Abstract
Free full text

Rho-associated kinases in tumorigenesis: re-considering ROCK inhibition for cancer therapy
Abstract
The Rho-associated (ROCK) serine/threonine kinases have emerged as central regulators of the actomyosin cytoskeleton, their main purpose being to promote contractile force generation. Aided by the discovery of effective inhibitors such as Y27632, their roles in cancer have been extensively explored with particular attention focused on motility, invasion and metastasis. Recent studies have revealed a surprisingly diverse range of functions of ROCK. These insights could change the way ROCK inhibitors might be used in cancer therapy to include the targeting of stromal rather than tumour cells, the concomitant blocking of ROCK and proteasome activity in K-Ras-driven lung cancers and the combination of ROCK with tyrosine kinase inhibitors for treating haematological malignancies such as chronic myeloid leukaemia. Despite initial optimism for therapeutic efficacy of ROCK inhibition for cancer treatment, no compounds have progressed into standard therapy so far. However, by carefully defining the key cancer types and expanding the appreciation of ROCK's role in cancer beyond being a cell-autonomous promoter of tumour cell invasion and metastasis, the early promise of ROCK inhibitors for cancer therapy might still be realized.
See the Glossary for abbreviations used in this article.
Introduction
The Rho GTPase family is best known for its well-characterized roles in regulation of actin cytoskeleton organization and dynamics [1]. The actions of Rho proteins are mediated by effector proteins, which might have intrinsic catalytic activity, act as scaffolds for protein complexes and in some instances serve both functions [2]. The RhoA and RhoC family members act primarily to promote actomyosin contractile force generation through ROCK1- and ROCK2-mediated phosphorylation of numerous downstream target proteins, including LIM kinases 1 and 2 (LIMK1 and LIMK2), the myosin regulatory light chain (MLC), and the myosin binding subunit (MYPT1) of the MLC phosphatase to inhibit catalytic activity and consequent MLC dephosphorylation (Fig 1; [3,4]). Collectively, these events promote actin filament stabilization through LIMK-mediated phosphorylation and inactivation of cofilin family proteins, and through MLC phosphorylation leading to increased actin filament bundling and myosin-driven contraction. The increase in actomyosin contractility contributes directly to several proximal processes, such as regulation of morphology, motility, and cell–cell and cell–matrix adhesion. In addition, ROCK kinases influence more distal cellular processes including gene transcription, proliferation, differentiation, apoptosis and oncogenic transformation, although in many instances the molecular mechanisms have not been fully characterized. Given the wide spectrum of biological processes influenced by ROCK, it is not surprising that they have been implicated in numerous aspects of cancer. Reinforcing this association, studies in the 1990s with the selective small molecule ROCK inhibitor Y27632 [5] showed that ROCK inhibition blocked the ability of a hepatoma cell line implanted intraperitoneally into rats to form tumour nodules and to spread within the peritoneal compartment [6]. In the same year it was reported that ROCK inhibition blocked oncogenic transformation of mouse fibroblasts by RhoA, H-Ras and activated forms of the Rho guanine nucleotide exchange factors (RhoGEFs) Dbl and mNet1, whilst ROCK1 cooperated with a mildly activated version of c-Raf to promote transformation [7]. More recently, we showed that somatic ROCK1 mutations identified in human cancers have significantly increased catalytic activity [8], and subsequent high-throughput sequencing efforts have identified further ROCK1 and ROCK2 cancer-associated somatic mutations that are probably activating [9,10]. Since these initial studies, there has been considerable interest in ROCK as a potential therapeutic target for cancer. Other publications have evaluated the possible therapeutic uses for ROCK inhibitors in conditions such as hypertension and glaucoma [11,12,13]. In this review, we highlight some of the findings that have extended our understanding of the role of ROCK signalling in cancer into unexpected and potentially clinically important areas.
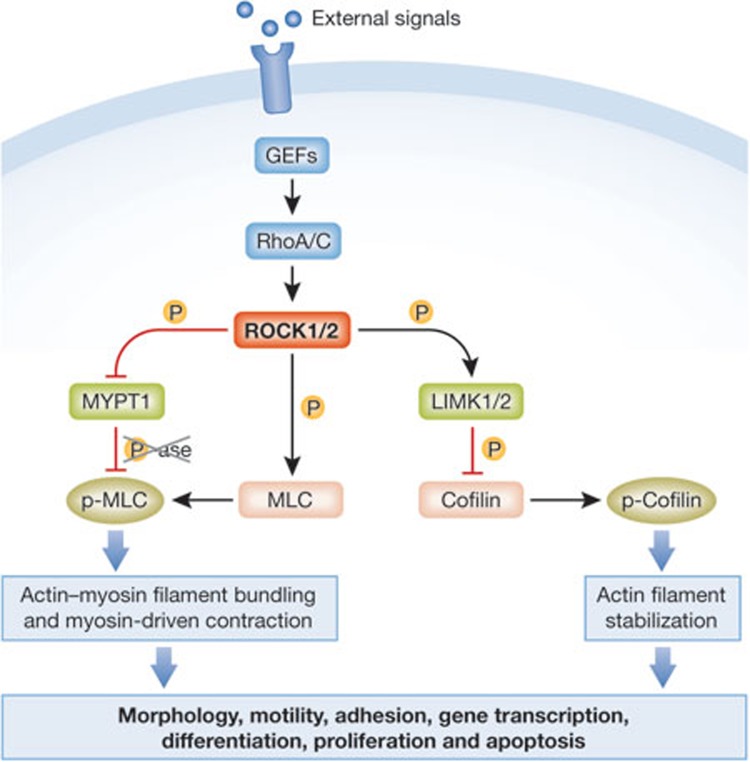
Rho/ROCK signalling promotes actomyosin contractility. RhoA/C are activated by guanine exchange factors (GEFs) that translate external signals from activated membrane receptors into cellular responses. Activated Rho proteins bind to and activate ROCK1 and ROCK2, which phosphorylate target proteins such as MYPT1, MLC and LIMK1/2 . ROCK-mediated phosphorylation of MLC and LIMK-mediated phosphorylation of cofilin promotes increased actin fibre bundling and actomyosin contraction, which directly or indirectly influence various cellular behaviours. LIMK1/2, LIN-11, Isl1 and MEC-3 domain kinase 1/2; MLC, myosin light chain; MYPT1, myosin binding subunit of the MLC phosphatase; ROCK1/2, Rho-associated coiled-coil protein kinase 1/2.
ROCK features and regulation
The two serine and threonine kinases ROCK1 (also called ROCK I or ROKβ) and ROCK2 (also known as ROCK II, Rho kinase and ROKα) were originally isolated due to their interaction with active RhoA-GTP [3,4]. Although there are two ROCK kinases in higher amniotic species, the progenitor form is probably ROCK2 given that its homologues are found throughout the bilaterial subregnum—for example DRok in Drosophila melanogaster and LET-502 in Caenorhabditis elegans. Interestingly, genetic evidence from these organisms indicates that the actomyosin-regulating function is conserved from ancestral versions to higher vertebrates. Although many studies that have identified ROCK substrates make use of ROCK2, the same proteins are usually also ROCK1 substrates. In humans, the two kinases have 65% overall identity, with 87% identity in the catalytic kinase domain, which probably accounts for the substrate promiscuity (Fig 2). Both kinases contain a coiled-coil region (56% identity), the structure of which has been solved for ROCK1 [14], and a split PH domain that is bisected by a cysteine-rich C1 conserved region (73% identity). Despite the linear arrangement of the PH-C1-PH region, structural determination by NMR has revealed that the two split PH segments of ROCK2 come together to form a conventional PH unit, attached to a separate and adjacent C1 domain [15]. Although the C1 domain was found to be atypical in that it did not bind to diacylglycerol, the distribution of charged amino acids on both C1 and PH domains were proposed to form an anchoring surface that promotes association of ROCK2—and possibly ROCK1 given the extensive conservation—with the plasma membrane [15]. Although only a single Rho-binding domain (RBD) within the coiled-coil region was originally identified [16], subsequent analysis revealed multiple contact points with Rho GTPases within the coiled-coil region of ROCK1 that probably contribute to regulation and localization [17]. RhoA, RhoB and RhoC associate with and activate ROCK most probably through the induction of conformational changes that displace the autoinhibitory carboxyl terminus. Crystal structural analysis showed that binding to the RBD of ROCK1 occurs through interactions with the Switch I and II regions of RhoA that undergo GTP-dependent conformational changes [18]. Other GTP-binding proteins are inhibitory as shown for RhoE [19], Rad and Gem [20], which bind to sites distinct from the canonical RBD. In the case of RhoE, structural and mutational analysis revealed that RhoE antagonizes the formation of actin stress fibres independently of ROCK1 binding, indicating that it affects actin cytoskeletal structures through additional protein interactions [21]. During apoptosis, proteolytic cleavage by caspases (ROCK1; [22,23]) or granzyme B (ROCK2; [24]) removes a C-terminal portion that normally represses activity, resulting in the generation of constitutively active kinases. Although many kinases are themselves regulated through phosphorylation by other kinases, association with PDK1 promotes ROCK1 activity by blocking association with inhibitory RhoE [25]. Phosphorylation at multiple specific sites by polo-like kinase 1 was found to promote the ability of RhoA to activate ROCK2 [26]. In contrast, Src-mediated ROCK2 phosphorylation on Tyr 722 reduces Rho-GTP binding, leading to a decreased response to upstream activation [27]. Given that additional uncharacterized phosphorylation sites in ROCK1 and ROCK2 have been detected (www.phosphosite.org), other serine, threonine and tyrosine kinases might also influence ROCK activity. However, X-ray crystallographic studies revealed that the kinase activation loop adopts an active conformation in the absence of phosphorylation, which differentiates the ROCK1 [28] and closely related MRCKs [29] from other members of the AGC kinase family [30]. It was shown that ROCK2 undergoes autophosphorylation upon activation at several C-terminal sites that do not influence catalytic activity [31]. At least one of these phosphorylation events (Ser 1366) directly reflects ROCK2 activation status, allowing for the development of antibody reagents that gave a read-out of ROCK2 kinase activity in cell lines and breast cancer tumour samples [31]. Further characterization of ROCK1 and ROCK2 autophosphorylation might enable development of additional antibody tools to measure ROCK activity across tumour types, which will be invaluable in assessing the association of these kinases with tumour growth and progression. In addition, such activation-state-dependent antibody reagents could be used as pharmacodynamic probes to assess whether ROCK inhibitors were hitting their target should clinical trials be initiated.
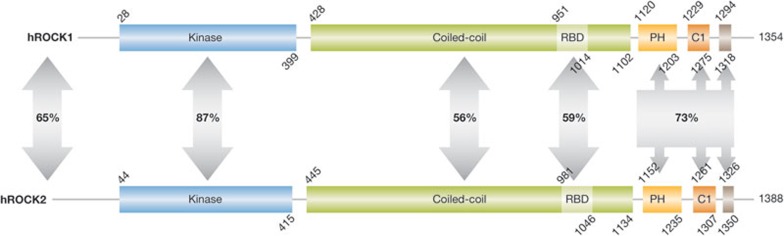
ROCK functional domains. Conserved domains in human ROCK1 and ROCK2. The percentage identities between regions were determined by pairwise BLAST comparisons. C1, protein kinase C conserved region 1; PH, pleckstrin homology domain; RBD, Rho-binding domain; hROCK1/2, human Rho-associated coiled-coil protein kinase 1/2.
Given the extensive homology between the two catalytic domains, it is not surprising that ROCK1 and ROCK2 have common substrates in vitro or during overexpression conditions. Any divergences in substrate specificity and, by extension, differences in biological activities are probably due to subtle variations in subcellular localization [32], which might be influenced through interactions with plasma membrane lipids [15] as well as with adaptor proteins such as Shroom3 [33,34] or dynamin 1 [35]. For example, by using siRNA-mediated knockdown, it was observed that ROCK1 promotes focal adhesion maturation [36] and keratinocyte differentiation [37], whereas ROCK2 inhibits keratinocyte differentiation [37], increases adhesion complex turnover [36] and promotes expression of adhesion molecules on endothelial cells [38]. Early studies in genetically modified mice showed a similar pattern of impairments in epithelial sheet movement in either ROCK1 or ROCK2 homozygous knockouts in the C57BI/6 strain, which was phenocopied in double ROCK1/ROCK2 heterozygotes [39,40,41]. However, both ROCK1 and ROCK2 knockouts were not affected by these defects in epithelial sheet movement in other mouse strains [42,43]. These data suggest that the in vivo functions of the two kinases are largely analogous and compensatory. Some differences in tissue expression patterns have been detected that could result in apparently isoform-specific functions in some cell types. For example, ROCK1 is expressed in 52 out of 66 cell types, with the highest levels found in haematopoietic and digestive organs, whereas ROCK2 expression was found to be expressed in 49 out of 65 cell types with the highest levels found in the central nervous system (www.proteinatlas.org). As mentioned above, the development of methods that report on ROCK activity, such as antibodies against autophosphorylation sites, rather than just changes in expression levels, would help generate a refined assessment of cancer types that could benefit from inhibitor treatments. As it has been proposed that ROCK1 is dominant in regulating vascular smooth muscle tone, it has been suggested that small molecule inhibitors that selectively target ROCK2 over ROCK1 might provide therapeutic efficacy whilst avoiding the profound hypotensive effects induced by pan-ROCK inhibition [44,45]. To this end, both traditional high-throughput library screens and fragment-based drug discovery efforts have yielded compounds that are reported to have significant selectivity for ROCK2 relative to ROCK1 [46,47,48]. Structural solutions of ROCK1 complexes with inhibitors such as Y27632, fasudil, hydroxyfasudil and H-1152P provide a significant amount of structure–activity relationship data for improving potency and selectivity [28,46,49,50]. The next advance in determining whether the hypothesis that targeting ROCK2 would be adequate for cancer treatment, and superior to pan-ROCK inhibition for limiting adverse effects, will come from in vivo studies using compounds with ROCK2 selectivity and from conditional tissue-selective ROCK2 knockout experiments in genetically modified mouse cancer models.
MicroRNA-mediated regulation of ROCK
The miRNA prediction database ‘TargetScan’ predicts several binding sites for miRNAs in the 3′ UTRs of ROCK1 and ROCK2 (http://www.targetscan.org). Interestingly, their 3′ UTRs comprise different sets of miRNA-binding sites, indicating an additional potential regulatory level for distinct spatial and temporal expression of either ROCK1 or ROCK2. Validation of post-transcriptional regulation of ROCK expression by miRNAs has been described in several studies, in which analyses of various tumour types have revealed inverse correlations between ROCK1 or ROCK2 expression and specific miRNAs. In primary hepatocellular carcinomas (HCCs) miR-139 was found to be downregulated whereas ROCK2 expression was increased, which was associated with poor prognosis of patients, whilst conversely miR-139 re-expression directly suppressed ROCK2 protein levels in HCC cell lines [51]. Reduced expression of the ROCK2-targeting miR-124 in HCC also correlated with increased ROCK2 expression and poor patient prognosis, whilst overexpression of miR-124 in HCC cell lines repressed ROCK2 expression [52]. The expression of miR-138 is reduced in lung cancers [53], thyroid carcinoma [54] and tongue squamous cell carcinoma [55], in which it was shown to negatively regulate RhoC and ROCK2 expression [56]. ROCK1 was found to be a target of miR-584 in renal cell carcinoma cell lines [57]. The overall poor survival of neuroblastoma patients was associated with elevated MYCN expression, which represses miR-335, thereby leading to increased levels of several proteins including ROCK1 [58]. Finally, the transition from hormone-dependent to hormone-refractory prostate cancer was associated with decreased miR-146a levels, which targets ROCK1 [59]. These studies demonstrate that the additional layer of regulation provided by miRNAs influences ROCK1 and ROCK2 expression in specific cancers. Although there is evidence that decreased expression of specific miRNAs has direct consequences for the actomyosin cytoskeleton mediated by increased ROCK1 or ROCK2 expression, it remains to be determined whether the cancer-promoting effects of miRNA repression can be universally attributed to ROCK1 or ROCK2 distinct from additional potential target transcripts. If it were true that increased ROCK1 or ROCK2 levels were the main tumorigenic effectors for specific tumour-suppressing miRNAs, then cancers with reduced levels of these miRNAs would be candidates for ROCK inhibitor therapy.
ROCK and the tumour microenvironment
There has been a great deal of research establishing the cell-autonomous role of ROCK signalling in tumour cell motility [60]. As individuals, cells can migrate in rounded or elongated shapes and often have the ability to convert between these modes dependent on the external environment [61]. Alternatively, tumour cells might maintain cell–cell adhesions and invade collectively [60]. The rounded (also called amoeboid) type of migration depends on Rho/ROCK-induced actomyosin contractility that results from increased MLC phosphorylation to promote the formation of bleb-like protrusions [62,63]. In line with this, cells using the rounded mode of movement are sensitive to Rho and ROCK inhibitors [62]. Furthermore, ROCK signalling suppresses the elongated (also called mesenchymal) type of migration in favour of the rounded mode by inhibiting Rac activity [64].
In addition to cell-autonomous factors, investigations have revealed that cancer-associated fibroblasts (CAFs) in adjacent stroma have a substantial impact on cancer cell invasion [65]. Organotypic assays simulating an epidermal and dermal environment using squamous cell carcinoma cells (SCC12) and CAFs revealed that ROCK activity is actually required in the fibroblasts, rather than in the tumour cells, to remodel collagen matrices and thereby generate tracks for the invading SCC12 cells [66]. Instead of being ROCK-dependent, the ability of SCC12 cells to invade collectively depends on the related Cdc42-regulated MRCK kinase to phosphorylate MLC and generate the actomyosin-mediated contractile force necessary for cell movement [66]. The ROCK activity in CAFs necessary for SCC12 invasion might be driven by cell-autonomous factors, or might be due to proinflammatory cytokines such as IL-6 or oncostatin M in the tumour microenvironment signalling through JAK1–STAT3 pathways [67]. Similarly, autocrine IL-1 produced by SCC12 cells promotes the production and release of TNFα, which acts on CAFs to promote contractility and SCC invasion [68]. Interestingly, ROCK-induced actomyosin contractility positively feeds-forward to JAK1–STAT3 signalling, indicating that there is a self-reinforcing positive feedback loop [67]. Therefore, inhibition of ROCK signalling would interrupt both intrinsic and microenvironment-derived extrinsic signals that promote CAF-facilitated invasion, and could potentially have a sustained effect by breaking the positive feedback loop.
There has been an appreciation that the stiff tissue surrounding tumours is not merely a passive by-product, but is actually an active participant in tumour growth and progression [69]. Deposition and modification of extracellular matrix components, such as collagen, can lead to activation of signal transduction pathways that promote tumour cell growth, proliferation and survival [70]. One pathway that is activated is the Rho–ROCK signalling axis, which leads to increased actomyosin bundling and contractile force generation in an attempt to balance the external forces experienced with internal cytoskeletal structural reinforcement [71]. To determine how ROCK activation would affect homeostasis and cancer in mouse skin—an epithelial tissue known to be influenced by mechanical force—we expressed an oestrogen-regulated conditionally active form of ROCK2 under the transcriptional regulation of the cytokeratin 14 promoter [72,73]. We found that ROCK activation was sufficient to promote significant collagen deposition and elevated tissue stiffness, resulting in increased basal keratinocyte proliferation and epidermal thickening (Fig 3). These responses to tissue stiffness were mediated by a signalling pathway that led to stabilization and activation of the transcription promoting function of β-catenin. Inhibition of either LIM kinases or myosin ATPase, which act downstream from ROCK to drive actomyosin contraction, was sufficient to reverse the effects of ROCK activation, indicating that cytoskeletal contractile force generation was the key ROCK-induced cellular response. In addition, inhibition of focal adhesion kinase (FAK)—which acts in response to integrin activation, ultimately leading to β-catenin accumulation and activation—or lysyl oxidase—which cross-links collagen to increase extracellular matrix stiffness—also blocked hyperproliferation and epidermal thickening. When ROCK was activated in the context of a two-stage chemical carcinogenesis skin cancer model, there were more benign papillomas that grew faster and progressed more rapidly to become invasive carcinoma relative to controls. Conversely, application of the ROCK inhibitor Y27632 reduced papilloma number, growth and progression to malignancy. The implications of these findings are that in some cancers internal and external forces might be engaged in a positive-feedback mechanical autocrine loop that results in ever-increasing tissue stiffness in regions surrounding tumours. Drugs that break this mechanical autocrine loop might have beneficial effects by reducing the stiffness of tumour-associated tissue. For example, this could include blocking of collagen cross-linking to reduce external stiffness, or inhibiting actomyosin contractility—for example, by ROCK and LIMK inhibitors—to reduce internal cellular tension. Alternatively, inhibition of pathways activated by external tissue stiffness, such as FAK and PI3K, could also have beneficial effects independently of cell-autonomous factors. Clinical trials are under way in which the production of extracellular matrix proteins in pancreatic cancer will be targeted with Hedgehog antagonists to reduce stromal desmoplasia and increase delivery of cytotoxic agents such as gemcitabine (www.clinicaltrials.gov; [74]). Therefore, a further possible benefit of breaking the mechanical autocrine loop that results in elevated collagen deposition could be improved responses to standard chemotherapeutics.
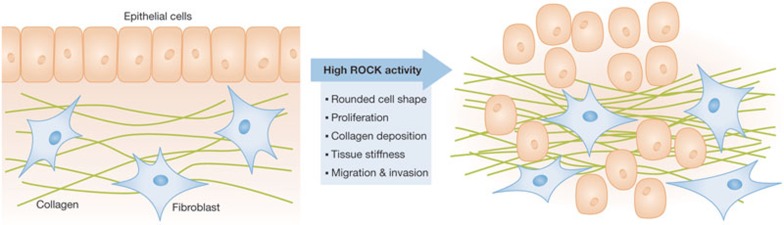
ROCK actions on epithelial tumour growth and progression. ROCK activation results in several changes at the cellular level: cells round up, lose cell–cell contacts and might be more able to become disseminated. Moreover, pathways leading to modifications in the microenvironment are activated—for example, increased collagen deposition promoting elevated tissue stiffness. These cell and non-cell-autonomous responses occur during the transition of normal cells to cancer cells and eventually promote tumour growth and metastasis. ROCK, Rho-associated coiled-coil protein kinase.
ROCK contributes to K-Ras-induced lung cancers
One attractive property of potential cancer drug targets is a selective requirement in cancer cells over normal cells. Screening of siRNA libraries for genes necessary for growth and survival of non-small-cell lung cancer cells expressing mutant K-Ras, but not for cells expressing wild-type K-Ras, identified the transcription factor GATA2 as an essential factor [75]. Sequencing of genomic DNA associated with GATA2 in K-Ras-expressing cells identified ROCK1 and EPHA3—which acts as an ephrin receptor and Rho pathway activator—as transcriptional targets that promote increased MLC phosphorylation. Interestingly, although ROCK activation was sufficient to restore viability when GATA2 was repressed, treatment with ROCK inhibitors or siRNA-mediated knockdown of ROCK1 on their own did not affect cell survival. When combined with proteasome inhibition, treatment with ROCK inhibitors was effective in killing cells. Proteasome inhibitors, for example bortezomib, have been evaluated and recommended for the treatment of progressive multiple myeloma (www.nice.org.uk/TA129), and ROCK inhibitors—for example fasudil—have been used safely for several years in Japan for the treatment of subarachnoid haemorrhage after a head trauma [11]. Therefore, it should be possible for combination trials to be undertaken on non-small-cell lung cancer patients with mutant K-Ras by using readily available compounds.
ROCK in haematological malignancies
Advances in molecular medicine led to the identification of activating mutations and translocations in tyrosine kinases (TK), such as KIT, FLT3 and BCR-ABL, that drive haematological malignancies [76]. These results spurred development of molecularly targeted tyrosine kinase inhibitors (TKIs), such as imatinib mesylate, that have proven to be effective in the treatment of chronic myeloid leukaemia (CML). However, one issue with TKI therapies is the emergence of resistance and consequent relapse over time, often due to selection for secondary mutations that confer TKI insensitivity. In addition, cessation of TKI administration in best-responding patients leads to relapse in about 60% of the patient population. This indicates that although therapy might be effective at reducing the bulk of malignant cells and alleviating symptoms whilst allowing for re-growth of normal bone marrow, CML stem cells that can re-establish disease are intrinsically TKI resistant.
Although TKIs have proven to be a major therapeutic advance, combination therapies are more likely to overcome problems of drug resistance and relapse due to the difficulty in killing CML stem cells. Combination therapies might be comprised of multiple inhibitors against related target classes, for example the combination of imatinib mesylate plus dasatinib [77], or different points of action, for example imatinib mesylate plus histone deacetylase inhibitors [78]. Rho and ROCK regulation of the actomyosin cytoskeleton might also be a target for treatment of oncogenic TK-driven malignancies, either alone or in combination with TKIs [79]. Rho and ROCK are activated in several blood cancers including CML [79] and AML [80]. In fact, a chromosomal translocation that resulted in the activation of the LARG RhoGEF was implicated as the cause of AML in one case [80]. Chromosomal translocations [81] or epigenetic silencing [82,83] that result in reduced activity of the ARHGAP26 Rho GTPase accelerating protein 26 (RhoGAP), which diminishes RhoA activity, have also been reported in numerous AML patients. These findings suggest that Rho, or ROCK activation downstream from Rho, might contribute to haematological malignancies and would therefore be potential chemotherapeutic targets. Consistently, inhibition of Rho with the Clostridium botulinum C3 ADP-ribosylase exoenzyme induced apoptosis and/or decreased proliferation of BaF and K562 CML cell lines expressing oncogenic BCR-ABL [84], in oncogenic KIT or FLT3 tyrosine kinase-expressing 32D myeloid cells [79] and in cells isolated from a CML patient [85]. When the murine myeloid 32D cell line was oncogenically transformed by active KIT, BCR-ABL or FLT3, ROCK substrate phosphorylation was elevated and constitutive growth of oncogene-bearing cells was sensitive to ROCK inhibition [79]. Cells expressing a pan-TKI resistant BCR-ABL T315I mutant were sensitive to ROCK inhibition. Primary haematopoietic progenitor cells that were made cytokine-independent by oncogene expression also showed reduced proliferation when treated with ROCK inhibitors. Interestingly, knockdown of MLC had the same effect as ROCK inhibition, consistent with ROCK acting through MLC. The survival of mice transplanted with leukaemic cells was prolonged by ROCK inhibition. Importantly, imatinib mesylate synergized with ROCK inhibitors to kill CD34-positive CML stem cells from human patients [86], suggesting that these drug combinations might overcome the issues with relapse occurring after TKI administration. One of the most intriguing observations is that expression of active ROCK alone, in transplanted cells, was sufficient to induce myeloproliferative disease with pronounced splenomegaly and hepatomegaly [79]. These results indicate that the ROCK-regulated actomyosin cytoskeleton makes essential contributions to the survival and growth of TK-driven haematological malignancies, and suggest that inhibitors of ROCK or other downstream actomyosin regulators could be effective therapeutics as single agents or in combination with TKIs. Since imatinib has been recommended as a first-line treatment of CML (http://guidance.nice.org.uk/TA251), and the ROCK inhibitor fasudil has been used safely for some years in Japan [11], it should be possible to test this combination in clinical trials.
Concluding remarks
Despite the initial early promise and marked interest in ROCK as a potential cancer therapeutic target, ROCK inhibitors have not yet progressed to clinical use (Sidebar A). One limitation has been a lack of clarity as to the most relevant tumour types. Most of the data on ROCK expression in cancer are anecdotal, in fact some studies have made use of invalidated antibody reagents that might not be specific. Progress will be made with the rigorous validation of ROCK1 and ROCK2 selective antibodies, the development of phosphorylation-specific antibodies that inform kinase activation status, and systematic evaluation of their immunoreactivity in normal compared with tumour tissue (Fig 4). There are many ROCK inhibitors that could potentially be used for cancer therapies [87]. The well-established ROCK inhibitor Y27632 is effective in vitro and has become the gold standard for basic research, but it is not selective nor was it optimized for properties necessary for in vivo use. Similarly, fasudil is not highly selective, but does have the advantage of having been used safely in humans for a number of years in Japan. Progress in basic and pre-clinical research will be made with new optimized inhibitors, possibly by using those with a marked selectivity for ROCK2 over ROCK1.
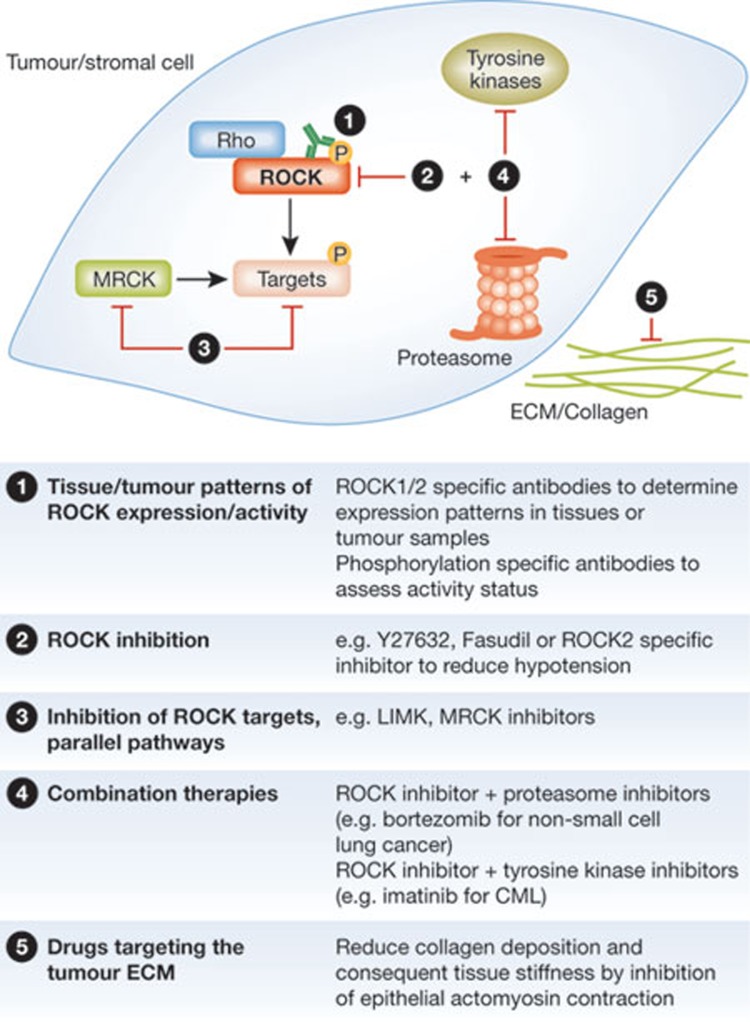
ROCK signalling: potential for diagnosis and treatment of cancer. Although there are no standard therapies using ROCK inhibition for cancer treatment, there are possible approaches for the translation of our knowledge of ROCK signalling into clinical use. Antibodies can be used to evaluate tumour types suitable for therapy. The development of optimized inhibitors blocking ROCK signalling proteins themselves and proteins in parallel or downstream pathways, in tumour cells as well as stromal cells, could be useful for treatment in the future. Furthermore, combination therapies as well as targeting the collagen in the extracellular matrix (ECM) might help to increase therapeutic efficiency. CML, chronic myeloid leukaemia; LIMK, LIN-11, Isl1 and MEC-3 domain kinase; MRCK, myotonic dystrophy kinase-related Cdc42-binding kinase; ROCK 1/2, Rho-associated coiled-coil protein kinase 1/2.
There has been an emerging realization that the use of molecularly targeted agents rapidly leads to resistance through several mechanisms, one being compensation by other participants in the cognate signalling network. Intuitively, it seems probable that ROCK2 selective inhibitors could result in elevated signalling through ROCK1 to compensate, possibly due to elevated ROCK1 expression or increased Rho activity. Additionally, increased signalling by downstream kinases, such as LIM kinases [88], or by kinases that have similar functions in actomyosin regulation, such as MRCK, could compensate for ROCK inhibition. Targeting of multiple points in a signalling cascade has been one strategy adopted for cancers driven by activation of the Ras–Raf–MEK–ERK pathway to avoid or delay the development of resistance [89], therefore a similar approach could be used by concomitantly targeting ROCK and LIM kinases (Fig 4). The combined inhibition of ROCK and MRCK kinases was found to be more effective in blocking actomyosin-mediated cell activities [29,90], and could help to avoid the development of resistance to ROCK inhibitors. Other drug combinations might produce greater anti-cancer effects by blocking completely independent targets that both have essential functions—for example, ROCK and proteasome inhibitors in non-small-cell lung cancer and ROCK and TKIs in CML—which might also help avoid or delay resistance to single agents (Fig 4). Further hypothesis-driven mechanistic studies, as well as unbiased screening efforts are needed to identify additional potential combinations with ROCK inhibitors that might ultimately lead to effective cancer treatments.
Finally, there seems to be considerable promise in targeting the tumour microenvironment as an alternative or complement to only treating the tumour cells (Fig 4). There is a great deal of interest in developing inhibitors for the collagen cross-linking lysyl oxidase to reduce extracellular matrix rigidity [91], whilst existing inhibitors that target Hedgehog signalling show promise in reducing the dense fibrotic stroma in pancreatic cancers [92]. Evidence indicates that blocking actomyosin contraction through ROCK inhibition, or cytokine signalling, could also have beneficial effects by reducing mechanical feedback loops that promote increased extracellular matrix protein deposition and tissue stiffness [67,68,73].
Acknowledgments
Work in the authors' laboratory is supported by Cancer Research UK. We thank T. Holyoake (University of Glasgow) for discussions regarding haematological malignancies.
References
- Hall A (2009) The cytoskeleton and cancer. Cancer Metastasis Rev 28: 5–14 [Abstract] [Google Scholar]
- Bishop AL, Hall A (2000) Rho GTPases and their effector proteins. Biochem J 348: 241–255 [Europe PMC free article] [Abstract] [Google Scholar]
- Riento K, Ridley AJ (2003) Rocks: multifunctional kinases in cell behaviour. Nat Rev Mol Cell Biol 4: 446–456 [Abstract] [Google Scholar]
- Amano M, Nakayama M, Kaibuchi K (2010) Rho-kinase/ROCK: A key regulator of the cytoskeleton and cell polarity. Cytoskeleton (Hoboken) 67: 545–554 [Europe PMC free article] [Abstract] [Google Scholar]
- Uehata M et al. (1997) Calcium sensitization of smooth muscle mediated by a Rho-associated protein kinase in hypertension. Nature 389: 990–994 [Abstract] [Google Scholar]
- Itoh K, Yoshioka K, Akedo H, Uehata M, Ishizaki T, Narumiya S (1999) An essential part for Rho-associated kinase in the transcellular invasion of tumor cells. Nat Med 5: 221–225 [Abstract] [Google Scholar]
- Sahai E, Ishizaki T, Narumiya S, Treisman R (1999) Transformation mediated by RhoA requires activity of ROCK kinases. Curr Biol 9: 136–145 [Abstract] [Google Scholar]
- Lochhead PA, Wickman G, Mezna M, Olson MF (2010) Activating ROCK1 somatic mutations in human cancer. Oncogene 29: 2591–2598 [Abstract] [Google Scholar]
- Liu P et al. (2012) Identification of somatic mutations in non-small cell lung carcinomas using whole-exome sequencing. Carcinogenesis 33: 1270–1276 [Europe PMC free article] [Abstract] [Google Scholar]
- Holbrook JD, Parker JS, Gallagher KT, Halsey WS, Hughes AM, Weigman VJ, Lebowitz PF, Kumar R (2011) Deep sequencing of gastric carcinoma reveals somatic mutations relevant to personalized medicine. J Transl Med 9: 119. [Europe PMC free article] [Abstract] [Google Scholar]
- Olson MF (2008) Applications for ROCK kinase inhibition. Curr Opin Cell Biol 20: 242–248 [Europe PMC free article] [Abstract] [Google Scholar]
- Dong M, Yan BP, Yu CM (2009) Current status of rho-associated kinases (ROCKs) in coronary atherosclerosis and vasospasm. Cardiovasc Hematol Agents Med Chem 7: 322–330 [Abstract] [Google Scholar]
- Oka M, Fagan KA, Jones PL, McMurtry IF (2008) Therapeutic potential of RhoA/Rho kinase inhibitors in pulmonary hypertension. Br J Pharmacol 155: 444–454 [Europe PMC free article] [Abstract] [Google Scholar]
- Tu D, Li Y, Song HK, Toms AV, Gould CJ, Ficarro SB, Marto JA, Goode BL, Eck MJ (2011) Crystal structure of a coiled-coil domain from human ROCKI. PLoS ONE 6: e18080. [Europe PMC free article] [Abstract] [Google Scholar]
- Wen W, Liu W, Yan J, Zhang M (2008) Structure basis and unconventional lipid membrane binding properties of the PH-C1 tandem of Rho kinases. J Biol Chem 283: 26263–26273 [Europe PMC free article] [Abstract] [Google Scholar]
- Fujisawa K, Fujita A, Ishizaki T, Saito Y, Narumiya S (1996) Identification of the Rho-binding domain of p160ROCK, a Rho-associated coiled-coil containing protein kinase. J Biol Chem 271: 23022–23028 [Abstract] [Google Scholar]
- Blumenstein L, Ahmadian MR (2004) Models of the cooperative mechanism for Rho effector recognition: implications for RhoA-mediated effector activation. J Biol Chem 279: 53419–53426 [Abstract] [Google Scholar]
- Dvorsky R, Blumenstein L, Vetter IR, Ahmadian MR (2004) Structural insights into the interaction of ROCKI with the switch regions of RhoA. J Biol Chem 279: 7098–7104 [Abstract] [Google Scholar]
- Riento K, Guasch RM, Garg R, Jin B, Ridley AJ (2003) RhoE binds to ROCKI and inhibits downstream signaling. Mol Cell Biol 23: 4219–4229 [Europe PMC free article] [Abstract] [Google Scholar]
- Ward Y, Yap SF, Ravichandran V, Matsumura F, Ito M, Spinelli B, Kelly K (2002) The GTP binding proteins Gem and Rad are negative regulators of the Rho–Rho kinase pathway. J Cell Biol 157: 291–302 [Europe PMC free article] [Abstract] [Google Scholar]
- Komander D, Garg R, Wan PT, Ridley AJ, Barford D (2008) Mechanism of multi-site phosphorylation from a ROCK-I:RhoE complex structure. EMBO J 27: 3175–3185 [Europe PMC free article] [Abstract] [Google Scholar]
- Coleman ML, Sahai EA, Yeo M, Bosch M, Dewar A, Olson MF (2001) Membrane blebbing during apoptosis results from caspase-mediated activation of ROCKI. Nat Cell Biol 3: 339–345 [Abstract] [Google Scholar]
- Sebbagh M, Renvoize C, Hamelin J, Riche N, Bertoglio J, Breard J (2001) Caspase-3-mediated cleavage of ROCKI induces MLC phosphorylation and apoptotic membrane blebbing. Nat Cell Biol 3: 346–352 [Abstract] [Google Scholar]
- Sebbagh M, Hamelin J, Bertoglio J, Solary E, Breard J (2005) Direct cleavage of ROCK II by granzyme B induces target cell membrane blebbing in a caspase-independent manner. J Exp Med 201: 465–471 [Europe PMC free article] [Abstract] [Google Scholar]
- Pinner S, Sahai E (2008) PDK1 regulates cancer cell motility by antagonising inhibition of ROCK1 by RhoE. Nat Cell Biol 10: 127–137 [Abstract] [Google Scholar]
- Lowery DM et al. (2007) Proteomic screen defines the Polo-box domain interactome and identifies Rock2 as a Plk1 substrate. EMBO J 26: 2262–2273 [Europe PMC free article] [Abstract] [Google Scholar]
- Lee HH, Tien SC, Jou TS, Chang YC, Jhong JG, Chang ZF (2010) Src-dependent phosphorylation of ROCK participates in regulation of focal adhesion dynamics. J Cell Sci 123: 3368–3377 [Abstract] [Google Scholar]
- Jacobs M, Hayakawa K, Swenson L, Bellon S, Fleming M, Taslimi P, Doran J (2006) The structure of dimeric ROCKI reveals the mechanism for ligand selectivity. J Biol Chem 281: 260–268 [Abstract] [Google Scholar]
- Heikkila T et al. (2011) Co-crystal structures of inhibitors with MRCKbeta, a key regulator of tumor cell invasion. PLoS ONE 6: e24825. [Europe PMC free article] [Abstract] [Google Scholar]
- Pearce LR, Komander D, Alessi DR (2010) The nuts and bolts of AGC protein kinases. Nat Rev Mol Cell Biol 11: 9–22 [Abstract] [Google Scholar]
- Chuang HH, Yang CH, Tsay YG, Hsu CY, Tseng LM, Chang ZF, Lee HH (2012) ROCKII Ser1366 phosphorylation reflects the activation status. Biochem J 443: 145–151 [Abstract] [Google Scholar]
- Yoneda A, Multhaupt HA, Couchman JR (2005) The Rho kinases I and II regulate different aspects of myosin II activity. J Cell Biol 170: 443–453 [Europe PMC free article] [Abstract] [Google Scholar]
- Nishimura T, Takeichi M (2008) Shroom3-mediated recruitment of Rho kinases to the apical cell junctions regulates epithelial and neuroepithelial planar remodeling. Development 135: 1493–1502 [Abstract] [Google Scholar]
- Mohan S, Rizaldy R, Das D, Bauer RJ, Heroux A, Trakselis MA, Hildebrand JD, VanDemark AP (2012) Structure of Shroom domain 2 reveals a three-segmented coiled-coil required for dimerization, Rock binding, and apical constriction. Mol Biol Cell 23: 2131–2142 [Europe PMC free article] [Abstract] [Google Scholar]
- Tumusiime S, Rana MK, Kher SS, Kurella VB, Williams KA, Guidry JJ, Worthylake DK, Worthylake RA (2009) Regulation of ROCKII by localization to membrane compartments and binding to DynaminI. Biochem Biophys Res Commun 381: 393–396 [Europe PMC free article] [Abstract] [Google Scholar]
- Lock FE, Ryan KR, Poulter NS, Parsons M, Hotchin NA (2012) Differential regulation of adhesion complex turnover by ROCK1 and ROCK2. PLoS ONE 7: e31423. [Europe PMC free article] [Abstract] [Google Scholar]
- Lock FE, Hotchin NA (2009) Distinct roles for ROCK1 and ROCK2 in the regulation of keratinocyte differentiation. PLoS ONE 4: e8190. [Europe PMC free article] [Abstract] [Google Scholar]
- Shimada H, Rajagopalan LE (2010) Rho kinase-2 activation in human endothelial cells drives lysophosphatidic acid-mediated expression of cell adhesion molecules via NF-kappaB p65. J Biol Chem 285: 12536–12542 [Europe PMC free article] [Abstract] [Google Scholar]
- Thumkeo D, Keel J, Ishizaki T, Hirose M, Nonomura K, Oshima H, Oshima M, Taketo MM, Narumiya S (2003) Targeted disruption of the mouse rho-associated kinase 2 gene results in intrauterine growth retardation and fetal death. Mol Cell Biol 23: 5043–5055 [Europe PMC free article] [Abstract] [Google Scholar]
- Shimizu Y et al. (2005) ROCK-I regulates closure of the eyelids and ventral body wall by inducing assembly of actomyosin bundles. J Cell Biol 168: 941–953 [Europe PMC free article] [Abstract] [Google Scholar]
- Thumkeo D, Shimizu Y, Sakamoto S, Yamada S, Narumiya S (2005) ROCK-I and ROCK-II cooperatively regulate closure of eyelid and ventral body wall in mouse embryo. Genes Cells 10: 825–834 [Abstract] [Google Scholar]
- Zhang YM et al. (2006) Targeted deletion of ROCK1 protects the heart against pressure overload by inhibiting reactive fibrosis. FASEB J 20: 916–925 [Abstract] [Google Scholar]
- Zhou Z, Meng Y, Asrar S, Todorovski Z, Jia Z (2009) A critical role of Rho-kinase ROCK2 in the regulation of spine and synaptic function. Neuropharmacology 56: 81–89 [Abstract] [Google Scholar]
- Doe C et al. (2007) Novel Rho kinase inhibitors with anti-inflammatory and vasodilatory activities. J Pharmacol Exp Ther 320: 89–98 [Abstract] [Google Scholar]
- Schueller O, Tong W, Ferkany JW, Sweetnam P (2006) Abstract 1216: Selective ROCK 2 inhibition attenuates arterial plaque formation in an ApoE knockout mouse model. Circulation 114: II–228 [Google Scholar]
- Li R et al. (2012) Fragment-based and structure-guided discovery and optimization of Rho kinase inhibitors. J Med Chem 55: 2474–2478 [Europe PMC free article] [Abstract] [Google Scholar]
- Boerma M et al. (2008) Comparative gene expression profiling in three primary human cell lines after treatment with a novel inhibitor of Rho kinase or atorvastatin. Blood Coagul Fibrinolysis 19: 709–718 [Europe PMC free article] [Abstract] [Google Scholar]
- Feng Y, Cameron MD, Frackowiak B, Griffin E, Lin L, Ruiz C, Schroter T, LoGrasso P (2007) Structure-activity relationships, and drug metabolism and pharmacokinetic properties for indazole piperazine and indazole piperidine inhibitors of ROCK-II. Bioorg Med Chem Lett 17: 2355–2360 [Abstract] [Google Scholar]
- Bosanac T et al. (2010) Substituted 2H-isoquinolin-1-ones as potent Rho-kinase inhibitors: part 3, aryl substituted pyrrolidines. Bioorg Med Chem Lett 20: 3746–3749 [Abstract] [Google Scholar]
- Ginn JD et al. (2010) Substituted 2H-isoquinolin-1-ones as potent Rho-kinase inhibitors: part 2, optimization for blood pressure reduction in spontaneously hypertensive rats. Bioorg Med Chem Lett 20: 5153–5156 [Abstract] [Google Scholar]
- Wong CC, Wong CM, Tung EK, Au SL, Lee JM, Poon RT, Man K, Ng IO (2011) The microRNA miR-139 suppresses metastasis and progression of hepatocellular carcinoma by down-regulating Rho-kinase 2. Gastroenterology 140: 322–331 [Abstract] [Google Scholar]
- Zheng F et al. (2012) The putative tumour suppressor microRNA-124 modulates hepatocellular carcinoma cell aggressiveness by repressing ROCK2 and EZH2. Gut 61: 278–289 [Abstract] [Google Scholar]
- Seike M et al. (2009) MiR-21 is an EGFR-regulated anti-apoptotic factor in lung cancer in never-smokers. Proc Natl Acad Sci USA 106: 12085–12090 [Europe PMC free article] [Abstract] [Google Scholar]
- Mitomo S et al. (2008) Downregulation of miR-138 is associated with overexpression of human telomerase reverse transcriptase protein in human anaplastic thyroid carcinoma cell lines. Cancer Sci 99: 280–286 [Abstract] [Google Scholar]
- Wong TS, Liu XB, Wong BY, Ng RW, Yuen AP, Wei WI (2008) Mature miR-184 as potential oncogenic microRNA of squamous cell carcinoma of tongue. Clin Cancer Res 14: 2588–2592 [Abstract] [Google Scholar]
- Jiang L, Liu X, Kolokythas A, Yu J, Wang A, Heidbreder CE, Shi F, Zhou X (2010) Downregulation of the Rho GTPase signaling pathway is involved in the microRNA-138-mediated inhibition of cell migration and invasion in tongue squamous cell carcinoma. Int J Cancer 127: 505–512 [Europe PMC free article] [Abstract] [Google Scholar]
- Ueno K, Hirata H, Shahryari V, Chen Y, Zaman MS, Singh K, Tabatabai ZL, Hinoda Y, Dahiya R (2011) Tumour suppressor microRNA-584 directly targets oncogene Rock-1 and decreases invasion ability in human clear cell renal cell carcinoma. Br J Cancer 104: 308–315 [Europe PMC free article] [Abstract] [Google Scholar]
- Lynch J, Fay J, Meehan M, Bryan K, Watters KM, Murphy DM, Stallings RL (2012) MiRNA-335 suppresses neuroblastoma cell invasiveness by direct targeting of multiple genes from the non-canonical TGF-β signalling pathway. Carcinogenesis 33: 976–985 [Europe PMC free article] [Abstract] [Google Scholar]
- Lin SL, Chiang A, Chang D, Ying SY (2008) Loss of mir-146a function in hormone-refractory prostate cancer. RNA 14: 417–424 [Europe PMC free article] [Abstract] [Google Scholar]
- Olson MF, Sahai E (2009) The actin cytoskeleton in cancer cell motility. Clin Exp Metastasis 26: 273–287 [Abstract] [Google Scholar]
- Croft DR, Olson MF (2008) Regulating the conversion between rounded and elongated modes of cancer cell movement. Cancer Cell 14: 349–351 [Abstract] [Google Scholar]
- Sahai E, Marshall CJ (2003) Differing modes of tumour cell invasion have distinct requirements for Rho/ROCK signalling and extracellular proteolysis. Nat Cell Biol 5: 711–719 [Abstract] [Google Scholar]
- Wyckoff JB, Pinner SE, Gschmeissner S, Condeelis JS, Sahai E (2006) ROCK- and myosin-dependent matrix deformation enables protease-independent tumor-cell invasion in vivo. Curr Biol 16: 1515–1523 [Abstract] [Google Scholar]
- Sanz-Moreno V, Gadea G, Ahn J, Paterson H, Marra P, Pinner S, Sahai E, Marshall CJ (2008) Rac activation and inactivation control plasticity of tumor cell movement. Cell 135: 510–523 [Abstract] [Google Scholar]
- Kalluri R, Zeisberg M (2006) Fibroblasts in cancer. Nat Rev Cancer 6: 392–401 [Abstract] [Google Scholar]
- Gaggioli C, Hooper S, Hidalgo-Carcedo C, Grosse R, Marshall JF, Harrington K, Sahai E (2007) Fibroblast-led collective invasion of carcinoma cells with differing roles for RhoGTPases in leading and following cells. Nat Cell Biol 9: 1392–1400 [Abstract] [Google Scholar]
- Sanz-Moreno V et al. (2011) ROCK and JAK1 signaling cooperate to control actomyosin contractility in tumor cells and stroma. Cancer Cell 20: 229–245 [Abstract] [Google Scholar]
- Chaudhry SI, Hooper S, Nye E, Williamson P, Harrington K, Sahai E (2012) Autocrine IL-1beta-TRAF6 signalling promotes squamous cell carcinoma invasion through paracrine TNFalpha signalling to carcinoma-associated fibroblasts. Oncogene [Epub ahead of print] ; DOI: 10.1038/onc.2012.91 [Europe PMC free article] [Abstract] [CrossRef] [Google Scholar]
- DuFort CC, Paszek MJ, Weaver VM (2011) Balancing forces: architectural control of mechanotransduction. Nat Rev Mol Cell Biol 12: 308–319 [Europe PMC free article] [Abstract] [Google Scholar]
- Lu P, Weaver VM, Werb Z (2012) The extracellular matrix: a dynamic niche in cancer progression. J Cell Biol 196: 395–406 [Europe PMC free article] [Abstract] [Google Scholar]
- Keely PJ (2011) Mechanisms by which the extracellular matrix and integrin signaling act to regulate the switch between tumor suppression and tumor promotion. J Mammary Gland Biol Neoplasia 16: 205–219 [Europe PMC free article] [Abstract] [Google Scholar]
- Samuel MS, Munro J, Bryson S, Forrow S, Stevenson D, Olson MF (2009) Tissue selective expression of conditionally-regulated ROCK by gene targeting to a defined locus. Genesis 47: 440–446 [Abstract] [Google Scholar]
- Samuel MS et al.. (2011) Actomyosin-mediated cellular tension drives increased tissue stiffness and β-catenin activation to induce epidermal hyperplasia and tumor growth. Cancer Cell 19: 776–791 [Europe PMC free article] [Abstract] [Google Scholar]
- Olive KP et al. (2009) Inhibition of Hedgehog signaling enhances delivery of chemotherapy in a mouse model of pancreatic cancer. Science 324: 1457–1461 [Europe PMC free article] [Abstract] [Google Scholar]
- Kumar MS et al. (2012) The GATA2 transcriptional network is requisite for RAS oncogene-driven non-small cell lung cancer. Cell 149: 642–655 [Abstract] [Google Scholar]
- Hamilton A, Gallipoli P, Nicholson E, Holyoake TL (2010) Targeted therapy in haematological malignancies. J Pathol 220: 404–418 [Abstract] [Google Scholar]
- Bradeen HA, Eide CA, O'Hare T, Johnson KJ, Willis SG, Lee FY, Druker BJ, Deininger MW (2006) Comparison of imatinib mesylate, dasatinib (BMS-354825), and nilotinib (AMN107) in an N-ethyl-N-nitrosourea (ENU)-based mutagenesis screen: high efficacy of drug combinations. Blood 108: 2332–2338 [Europe PMC free article] [Abstract] [Google Scholar]
- Zhang B et al. (2010) Effective targeting of quiescent chronic myelogenous leukemia stem cells by histone deacetylase inhibitors in combination with imatinib mesylate. Cancer Cell 17: 427–442 [Europe PMC free article] [Abstract] [Google Scholar]
- Mali RS et al. (2011) Rho kinase regulates the survival and transformation of cells bearing oncogenic forms of KIT, FLT3, and BCR-ABL. Cancer Cell 20: 357–369 [Europe PMC free article] [Abstract] [Google Scholar]
- Reuther GW, Lambert QT, Booden MA, Wennerberg K, Becknell B, Marcucci G, Sondek J, Caligiuri MA, Der CJ (2001) Leukemia-associated Rho guanine nucleotide exchange factor, a Dbl family protein found mutated in leukemia, causes transformation by activation of RhoA. J Biol Chem 276: 27145–27151 [Abstract] [Google Scholar]
- Somervaille TC, Cleary ML (2006) Identification and characterization of leukemia stem cells in murine MLL-AF9 acute myeloid leukemia. Cancer Cell 10: 257–268 [Abstract] [Google Scholar]
- Wei J et al. (2008) Microenvironment determines lineage fate in a human model of MLL-AF9 leukemia. Cancer Cell 13: 483–495 [Europe PMC free article] [Abstract] [Google Scholar]
- Qian J et al. (2011) Abnormal methylation of GRAF promoter Chinese patients with acute myeloid leukemia. Leuk Res 35: 783–786 [Abstract] [Google Scholar]
- Molli PR, Pradhan MB, Advani SH, Naik NR (2012) RhoA: a therapeutic target for chronic myeloid leukemia. Mol Cancer 11: 16. [Europe PMC free article] [Abstract] [Google Scholar]
- Selleri C, Maciejewski JP, Montuori N, Ricci P, Visconte V, Serio B, Luciano L, Rotoli B (2003) Involvement of nitric oxide in farnesyltransferase inhibitor-mediated apoptosis in chronic myeloid leukemia cells. Blood 102: 1490–1498 [Abstract] [Google Scholar]
- Burthem J, Rees-Unwin K, Mottram R, Adams J, Lucas GS, Spooncer E, Whetton AD (2007) The rho-kinase inhibitors Y-27632 and fasudil act synergistically with imatinib to inhibit the expansion of ex vivo CD34(+) CML progenitor cells. Leukemia 21: 1708–1714 [Abstract] [Google Scholar]
- Mardilovich K, Olson MF, Baugh M (2012) Targeting Rho GTPase signaling for cancer therapy. Future Oncol 8: 165–177 [Abstract] [Google Scholar]
- Scott RW, Olson MF (2007) LIM kinases: function, regulation and association with human disease. J Mol Med 85: 555–568 [Abstract] [Google Scholar]
- Poulikakos PI, Solit DB (2011) Resistance to MEK inhibitors: should we co-target upstream? Sci Signal 4: pe16. [Abstract] [Google Scholar]
- Wilkinson S, Paterson HF, Marshall CJ (2005) Cdc42-MRCK and Rho-ROCK signalling cooperate in myosin phosphorylation and cell invasion. Nat Cell Biol 7: 255–261 [Abstract] [Google Scholar]
- Nishioka T, Eustace A, West C (2012) Lysyl oxidase: from basic science to future cancer treatment. Cell Struct Funct 37: 75–80 [Abstract] [Google Scholar]
- Kelleher FC (2011) Hedgehog signaling and therapeutics in pancreatic cancer. Carcinogenesis 32: 445–451 [Abstract] [Google Scholar]
Articles from EMBO Reports are provided here courtesy of Nature Publishing Group
Full text links
Read article at publisher's site: https://doi.org/10.1038/embor.2012.127
Read article for free, from open access legal sources, via Unpaywall:
https://europepmc.org/articles/pmc3463970?pdf=render
Citations & impact
Impact metrics
Article citations
GLYAT suppresses liver cancer and clear cell renal cell carcinoma progression by downregulating ROCK1 expression.
Transl Cancer Res, 13(9):5097-5111, 27 Sep 2024
Cited by: 0 articles | PMID: 39430840 | PMCID: PMC11483444
Modulation of the LIMK Pathway by Myricetin: A Protective Strategy Against Neurological Impairments in Spinal Cord Injury.
Neurospine, 21(3):878-889, 30 Sep 2024
Cited by: 0 articles | PMID: 39363468 | PMCID: PMC11456951
Non-Muscle Myosin II A: Friend or Foe in Cancer?
Int J Mol Sci, 25(17):9435, 30 Aug 2024
Cited by: 0 articles | PMID: 39273383 | PMCID: PMC11395477
Review Free full text in Europe PMC
Molecular Mechanisms of Plant Extracts in Protecting Aging Blood Vessels.
Nutrients, 16(14):2357, 20 Jul 2024
Cited by: 0 articles | PMID: 39064801 | PMCID: PMC11279783
Review Free full text in Europe PMC
Rho-Associated Protein Kinase Activity Is Required for Tissue Homeostasis in the Xenopus laevis Ciliated Epithelium.
J Dev Biol, 12(2):17, 11 Jun 2024
Cited by: 0 articles | PMID: 38921484 | PMCID: PMC11204898
Go to all (198) article citations
Other citations
Wikipedia
Data
Data behind the article
This data has been text mined from the article, or deposited into data resources.
BioStudies: supplemental material and supporting data
Similar Articles
To arrive at the top five similar articles we use a word-weighted algorithm to compare words from the Title and Abstract of each citation.
Novel Insights into the Roles of Rho Kinase in Cancer.
Arch Immunol Ther Exp (Warsz), 64(4):259-278, 02 Jan 2016
Cited by: 100 articles | PMID: 26725045 | PMCID: PMC4930737
Review Free full text in Europe PMC
Rho/ROCK signaling in motility and metastasis of gastric cancer.
World J Gastroenterol, 20(38):13756-13766, 01 Oct 2014
Cited by: 57 articles | PMID: 25320513 | PMCID: PMC4194559
Review Free full text in Europe PMC
Preclinical to clinical utility of ROCK inhibitors in cancer.
Trends Cancer, 9(3):250-263, 02 Jan 2023
Cited by: 20 articles | PMID: 36599733
Review
Funding
Funders who supported this work.