, followed by DAB to visualize immunocomplexes. Primary antibodies were: anti-PECAM antibody (MEC13.3, rat anti-mouse monoclonal, Pharmingen, San Diego, CA), anti-smooth muscle α-actin antibody (Spring Bioscience, Fremont, CA), anti-cleaved Caspase-3 antibody (Asp175) (9661, cell signaling, Danvers, MA), anti-Brdu antibody (M0744, DAKO, Carpinteria, CA), anti-AFP antibody (MAB1368, R&D Sytems, Minneapolis, MN) and anti-IKKβ antibody (ab55404, abcam, Cambridge, MA).
Flow cytometry
Embryos and embryonic livers were isolated at E12.5. DNA prepared from yolk sacs was used to genotype the embryos. A single cell suspension of embryonic livers or whole embryos was prepared by established method (Motoike et al., 2000) and treated with red blood cell lysing buffer (Sigma, St. Louis, MO). 1×106 cells for flow or 1 ×107 cellsfor sorting were incubated with antibodies (0.5 mg/1×106 cells) in DMEM +2% FCS on ice for 30 minutes in the dark. Cells were washed once with 1X PBS + 1% FCS. Cells fixed in 1% formalin were analyzed with the FACS Calibur (Beckton-Dickinson, San Jose, CA), or directly sorted without fixation on the FACS Aria (Beckton-Dickinson, San Jose, CA). Monoclonal antibodies against the following antigens were used: Gr-1-FITC, F4/80-PE, Mac-1-FITC, Ter119-PE, c-Kit- FITC and CD31-PE (eBioscience, San Diego, CA and Caltag, Carlsbad, CA). Appropriate isotype controls were also analyzed.
BrdU incorporation assay
100 μg BrdU (5-Bromo-2’-deoxyuridine, Sigma, St. Louis, MO) per gram of body weight in 1X PBS was injected ventrally into the abdomen of pregnant mice avoiding the embryos. Mice were sacrificed two hours after injection. Embryos were fixed in 10% buffered formalin and embedded in paraffin. BrdU incorporation was measured by anti-BrdU antibody immunohistochemistry. Three individual embryos were analyzed in each genotype group at each time point.
Supplementary Material
Supp Fig 1
Supplementary Figure 1. Ikkβ was not deleted in aortic endothelial cells from IkkβF/F/Tie2-Cre mice that survived to adults:A. Genomic DNA was prepared from IkkβF/F/Tie2-Cre and IkkβF/F aortic endothelial cells. Control DNA sample was prepared from IkkβF/F/Tie2-Cre embryo. Normal PCR was performed to analyze the genotype. No knockout band was detected in IkkβF/F/Tie2-Cre aortic endothelial cells. B. The level of IKKβ was measured by Western blot. These results are representative of three individual experiments.
Supp Fig 2
Supplementary Figure 2. Fluorescence-activated cell sorting (FACS)High speed FACS analysis of endothelial cells with PECAM-1-PE antibody from E12.5 embryos. Green curve shows the total cell population. P1 population (approximately 5% of the total cells) is the PECAM-1 positive portion that was sorted and collected.
Supp Fig 3
Supplementary Figure 3. Unaltered proliferation in other organs at E13.5 in Ikkβ−/F/Tie2-Cre embryos:BrdU incorporation in hearts, lungs and kidneys of Ikkβ−/F/Tie2-Cre embryos at E13.5 was normal compared to Ikkβ−/F embryos. 40X, scale bar 50 μm.
Supp Fig 4
Supplementary Figure 4. Apoptosis was less severe in Ikkβ−/F/Tie2-Cre livers at E12.5 than at E13.5:Cleaved Caspase-3 (Asp175) antibody IHC staining of paraffin-embedded sections at E12.5. 40X, scale bar 50 μm.
Supplementary Table 1. Lethality of IkkβF/F/Tie2-Cre mice
Acknowledgments
We thank Masashi Yanagisawa for Tie2-Cre transgenic mice. We are grateful to the staff of mouse facilities for animal husbandry, and to the Flow Cytometry Shared Resource and Histology Services of Ohio State University for technical support. We thank Denis Guttridge for critical discussions and advice. This work was supported by NIH grants R01 CA053271 and P01 CA097189 (MCO).
References
- Bonizzi G, Karin M. The two NF-kappaB activation pathways and their role in innate and adaptive immunity. Trends Immunol. 2004;25:280–288. [Abstract] [Google Scholar]
- Braet F, Wisse E. Structural and functional aspects of liver sinusoidal endothelial cell fenestrae: a review. Comp Hepatol. 2002;1:1. [Europe PMC free article] [Abstract] [Google Scholar]
- Chen F, Castranova V. Nuclear factor-kappaB, an unappreciated tumor suppressor. Cancer Res. 2007;67:11093–11098. [Abstract] [Google Scholar]
- Chilov D, Kukk E, Taira S, Jeltsch M, Kaukonen J, Palotie A, Joukov V, Alitalo K. Genomic organization of human and mouse genes for vascular endothelial growth factor C. J Biol Chem. 1997;272:25176–25183. [Abstract] [Google Scholar]
- Cleaver O, Melton DA. Endothelial signaling during development. Nat Med. 2003;9:661–668. [Abstract] [Google Scholar]
- Couvelard A, Scoazec JY, Dauge MC, Bringuier AF, Potet F, Feldmann G. Structural and functional differentiation of sinusoidal endothelial cells during liver organogenesis in humans. Blood. 1996;87:4568–4580. [Abstract] [Google Scholar]
- Edwards S, Lalor PF, Nash GB, Rainger GE, Adams DH. Lymphocyte traffic through sinusoidal endothelial cells is regulated by hepatocytes. Hepatology. 2005;41:451–459. [Abstract] [Google Scholar]
- Godin I, Cumano A. Of birds and mice: hematopoietic stem cell development. Int J Dev Biol. 2005;49:251–257. [Abstract] [Google Scholar]
- Hacker H, Karin M. Regulation and function of IKK and IKK-related kinases. Sci STKE. 2006;2006:re13. [Abstract] [Google Scholar]
- Harimoto M, Yamato M, Hirose M, Takahashi C, Isoi Y, Kikuchi A, Okano T. Novel approach for achieving double-layered cell sheets co-culture: overlaying endothelial cell sheets onto monolayer hepatocytes utilizing temperature-responsive culture dishes. J Biomed Mater Res. 2002;62:464–470. [Abstract] [Google Scholar]
- Hayashi S, Lewis P, Pevny L, McMahon AP. Efficient gene modulation in mouse epiblast using a Sox2Cre transgenic mouse strain. Mech Dev. 2002;119(Suppl 1):S97–S101. [Abstract] [Google Scholar]
- Hayden MS, Ghosh S. Shared principles in NF-kappaB signaling. Cell. 2008;132:344–362. [Abstract] [Google Scholar]
- Ishdorj G, Graham BA, Hu X, Chen J, Johnston JB, Fang X, Gibson SB. Lysophosphatidic acid (LPA) protected cancer cells from histone deacetylase(HDAC) Inhibitor-induced apoptosis through activation of HDAC. J Biol Chem 2008 [Europe PMC free article] [Abstract] [Google Scholar]
- Kellendonk C, Opherk C, Anlag K, Schutz G, Tronche F. Hepatocyte-specific expression of Cre recombinase. Genesis. 2000;26:151–153. [Abstract] [Google Scholar]
- Kisanuki YY, Hammer RE, Miyazaki J, Williams SC, Richardson JA, Yanagisawa M. Tie2-Cre transgenic mice: a new model for endothelial cell-lineage analysis in vivo. Dev Biol. 2001;230:230–242. [Abstract] [Google Scholar]
- Koch AE, Halloran MM, Haskell CJ, Shah MR, Polverini PJ. Angiogenesis mediated by soluble forms of E-selectin and vascular cell adhesion molecule-1. Nature. 1995;376:517–519. [Abstract] [Google Scholar]
- Kurosawa Y, Taniguchi A, Okano T. Novel method to examine hepatocyte-specific gene expression in a functional coculture system. Tissue Eng. 2005;11:1650–1657. [Abstract] [Google Scholar]
- LeCouter J, Moritz DR, Li B, Phillips GL, Liang XH, Gerber HP, Hillan KJ, Ferrara N. Angiogenesis-independent endothelial protection of liver: role of VEGFR-1. Science. 2003;299:890–893. [Abstract] [Google Scholar]
- Li Q, Van Antwerp D, Mercurio F, Lee KF, Verma IM. Severe liver degeneration in mice lacking the IkappaB kinase 2 gene. Science. 1999a;284:321–325. [Abstract] [Google Scholar]
- Li ZW, Chu W, Hu Y, Delhase M, Deerinck T, Ellisman M, Johnson R, Karin M. The IKKbeta subunit of IkappaB kinase (IKK) is essential for nuclear factor kappaB activation and prevention of apoptosis. J Exp Med. 1999b;189:1839–1845. [Europe PMC free article] [Abstract] [Google Scholar]
- Li ZW, Omori SA, Labuda T, Karin M, Rickert RC. IKK beta is required for peripheral B cell survival and proliferation. J Immunol. 2003;170:4630–4637. [Abstract] [Google Scholar]
- Luedde T, Assmus U, Wustefeld T, Meyer zu Vilsendorf A, Roskams T, Schmidt-Supprian M, Rajewsky K, Brenner DA, Manns MP, Pasparakis M, Trautwein C. Deletion of IKK2 in hepatocytes does not sensitize these cells to TNF-induced apoptosis but protects from ischemia/reperfusion injury. J Clin Invest. 2005;115:849–859. [Europe PMC free article] [Abstract] [Google Scholar]
- Matsumoto K, Yoshitomi H, Rossant J, Zaret KS. Liver organogenesis promoted by endothelial cells prior to vascular function. Science. 2001;294:559–563. [Abstract] [Google Scholar]
- Mojzis J, Varinska L, Mojzisova G, Kostova I, Mirossay L. Antiangiogenic effects of flavonoids and chalcones. Pharmacol Res. 2008;57:259–265. [Abstract] [Google Scholar]
- Motoike T, Loughna S, Perens E, Roman BL, Liao W, Chau TC, Richardson CD, Kawate T, Kuno J, Weinstein BM, Stainier DY, Sato TN. Universal GFP reporter for the study of vascular development. Genesis. 2000;28:75–81. [Abstract] [Google Scholar]
- Risau W. Mechanisms of angiogenesis. Nature. 1997;386:671–674. [Abstract] [Google Scholar]
- Risau W, Flamme I. Vasculogenesis. Annu Rev Cell Dev Biol. 1995;11:73–91. [Abstract] [Google Scholar]
- Rius J, Guma M, Schachtrup C, Akassoglou K, Zinkernagel AS, Nizet V, Johnson RS, Haddad GG, Karin M. NF-kappaB links innate immunity to the hypoxic response through transcriptional regulation of HIF-1alpha. Nature. 2008;453:807–811. [Europe PMC free article] [Abstract] [Google Scholar]
- Sainson RC, Johnston DA, Chu HC, Holderfield MT, Nakatsu MN, Crampton SP, Davis J, Conn E, Hughes CC. TNF primes endothelial cells for angiogenic sprouting by inducing a tip cell phenotype. Blood 2008 [Europe PMC free article] [Abstract] [Google Scholar]
- Stetler-Stevenson WG. Matrix metalloproteinases in angiogenesis: a moving target for therapeutic intervention. J Clin Invest. 1999;103:1237–1241. [Europe PMC free article] [Abstract] [Google Scholar]
- Suri C, Jones PF, Patan S, Bartunkova S, Maisonpierre PC, Davis S, Sato TN, Yancopoulos GD. Requisite role of angiopoietin-1, a ligand for the TIE2 receptor, during embryonic angiogenesis. Cell. 1996;87:1171–1180. [Abstract] [Google Scholar]
- Tanaka M, Fuentes ME, Yamaguchi K, Durnin MH, Dalrymple SA, Hardy KL, Goeddel DV. Embryonic lethality, liver degeneration, and impaired NF-kappa B activation in IKK-beta-deficient mice. Immunity. 1999;10:421–429. [Abstract] [Google Scholar]
- Tanjore H, Zeisberg EM, Gerami-Naini B, Kalluri R. Beta1 integrin expression on endothelial cells is required for angiogenesis but not for vasculogenesis. Dev Dyn. 2008;237:75–82. [Abstract] [Google Scholar]
- Taylor BS, de Vera ME, Ganster RW, Wang Q, Shapiro RA, Morris SM, Jr, Billiar TR, Geller DA. Multiple NF-kappaB enhancer elements regulate cytokine induction of the human inducible nitric oxide synthase gene. J Biol Chem. 1998;273:15148–15156. [Abstract] [Google Scholar]
- van Beijnum JR, Buurman WA, Griffioen AW. Convergence and amplification of toll-like receptor (TLR) and receptor for advanced glycation end products (RAGE) signaling pathways via high mobility group B1 (HMGB1) Angiogenesis. 2008;11:91–99. [Abstract] [Google Scholar]
- Wei G, Guo J, Doseff AI, Kusewitt DF, Man AK, Oshima RG, Ostrowski MC. Activated Ets2 is required for persistent inflammatory responses in the motheaten viable model. J Immunol. 2004;173:1374–1379. [Abstract] [Google Scholar]
- Yasuda M, Shimizu S, Ohhinata K, Naito S, Tokuyama S, Mori Y, Kiuchi Y, Yamamoto T. Differential roles of ICAM-1 and E-selectin in polymorphonuclear leukocyte-induced angiogenesis. Am J Physiol Cell Physiol. 2002;282:C917–925. [Abstract] [Google Scholar]
- Zhou A, Scoggin S, Gaynor RB, Williams NS. Identification of NF-kappa B-regulated genes induced by TNFalpha utilizing expression profiling and RNA interference. Oncogene. 2003;22:2054–2064. [Abstract] [Google Scholar]
Full text links
Read article at publisher's site: https://doi.org/10.1002/dvdy.21723
Read article for free, from open access legal sources, via Unpaywall:
https://www.onlinelibrary.wiley.com/doi/pdfdirect/10.1002/dvdy.21723
Citations & impact
Impact metrics
Citations of article over time
Article citations
Netrin‑4 promotes VE‑cadherin expression in endothelial cells through the NF‑κB signaling pathway.
Exp Ther Med, 28(3):351, 04 Jul 2024
Cited by: 0 articles | PMID: 39071904 | PMCID: PMC11273250
Therapeutic potential of adipose-derived mesenchymal stem cell exosomes in tissue-engineered bladders.
J Tissue Eng, 12:20417314211001545, 01 Jan 2021
Cited by: 9 articles | PMID: 33868627 | PMCID: PMC8020766
UBA2 activates Wnt/β-catenin signaling pathway during protection of R28 retinal precursor cells from hypoxia by extracellular vesicles derived from placental mesenchymal stem cells.
Stem Cell Res Ther, 11(1):428, 02 Oct 2020
Cited by: 8 articles | PMID: 33008487 | PMCID: PMC7532108
IKKβ targeting reduces KRAS-induced lung cancer angiogenesis in vitro and in vivo: A potential anti-angiogenic therapeutic target.
Lung Cancer, 130:169-178, 25 Feb 2019
Cited by: 5 articles | PMID: 30885340 | PMCID: PMC6873699
Roles of Caspases in Necrotic Cell Death.
Cell, 167(7):1693-1704, 01 Dec 2016
Cited by: 146 articles | PMID: 27984721 | PMCID: PMC5381727
Review Free full text in Europe PMC
Go to all (14) article citations
Data
Data behind the article
This data has been text mined from the article, or deposited into data resources.
BioStudies: supplemental material and supporting data
Similar Articles
To arrive at the top five similar articles we use a word-weighted algorithm to compare words from the Title and Abstract of each citation.
Endothelial-specific loss of IKKβ disrupts pulmonary endothelial angiogenesis and impairs postnatal lung growth.
Am J Physiol Lung Cell Mol Physiol, 325(3):L299-L313, 13 Jun 2023
Cited by: 1 article | PMID: 37310763 | PMCID: PMC10625829
Conditional deletion of IkappaB-kinase-beta accelerates helicobacter-dependent gastric apoptosis, proliferation, and preneoplasia.
Gastroenterology, 138(3):1022-34.e1-10, 04 Dec 2009
Cited by: 50 articles | PMID: 19962981 | PMCID: PMC2831162
IKappaB-kinase/nuclear factor-kappaB signaling prevents thermal injury-induced gut damage by inhibiting c-Jun NH2-terminal kinase activation.
Crit Care Med, 35(5):1332-1340, 01 May 2007
Cited by: 13 articles | PMID: 17414734
IkappaB kinase beta (IKKbeta/IKK2/IKBKB)--a key molecule in signaling to the transcription factor NF-kappaB.
Cytokine Growth Factor Rev, 19(2):157-165, 04 Mar 2008
Cited by: 136 articles | PMID: 18308615
Review
Funding
Funders who supported this work.
NCI NIH HHS (3)
Grant ID: R01 CA053271
Grant ID: R01 CA053271-19
Grant ID: P01 CA097189