Abstract
Free full text

Human Fatty Liver Disease: Old Questions and New Insights
Abstract
Nonalcoholic fatty liver disease (NAFLD) is a burgeoning health problem that affects one-third of adults and an increasing number of children in developed countries. The disease begins with the aberrant accumulation of triglyceride in the liver, which in some individuals elicits an inflammatory response that can progress to cirrhosis and liver cancer. Although NAFLD is strongly associated with obesity and insulin resistance, its pathogenesis remains poorly understood, and therapeutic options are limited. Here, we discuss recent mechanistic insights into NAFLD, focusing primarily on those that have emerged from human genetic and metabolic studies.
Early in eukaryote evolution, triglycerides (TGs) emerged as the preferred storage nutrient to buffer against fluctuations in energy demand and availability. The ubiquitous selection of TG for this role is attributable to two physicochemical properties: TGs provide greater caloric density (9 kcal/g) than do carbohydrates (4.5 kcal/g) or proteins (4 kcal/g), and TGs are insoluble in water, so they can accumulate to high levels with no adverse osmotic or colloidal effects on cells. In higher organisms, TG is stockpiled in adipocytes, and it accumulates in other cell types only under unusual circumstances. For example, migratory birds store large quantities of TGs in the liver as an energy source in preparation for prolonged seasonal flights, a propensity that has been exploited to produce the culinary delicacy fois gras. Like migratory birds, some humans who consume excess calories deposit fat in the liver. In humans, however, fatty liver is maladaptive and can have severe clinical consequences.
Nonalcoholic fatty liver disease (NAFLD) is an umbrella term used to describe a range of related disorders (Fig. 1). The earliest stage is hepatic steatosis, which is characterized by the deposition of TG as lipid droplets in the cytoplasm of hepatocytes. Steatosis is defined as a hepatic TG level exceeding the 95th percentile for lean, healthy individuals (i.e., >55 mg per g of liver) or as the presence of cytoplasmic TG droplets in more than 5%of hepatocytes (Fig. 1B) (1). Hepatic steatosis is often self-limited, but it can progress to NASH (nonalcoholic steatohepatitis). NASH is distinguished from simple steatosis by the presence of hepatocyte injury (hepatocyte ballooning and cell death), an inflammatory infiltrate, and/or collagen deposition (fibrosis) (Fig. 1B). It is not known whether steatosis always precedes NASH or whether steatosis and NASH are distinct disorders (Fig. 1A). NASH, in turn, can progress to cirrhosis: Between 10 to 29% of individuals with NASH develop cirrhosis within 10 years (2). In cirrhosis, hepatocytes are replaced by scar tissue composed primarily of type 1 collagen. The collagen is produced by specialized cells called stellate cells, which are activated by liver injury and play a key role in liver regeneration. Cirrhosis can ultimately progress to liver cancer (Fig. 1A); 4 to 27% of individuals with NASH-induced cirrhosis develop hepatocellular carcinoma (3). Hepatic TG content can be quantified by noninvasive imaging modalities, but liver biopsies are required to determine the stage of NAFLD.
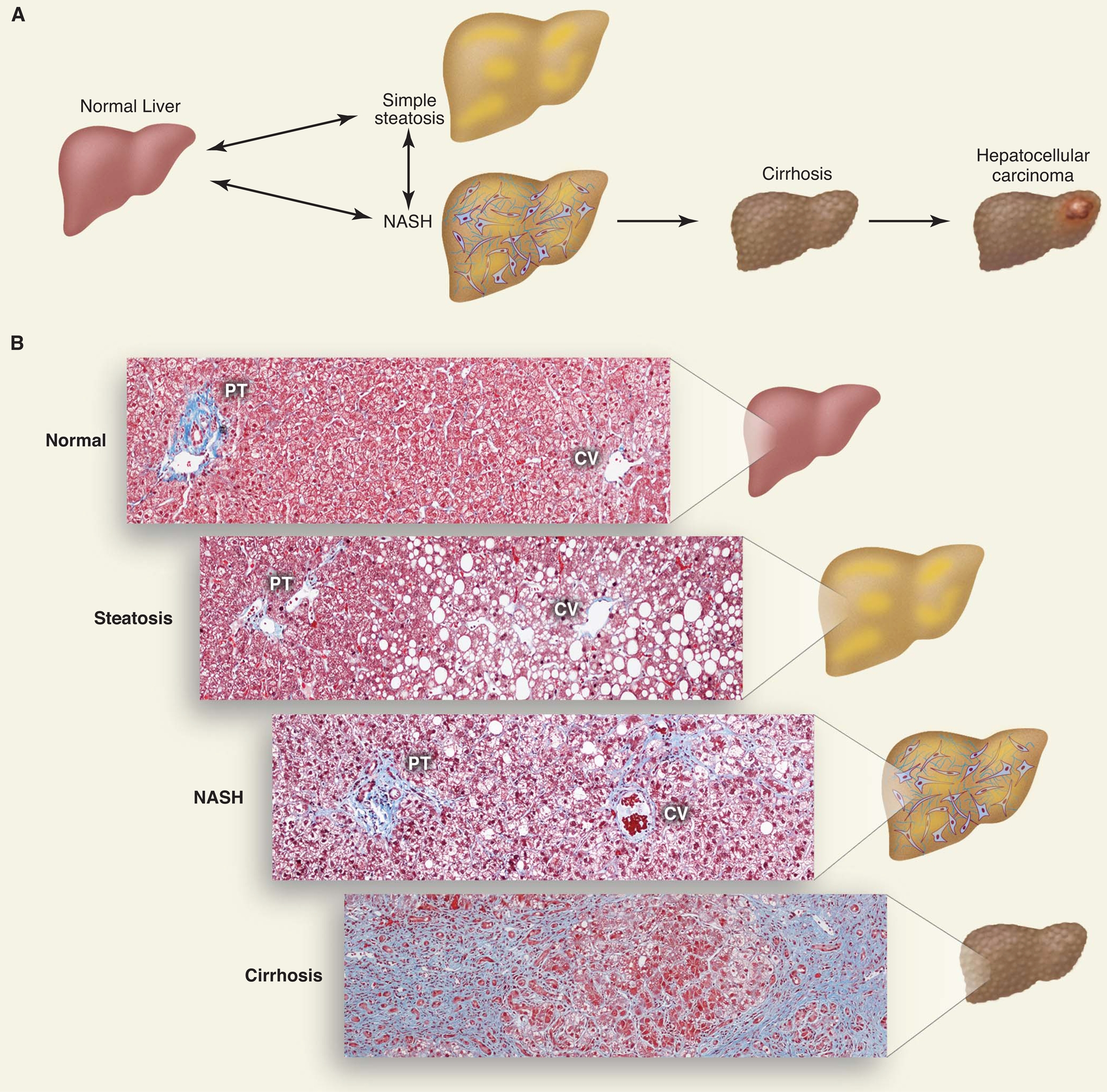
The disease spectrum of nonalcoholic fatty liver disease. (A) Schematic of progression of NAFLD. The accumulation of TG within lipid droplets in hepatocytes causes steatosis. Steatosis associated with inflammation, cell death, and fibrosis is referred to as NASH, which can progress to cirrhosis. Individuals with cirrhosis have an increased risk of hepatocellular carcinoma. (B) Histological sections illustrating normal liver, steatosis, NASH, and cirrhosis. Collagen fibers are stained blue with Masson’s trichrome stain. The portal triad (PT), which consists of the hepatic artery, portal vein, and bile duct, and the central vein (CV) are shown.
The factors that promote TG deposition in the liver and the transition from steatosis to steatohepatitis and cirrhosis in humans have not been clearly defined. Mouse models that recapitulate certain features of the human disease continuum have provided insights into possible pathological mechanisms contributing to its development (4), but the relative roles of these pathways in humans have not been conclusively determined. In this review, we focus primarily on new insights that have emerged from human genetic studies. The recent identification of sequence variations that are associated with the full spectrum of NAFLD is likely to provide new molecular clues to the pathogenesis of this increasingly common disorder (5, 6).
The Pathogenesis of Hepatic Steatosis in Humans
Hepatic steatosis arises from an imbalance between TG acquisition and removal. TGs are assembled by coupling three fatty acids to a glycerol backbone via ester bonds. As shown in Fig. 2, the fatty acids used for hepatic TG formation are derived from three sources: (i) diet, (ii) de novo synthesis, and (iii) adipose tissue. Dietary fats taken up in the intestine are packaged into TG-rich chylomicrons and delivered to the systemic circulation. In rats, ~80% of the TG in chylomicrons is hydrolyzed by lipoprotein lipase (LPL), releasing free fatty acids (FFAs) for uptake by peripheral tissues. The remaining ~20% is delivered to the liver (7). Extrapolating from these experiments, a typical American diet (100 g of fat per day) furnishes the liver with ~20 g of fat each day, equivalent to one-half of the total TG content of an average liver.
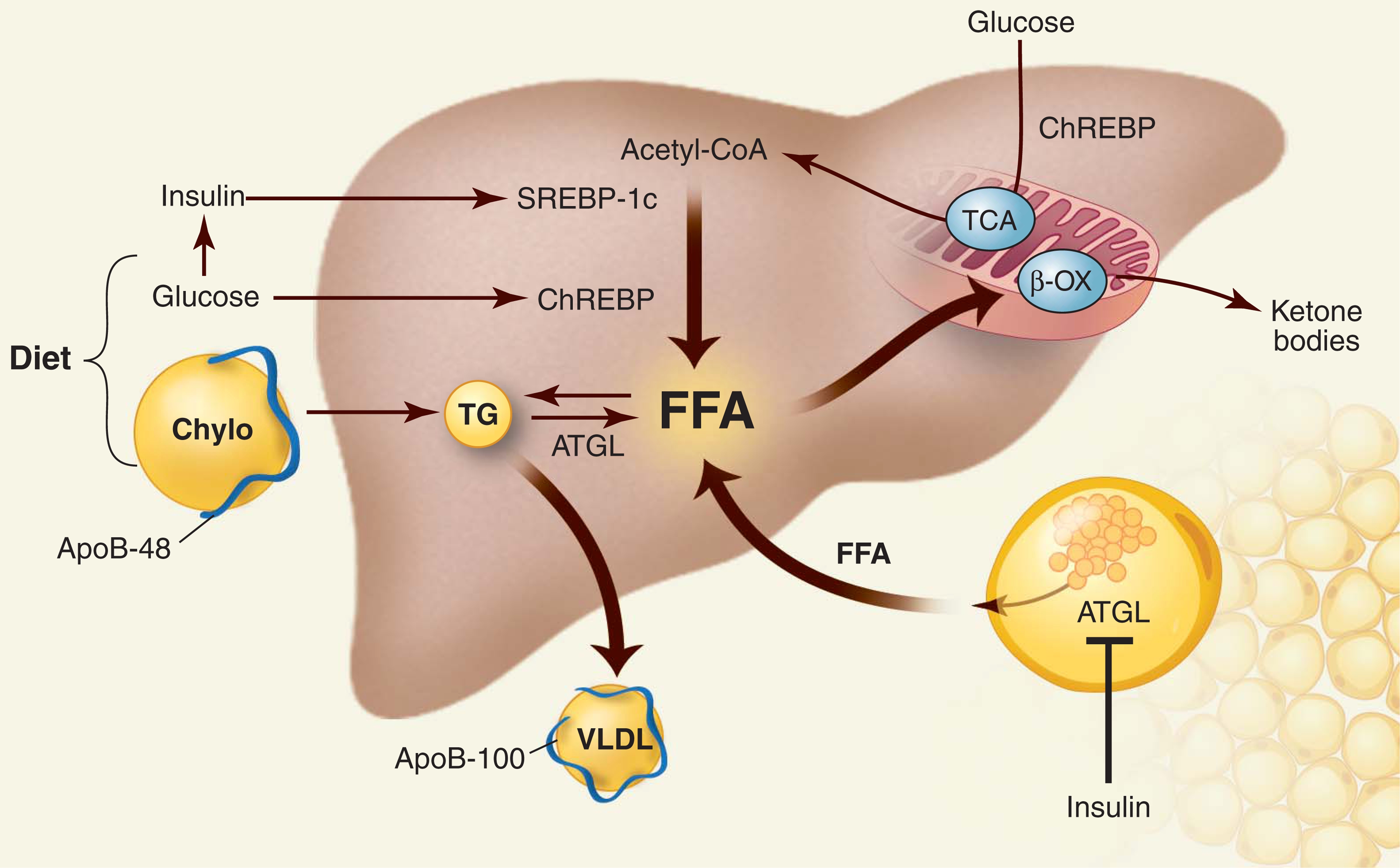
Metabolism of TG in the liver. The three major sources of FFAs are diet, endogenous synthesis, and peripheral tissues. FFAs have four possible fates. They can be metabolized by β oxidation (β-OX) in mitochondria, esterified and stored as TG in lipid droplets, used to form other lipids (not shown), or packaged with apoB into VLDL and secreted into blood. Processes that increase FFA and TG input or reduce FFA and TG output cause hepatic steatosis. Carbohydrate intake increases glucose and insulin levels, which activate two transcription factors in the liver that promote de novo lipogenesis: ChREBP and SREBP-1c. Insulin inhibits lipolysis in adipose tissue by suppressing ATGL. Chylo, chylomicron; TCA, tricarboxylic acid.
Carbohydrate feeding promotes de novo synthesis of FFA from acetyl–coenzyme A (CoA) (Fig. 2) by increasing the level of insulin and the availability of substrate. Insulin stimulates the transcription factor sterol regulatory element–binding protein–1c (SREBP-1c) via a signaling cascade involving AKT2, LXR, and mTOR (8). SREBP-1c up-regulates the enzymes that catalyze lipogenesis (9). Glucose also promotes lipogenesis by activating the transcription factor carbohydrate responsive element-binding protein (ChREBP) (10). Like SREBP-1c, ChREBP stimulates the expression of multiple genes in the fatty acid biosynthetic pathway. In addition, ChREBP increases expression of liver-type pyruvate kinase, thus providing more substrate for FFA and TG synthesis (10).
During fasting, plasma levels of insulin fall, whereas levels of glucagon and epinephrine increase, stimulating TG hydrolysis in adipocytes (Fig. 2). The first step in TG hydrolysis is catalyzed by adipocyte TG hydrolase (ATGL) (11). FFAs are released and transported to liver, mostly bound to albumin. FFAs in liver have three major fates: They can be oxidized in mitochondria to produce energy and ketone bodies, reesterified to TG and stored in lipid droplets, or coupled to apolipoproteins and secreted as a constituent of very-low-density lipoproteins (VLDL). FFAs in liver are also incorporated into phospholipids and other lipids. The flux of FFA through the circulation amounts to ~100 g/day with 20% being extracted by the liver. Thus, the daily input of TG from the diet (~20 g/day) and FFA from adipose tissue (~20 g/day) approximates the entire TG content of the liver.
Studies of humans with rare inherited disorders demonstrate that hepatic TG accretion from increased dietary intake, repartitioning of TG from adipose tissue to the liver, or increased de novo lipogenesis results in hepatic steatosis (12). Congenital generalized lipodystrophy is invariably associated with severe hepatic steatosis (12). Glycogen storage disease type 1a and citrin deficiency both lead to enhanced de novo lipogenesis and cause massive hepatic steatosis even in the absence of obesity or insulin resistance (13, 14). Citrin deficiency is caused by mutations in the mitochondrial aspartate-glutamate transporter. Inactivation of this transporter accelerates malate-citrate exchange, resulting in an increase in cytoplasmic citrate, which is converted to acetyl-CoA.
Genetic defects that prevent the removal of TG from the liver also cause steatosis. Mutations in ATGL or its cofactor, comparative gene identification–58 (CGI-58), prevent mobilization of FFA from lipid droplets (Fig. 2). Defects in the enzymes required for oxidation of FFA in mitochondria (the hydroxyacyl-CoA transferases) also cause hepatic steatosis (12). The major export pathway for hepatic TGs is secretion into the blood as VLDL (Fig. 2). Mutations in the structural protein of VLDL [apolipoprotein (apo)B] or in the protein that adds TG to the nascent lipoprotein particle in the endoplasmic reticulum (ER) (microsomal TG transfer protein, MTTP) are additional causes of hepatic steatosis. Individuals heterozygous for inactivating mutations in APOB produce fewer VLDL particles and have a three-fold increase in hepatic TG relative to healthy individuals (15).
Whereas single-gene mutations cause rare forms of severe steatosis, the increased prevalence of NAFLD in the general population over the past 30 years is due to changes in the quantity and composition of food (Fig. 2). The cardinal role of obesity in the development of hepatic steatosis is evident from cross-sectional correlations. For example, in the Dallas Heart Study, a multiethnic population-based sample that included more than 2000 individuals from Dallas, Texas, hepatic steatosis is uncommon among lean individuals [9% in individuals with body mass index (BMI) < 25 kg/m2] and highly prevalent among the severely obese (51% in individuals with BMI > 35 kg/m2) (16). Food composition also influences hepatic fat deposition. Carbohydrates in general and fructose in particular play important roles. Fructose consumption routes dietary carbons directly to the liver in a form that is primed to enter biosynthetic pathways, including de novo lipogenesis. Unlike glucose, circulating fructose is taken up almost entirely by liver (17). Because fructose is phosphorylated at carbon 1 rather than carbon 6, it cannot be used to synthesize glycogen but instead is quantitatively converted to glyceraldehyde-3-phosphate, providing substrate for de novo lipogenesis. The average yearly consumption of fructose has progressively increased and likely contributes to the increasing prevalence of NAFLD.
What Is the Relationship Between Obesity, Insulin Resistance, and Hepatic Steatosis?
The finding that genetic diseases that promote flux of energy substrates to fatty acids, such as glycogen storage disease type 1a and citrin deficiency, cause steatosis even in the absence of insulin resistance indicates that increased flux of FFA to the liver is sufficient to cause steatosis (13, 14). In obese individuals, the increased supply of FFA to the liver from the diet, from adipose tissue, and through increased de novo lipogenesis all serve to promote hepatic steatosis. The relative contributions of the three pathways to hepatic steatosis in humans have been defined in only one study: Donnelly et al. (18) reported that 59% of hepatic fat is derived from circulating FFAs, with lesser contributions from de novo lipogenesis (26%) and diet (15%).
A major unresolved question is whether NAFLD is a cause or consequence of insulin resistance. In liver, insulin inhibits glucose production and promotes fatty acid synthesis. With the development of hepatic insulin resistance, the inhibitory effect of insulin on glucose production is diminished, whereas the stimulatory effect of insulin on lipogenesis is retained (19). Insulin resistance is strongly correlated with steatosis, and interventions that ameliorate insulin resistance lead to lower insulin levels and decreased liver fat content. Multiple animal models support a direct causal relationship between insulin resistance, hyperinsulinemia, and hepatic steatosis (4). Evidence that insulin resistance causes steatosis in humans derives from patients with mutations in AKT2 (20). These patients have profound resistance to the glucoregulatory actions of insulin but presumably retain sensitivity to the lipogenic effects of the hormone. Studies of metastatic insulin-secreting tumors (insulinomas) and from pancreatic islet cell transplants provide further evidence that insulin directly promotes fat accumulation in liver cells. Hepatocytes surrounding metastatic insulinomas become engorged with TGs, as do hepatocytes surrounding transplanted islet cells (21).
The coincident occurrence of hepatic steatosis and insulin resistance has led to the hypothesis that excess TG in liver causes insulin resistance. Hepatic steatosis and insulin resistance occur together in several strains of genetically modified mice (4, 22). However, the notion that hepatic steatosis causes insulin resistance is contradicted by observations in mice with defects in diverse pathways that cause hepatic steatosis without insulin resistance. Mice with reduced fatty acid synthesis (23), mobilization (24), or oxidation (4), as well as defective cytokine signaling (25) or choline synthesis (26), maintain normal or improved insulin sensitivity despite hepatic TG accumulation. TG may be a marker for another molecule that interferes with insulin action, such as diacylglycerol (DAG), long-chain acyl-CoA, or ceramide. However, hepatic accumulation of any of these lipids does not invariably produce insulin resistance, at least in mice [for review, see (22)]. It remains possible that these lipids contribute to insulin resistance only if they accumulate within specific subcellular compartments or if they have a particular complement of fatty acids.
In humans, naturally occurring mutations provide a powerful tool to untangle mechanistic relationships between highly correlated metabolic traits. If increased hepatic TG content causes insulin resistance, then individuals with genetic variants that promote hepatic steatosis should be at increased risk of developing insulin resistance. An increasing number of Mendelian genetic defects have uncoupled these two variables. Individuals with inactivating mutations in APOB have increased levels of hepatic TG yet maintain normal insulin sensitivity (15). Patients with autosomal recessive disorders caused by mutations in either ATGL or CGI58 have severe steatosis but are not insulin resistant [reviewed in (12)]. In population-based studies, a genetic variant in PNPLA3 that is associated with hepatic steatosis is not associated with insulin resistance (see below) (5). Sequence variants in APOC3 have been associated with both hepatic steatosis and insulin resistance (25), but this association was not observed in other independent populations (26, 27). Thus, the preponderance of evidence is not compatible with the hypothesis that TG accumulation in hepatocytes causes insulin resistance in humans.
Genetic Risk Factors for Hepatic Steatosis
Although obesity and insulin resistance are the most prevalent risk factors for NAFLD, hepatic fat content varies substantially among individuals with equivalent adiposity, indicating that other factors contribute to this condition. One of these factors is gender. Before age 60, men are significantly more likely to develop steatosis than are women (16), but at older ages the disorder is more prevalent in women. Reasons for this gender dimorphism are not known. Another factor is ethnicity. In the Dallas Heart Study, hepatic steatosis was found in 45% of Hispanics, 33% of individuals of European ancestry, and 24% of African Americans (16). The higher prevalence of hepatic steatosis in Hispanics is due in part to a higher prevalence of obesity and insulin resistance in this population, but the lower prevalence in African Americans cannot be explained by ethnic differences in BMI, insulin resistance, ethanol ingestion, or medication use. Another ethnic group with an increased prevalence of hepatic steatosis is Asian Indians. A study of 482 lean young individuals revealed a twofold higher hepatic TG content in Asian Indians than men of European descent (28).
Hepatic steatosis, NASH, and cirrhosis cluster in families (29), with the heritability of NAFLD being estimated to be ~39% (30). One genetic variant that is consistently associated with NAFLD is a missense mutation [Ile148→Met148 (I148M)] in patatin-like phospholipase domain–containing (PNPLA) 3 gene PNPLA3 (also called adiponutrin) (5). This variant was initially identified through an association study of 9299 nonsynonymous sequence variations, and the relationship with hepatic TG content has been confirmed in many independent studies [for review, see (31)]. The frequency of the susceptibility variant (PNPLA3-148M) in ethnic groups mirrors the prevalence of NAFLD and accounts for ~70% of the differences in frequency of hepatic steatosis between Hispanics, African Americans, and individuals of European descent (5). Homozygotes for the risk allele in PNPLA3 (MM) have a ~twofold higher hepatic TG content, although the magnitude of the effect is strongly influenced by adiposity and insulin sensitivity.
PNPLA3 is a member of the PNPLA family, most closely resembling ATGL (PNPLA2) (Fig. 2) (11). PNPLA3 is most highly expressed in adipose tissue and liver and is transcriptionally regulated by insulin through a signaling cascade that includes LXR and SREBP-1c; hepatic PNPLA3 mRNA levels are reduced to nearly undetectable levels during fasting and increase 80-fold with refeeding in mice (32). Over 90% of the PNPLA3 in hepatocytes is located in lipid droplets, which are specialized organelles that participate in protein partitioning, trafficking, and degradation [for review, see (33)].
The physiological role of PNPLA3 and the mechanism by which the I148M isoform causes fatty liver are not known. The purified protein has both TG hydrolase and transacylase activity (34, 35). The I148M substitution markedly reduces TG hydrolase activity in vitro (35), suggesting that the I148M substitution causes a loss of function. However, inactivation of Pnpla3 in mice fails to increase hepatic TG content (36, 37), and adenoviral-mediated overexpression of PNPLA3-I148M in mouse liver causes an increase in hepatic TG content (35), which is more consistent with the I148M substitution conferring a gain of function. Additional studies will be required to determine the molecular mechanism by which variation in PNPLA3 confers susceptibility to NAFLD.
A recent genome-wide association study of hepatic steatosis in 7176 participants (6) revealed additional susceptibility loci for NAFLD. Surprisingly, none of the newly identified genomic intervals contained genes associated with rare Mendelian disorders of hepatic steatosis, such as APOB, ATGL, CGI-58, or genes associated with lipodystrophy. The allele of greatest effect size was PNPLA3-I148M, which conferred an odds ratio for NAFLD of 3.26. The other genomic regions associated with hepatic steatosis in this study included NCAN and PPP1R3B (Table 1). Analysis of an independent cohort with histologically defined NAFLD by the same group found an association with NCAN, GCKR, and LYPLAL1 but not with PPP1R3B, with odds ratios ranging from 1.37 (LYPLAL1) to1.65 (NCAN). Loss-of-function alleles in GCKR and PPP1R3B would be predicted to increase levels of glucose-6-phosphate and thus promote fatty acid synthesis. The mechanistic link between NAFLD and the other two implicated genes, NCAN and LYPLAL1, remains to be defined. Elucidation of the roles of these genes may provide new insights into the metabolic pathways that contribute to common forms of NAFLD in the population.
Table 1
Common variants associated with nonalcholic fatty liver disease. Odds ratios for NAFLD were calculated by using cases with biopsy-proven NAFLD and in ancestry-matched controls (6). EA, European American; AA, African American; ND, not determined.
Gene (first report) | Protein | Function | Allele variant | Minor allele frequency | Steatosis | NASH | Odds ratio NASH | Associations with other liver disorders |
---|---|---|---|---|---|---|---|---|
PNPLA3 (5) | Patatin-like phospholipase domain–containing protein 3 | TG hydrolase? | rs738409 I148M | 0.23 in EA 0.49 in Hispanics 0.17 in AA | Yes | Yes | 3.26 | Alcohol-related cirrhosis Hepatocellular carcinoma |
PPP1R3B (6) | Glycogen binding subunit of protein phosphatase 1 | Enhances protein phosphatase 1 activation of glycogen synthase | rs4240624 | 0.08 | Yes | No | 0.93 | ND |
NCAN (6) | Neurocan | Unknown | rs2228603 P92S | 0.07 | Yes | Yes | 1.65 | ND |
GCKR (6) | Glucokinase regulatory protein | Negative regulator of glucokinase | rs780094 P446L | 0.39 | No | Yes (not replicated) | 1.45 | ND |
LYPLAL1 (6) | Lysophospholipase-like 1 | Unknown | rs12137855 | 0.21 | No | Yes (not replicated) | 1.37 | ND |
APOC3 (25) | Apolipoprotein C3 | Limits hydrolysis of TG in circulating lipoproteins | rs2854116 rs2854117 | 0.38 in EA 0.38 in Hispanics 0.71 in AA 0.26 in EA 0.32 in Hispanics 0.66 in AA | Yes* | ND | ND | ND |
What Factors Contribute to NAFLD Progression?
The PNPLA3 allele associated with steatosis is also associated with elevated serum levels of alanine transaminase (ALT) (5, 38), an enzyme released from injured hepatocytes, and with pathological features of NASH in biopsy samples (39). PNPLA3-I148M may also contribute more generally in the response of the liver to injury. In support of this notion, PNPLA3-148M greatly increases the odds of developing cirrhosis in alcoholics (40). The finding that PNPLA3 is associated with hepatic steatosis, NASH, and cirrhosis provides strong molecular evidence that NAFLD is indeed a disease continuum as shown in Fig. 1.
About one-third of individuals with NAFLD who undergo liver biopsy have evidence of NASH. Of these individuals, 20 to 30% progress to severe fibrosis within 10 years (41). The factors causing progression of NAFLD to fibrosis and cirrhosis have not been defined in humans, in part because of the relative inaccessibility of liver tissue. Unfortunately, no animal model recapitulates the entire disease spectrum of NAFLD in humans (4). Nevertheless, studies in animal models have revealed several molecular processes that recapitulate the cardinal features of NASH: hepatocyte damage, inflammation, and fibrosis. Inflammation is a component of the wound healing process that leads to the deposition of extracellular matrix and fibrosis in liver. A growing body of evidence supports a central role for pro-inflammatory cytokines, particularly tumor necrosis factor α (TNF-α), and interleukin 6 (IL-6) (42) in the development of NASH. Levels of TNF-α and IL-6 are elevated in the liver and blood of patients with NASH, and inhibition of these cytokines has improved NAFLD in rodents (43). A second potential mechanism is ER stress, which results from improperly folded proteins accumulating in the ER, which elicits the unfolded protein response (UPR). The UPR activates nuclear factor κB, c-Jun N-terminal kinase, and oxidative stress pathways, all of which have been implicated in progression of steatosis to NASH (44).
Extrahepatic factors may also contribute to NAFLD development and progression. Up to 70% of individuals undergoing liver transplantation for NASH or cryptogenic cirrhosis develop NAFLD in the transplanted liver, although the number of studies is small and factors such as immunosuppressive drugs may play a role (45). Evidence from mice suggest that increased exposure of hepatocyctes to saturated fatty acids can trigger inflammation by interacting with Toll-like receptors and apoptosis by activating death receptors (46). Saturated fats can also inhibit mitochondrial function and induce the ER stress pathway in model organisms [see (46) for review].
Longitudinal studies of NAFLD show that a substantial number of individuals (up to 20 to 30%) improve between biopsies, suggesting that the disease might improve without treatment in some individuals (41). Striking reductions in hepatic TG content have been seen after bariatric surgery. A recent meta-analysis of 15 studies that included 766 paired liver biopsies revealed that bariatric surgery improved steatosis in 92% of patients, improved steatohepatitis in 81%, and led to complete resolution in 70% (47). Diet-induced weight loss with increased physical activity has also been shown to be associated with improvement in liver pathology (48). Recent studies using an antioxidant (vitamin E) and an insulin sensitizer, pioglitazone, have shown some success (49).
Outlook
NAFLD is a major health problem that has accompanied the trend toward an unhealthy diet. With recent advances in the prevention and treatment of hepatitis C, NAFLD is poised to become the primary indication for liver transplantation. Of greatest concern is the increased prevalence of hepatic steatosis in children, where the disease may be more virulent (50). Despite the increasing morbidity associated with NAFLD, many questions remain regarding the pathogenesis, natural history, and treatment of this disorder. For example, why is the frequency of NAFLD lower in individuals of African descent even though the prevalence of obesity and insulin resistance is high in this group? Elucidating the molecular basis for these ethnic differences may provide new therapeutic targets for the treatment of NAFLD and other components of the metabolic syndrome.
A critical question is whether hepatic TG accumulation alone is sufficient to cause disease progression in NAFLD. Is the increase in NASH and cirrhosis associated with the PNPLA3 variant conferred solely by increasing liver TG content, or does the variant have other adverse effects? In the Dallas Heart Study, the effect of PNPLA3 on liver enzyme levels is abolished after controlling for liver TG content. Some patients with primary accumulation of hepatic TG, such as patients with homozygous familial hypobetalipoproteinemia or with neutral lipid storage disease, develop cirrhosis (12). However, these disorders are uncommon, and only a minority of patients progress to cirrhosis. Thus, it is not clear whether the liver disease in these individuals is due solely to the accumulation of TG in hepatoctyes.
Improved methods for early detection of NASH are urgently needed. Hepatic steatosis can be diagnosed noninvasively, but determining the presence of hepatic inflammation and fibrosis requires a liver biopsy, which is usually reserved for individuals with elevated levels of circulating liver enzymes. Although having an elevated ALT increases the likelihood of having NASH, up to 59% of individuals with hepatic steatosis and normal ALTs have NASH on biopsy (51). Some patients with NAFLD present for the first time with cirrhosis. Development of noninvasive methods that detect inflammation and fibrosis in the liver are needed to more fully capture the natural history of this disorder and to test therapeutic approaches designed to halt or reverse disease progression. A further impediment toward the development of new therapeutic interventions is the lack of an animal model that fully recapitulates all the features of NAFLD (4). Until such a model becomes available, human genetic studies provide a good opportunity for delineating the molecular pathways that lead to steatosis, steatohepatitis, and cirrhosis.
Acknowledgments
We thank T. Rodgers for the histological sections in Fig. 1 and D. Russell, J. Goldstein, M. Brown, and J. Browning for helpful discussions. The University of Texas Southwestern Medical Center has applied to patent the association between rs738409 and NAFLD. Supported by grants from the NIH (RL1HL092550, UL1DE109584, and PO1 HL20948). H.H.H. is on the Scientific Advisory Board for Pfizer. J.D.H. is a consultant for Merck, Pfizer, and Sanofi-Aventis and is on the Scientific Advisory Board of Aegerion.
References and Notes
Full text links
Read article at publisher's site: https://doi.org/10.1126/science.1204265
Read article for free, from open access legal sources, via Unpaywall:
https://europepmc.org/articles/pmc3229276?pdf=render
Citations & impact
Impact metrics
Citations of article over time
Alternative metrics

Discover the attention surrounding your research
https://www.altmetric.com/details/102084309
Smart citations by scite.ai
Explore citation contexts and check if this article has been
supported or disputed.
https://scite.ai/reports/10.1126/science.1204265
Article citations
Cotinine exposure enhances the association of blood manganese and non-alcoholic fatty liver disease in American children: a cross-sectional study.
Sci Rep, 14(1):24593, 19 Oct 2024
Cited by: 0 articles | PMID: 39426991 | PMCID: PMC11490505
Strategy for treating MAFLD: Electroacupuncture alleviates hepatic steatosis and fibrosis by enhancing AMPK mediated glycolipid metabolism and autophagy in T2DM rats.
Diabetol Metab Syndr, 16(1):218, 11 Sep 2024
Cited by: 0 articles | PMID: 39261952 | PMCID: PMC11389443
Causal relationships between neuropsychiatric disorders and nonalcoholic fatty liver disease: A bidirectional Mendelian randomization study.
BMC Gastroenterol, 24(1):299, 04 Sep 2024
Cited by: 0 articles | PMID: 39227758 | PMCID: PMC11373482
Advanced Liver-on-a-Chip Model for Evaluating Drug Metabolism and Hepatotoxicity.
Biosensors (Basel), 14(9):435, 06 Sep 2024
Cited by: 0 articles | PMID: 39329810 | PMCID: PMC11430604
8-Prenylgenistein Isoflavone in Cheonggukjang Acts as a Novel AMPK Activator Attenuating Hepatic Steatosis by Enhancing the SIRT1-Mediated Pathway.
Int J Mol Sci, 25(17):9730, 08 Sep 2024
Cited by: 0 articles | PMID: 39273677 | PMCID: PMC11395689
Go to all (1,253) article citations
Data
Data behind the article
This data has been text mined from the article, or deposited into data resources.
BioStudies: supplemental material and supporting data
SNPs (Showing 7 of 7)
- (1 citation) dbSNP - rs2228603
- (1 citation) dbSNP - rs780094
- (1 citation) dbSNP - rs2854117
- (1 citation) dbSNP - rs2854116
- (1 citation) dbSNP - rs12137855
- (1 citation) dbSNP - rs738409
- (1 citation) dbSNP - rs4240624
Show less
Similar Articles
To arrive at the top five similar articles we use a word-weighted algorithm to compare words from the Title and Abstract of each citation.
The American lifestyle-induced obesity syndrome diet in male and female rodents recapitulates the clinical and transcriptomic features of nonalcoholic fatty liver disease and nonalcoholic steatohepatitis.
Am J Physiol Gastrointest Liver Physiol, 319(3):G345-G360, 05 Aug 2020
Cited by: 12 articles | PMID: 32755310 | PMCID: PMC7509261
Genetic Factors in the Pathogenesis of Nonalcoholic Fatty Liver and Steatohepatitis.
Biomed Res Int, 2015:460190, 27 Jul 2015
Cited by: 72 articles | PMID: 26273621 | PMCID: PMC4530215
Review Free full text in Europe PMC
Fructose as a key player in the development of fatty liver disease.
World J Gastroenterol, 19(8):1166-1172, 01 Feb 2013
Cited by: 112 articles | PMID: 23482247 | PMCID: PMC3587472
Review Free full text in Europe PMC
Nrf2 deletion causes "benign" simple steatosis to develop into nonalcoholic steatohepatitis in mice fed a high-fat diet.
Lipids Health Dis, 12:165, 04 Nov 2013
Cited by: 36 articles | PMID: 24188280 | PMCID: PMC3826845
Funding
Funders who supported this work.
NHLBI NIH HHS (6)
Grant ID: P01 HL020948-35
Grant ID: RL1 HL092550-05
Grant ID: RL1HL092550
Grant ID: P01 HL020948
Grant ID: RL1 HL092550
Grant ID: P01 HL20948
NIDCR NIH HHS (3)
Grant ID: UL1 DE019584-05
Grant ID: UL1DE109584
Grant ID: UL1 DE019584
NIDDK NIH HHS (1)
Grant ID: R01 DK090066