Abstract
Free full text

How Do Astrocytes Participate in Neural Plasticity?
Abstract
Work over the past 20 years has implicated electrically nonexcitable astrocytes in complex neural functions. Despite controversies, it is increasingly clear that many, if not all, neural processes involve astrocytes. This review critically examines past work to identify the commonalities among the many published studies of neuroglia signaling. Although several studies have shown that astrocytes can impact short-term and long-term synaptic plasticity, further work is required to determine the requirement for astrocytic Ca2+ and other second messengers in these processes. One of the roadblocks to the field advancing at a rapid pace has been technical. We predict that the novel experimental tools that have emerged in recent years will accelerate the field and likely disclose an entirely novel path of neuroglia signaling within the near future.
The year 2014 represents the 20th anniversary of a pair of papers published by the writers that provided the first indication that astrocytes actively signal to neurons, and that these glial cells have the potential to be participants in the control of neural circuit function and behavior (Nedergaard 1994; Parpura et al. 1994). Although we have taken independent paths, we come together on this anniversary to discuss what we have learned since 1994, where we see the field progressing, and, finally, to discuss some key steps that we believe should be taken in the next decade to begin to further clarify the diverse roles that astrocytes play in brain function in health and disease.
Without knowledge of one another’s work, we published a pair of papers that provided the first demonstration that physiological changes in astrocytes influence neurons: stimulated Ca2+ changes in astrocytes led to delayed Ca2+ responses in neurons (Nedergaard 1994; Parpura et al. 1994). To set the backdrop to these studies, this was a period of explosive growth in Ca2+ imaging that resulted from the availability of fluorescent Ca2+ indicators and sensitive cameras to permit low-light-level imaging of intracellular biochemistry. As a consequence, there had been several recently published studies revealing that astrocytes show Ca2+ elevations in response to mechanical contact or even to the addition of neurotransmitters, such as glutamate (Cornell-Bell et al. 1990). However, the functional downstream consequences were unknown. In our studies, which used a combination of different stimuli—mechanical, optical, as well as a chemical transmitter bradykinin—we were able to show a robust impact of the astrocytic Ca2+ signal on the adjacent neurons (Nedergaard 1994; Parpura et al. 1994). Despite differences in mechanistic conclusions, these papers stimulated revised thinking about roles of astrocytes in the brain—perhaps these glial cells were actively signaling, albeit on a slower time scale, to modulate neurons, circuits, and, ultimately, behavior.
The subsequent two decades have been spent examining the signaling of astrocytes to neurons in more intact systems. As a consequence of this work, it is clear that astrocytes play critical roles in actively modulating brain function, although we are still putting the pieces of the puzzle in place to understand where, when, and how this process occurs naturally in vivo and when dysfunction can lead to disorders of the brain.
The field has gone through an explosive growth that has consisted of several phases. Initially, there was the dish phase in which the potential of the astrocyte was revealed in cell culture. These studies were essential as they captured our imagination and stimulated new thinking; however, they were limited by the fact that the properties of astrocytes can be different in vitro and in vivo. Next, we had the in situ phase, in which we asked whether similar processes could be detected in situ in acutely isolated brain slices. Then we began asking about roles in vivo through Ca2+ imaging together with two-photon microscopy as well as with molecular genetic alterations to permit the inhibition and stimulation of astrocytes. Each of these phases has offered unique insights, challenges, and opportunities for the field.
Through all of these phases, an emergent picture is developing in which it is without doubt that astrocytes play important roles in vivo but that the mechanisms are so diverse and complex that an understanding is still to emerge.
Some of the major challenges that we have faced and continue to face include: What are the endogenous signals of these glial cells? How can we stimulate astrocytes in a physiologically relevant manner? How can we inhibit astrocytes to determine when they are needed for brain function? And last, but by no means least, how diverse are astrocytes?
We are still at the early days of understanding astrocytes, and patience regarding functional interpretation is required. We make this statement because there have been apparently contradictory conclusions drawn from different studies. The individual observations are important as they help provide a fuller picture of the biology of these complex cells. However, the jury still needs further evidence before definitive conclusions can be drawn. For example, a plethora of studies have shown that Ca2+ signals stimulate gliotransmission and the consequent modulation of neurons and synapses (see Table 1). A significant endogenous source of the Ca2+ signal of the astrocyte is from the IP3-sensitive Ca2+ store. Thus, when an IP3R2 knockout mouse was used and it was found to have no impact on synaptic transmission and plasticity (Agulhon et al. 2010), the field was rocked. We do not question the data of the study; instead, we believe this is an important piece of information that ultimately needs to be put in context. In contrast, additional studies have shown that IP3 receptors are important for other aspects of astrocyte-induced synaptic modulation. A more recent study has shown that, in addition to Ca2+ release from internal stores, the influx of Ca2+ through transient receptor potential (TRP) channels is important for gliotransmission (Shigetomi et al. 2013). Clearly, such influx sites were unlikely to have been affected by the IP3R2 knockout and were shown to regulate d-serine release from the astrocyte. Another piece of the puzzle is added. Undoubtedly, there will be further twists and turns, but that is the joy of discovery, and it should be embraced.
Table 1.
Effect of Ca2+ signaling on excitatory or inhibitory potentials, slow inward current, synaptic failure, or neural bistability
Preparation | Method for inducing astrocytic Ca2+ signaling | Change in the frequency of EPSP or IPSP (%)a | Duration of modulation of EPSP or IPSPa | References |
---|---|---|---|---|
Hippocampal cocultures | Mechanical stimulation or photolysis of caged Ca2+ | - | 10–50 sec | Araque et al. 1998 |
Retina | Mechanical stimulation | Modulation of light- induced neural activity | 10–20 sec | Newman and Zahs 1998 |
Frog neuromuscular junction | Injection of GTP-γS in perisynaptic Schwann cells | Modulation of nerve-evoked synaptic responses | For the duration of the recordings | Robitaille 1998 |
Hippocampal slices | Train of depolarization | 10%–30% | ~60–120 sec | Jourdain et al. 2007 |
Hippocampal slices | Photolysis | 20%–30% decrease in synaptic failure | 50–60 sec | Perea and Araque 2007 |
Hippocampal slices | Agonists (ATP, UTP, FMRF) | ~20%–30% | 10–60 sec | Wang et al. 2012a |
Hippocampal slices | Photolysis of caged Ca2+b | ~30% | 10–60 sec | Wang et al. 2013 |
Slow inward current | ||||
Hippocampal slices | Agonist (DHPG) and photolysis of caged Ca2+ | Slow inward current | ~20–50 sec | Fellin et al. 2004 |
Hippocampal slices | Neuronal depolarization | Slow inward current | ~50 sec | Navarrete and Araque 2008 |
Decrease in synaptic failure rate | ||||
Hippocampal slices | Train of depolarization | ~20%–30% | 20 min | Kang et al. 1998 |
Hippocampal slices | Photolysis of caged Ca2+ | ~20%–30% | ~60 sec | Perea and Araque 2007 |
Hippocampal slices | Agonists | ~20%–30% | 10–60 sec | Wang et al. 2012a |
Hippocampal slices | Comparison of agonists and photolysis of caged Ca2+ | ~20%–30% | 10–60 sec | Wang et al. 2013 |
Bistability | ||||
Cortical slices | Trains of depolarization | Upstate synchronizations | - | Poskanzer and Yuste 2011 |
Cerebellar slices | Agonists (ATP, UTP, FMRF) | Increase in duration of upstate | 40–60 sec | Wang et al. 2012b |
All of the studies included in the table show that the modulatory effect on neural activity is Ca2+-dependent (BAPTA loading, thapsigargin, and/or use of transgenic mice with deletion of IP3R2 receptors).
DHPG, dihydroxyphenylglycine; EPSP, excitatory postsynaptic potential; IPSP, inhibitory postsynaptic potential.
aFor simplicity, EPSP and IPSP denote excitatory or inhibitory potentials or currents in both presence and absence of tetrodotoxin (TTX). Details can be found in the original papers.
bThe same study compared the effect of photolysis and agonist-induced astrocytic Ca2+ signaling and found that only photolysis, but not agonist exposure, induced changes in the frequency of EPSPs.
We believe that it is also important to be constrained when discussing Ca2+ as there is not just one type of Ca2+ signal. For example, there are global Ca2+ signals in which large somatic Ca2+ elevations arise and that can propagate as slow waves between adjacent astrocytes in slices preparation. In vivo, astrocytes in awake mice display global Ca2+ increases that often simultaneously engaged most cells within the field of view. Isolated oscillatory cellular Ca2+ signals can be restricted to one cell, and there are “spotty” Ca2+ signals that can be restricted to local microdomains (Shigetomi et al. 2013). It is possible, even likely, that each of these signals mediates different processes and more effort should focus on understanding the important functional distinction between each.
Other areas of interesting debate have concerned how gliotransmitters are released. Evidence exists for multiple mechanisms: exocytosis, anion transporters, and connexin hemichannels, to name a few. Significant evidence exists for each, and it is likely that all are used, although in different locales, and are recruited under differing conditions. A challenge is to perform precise experiments that allow the discrimination between each mechanism and to identify when each is recruited in physiology and/or pathology.
CA2+-DEPENDENT GLIOTRANSMITTER RELEASE
A wealth of studies over the past two decades has shown that astrocytes are active participants in synaptic transmission. Astrocytes provide not only metabolic support for hungry synapses, but also actively modulate synaptic strength. Work from multiple laboratories shows that astrocytes participate in synaptic transmission by releasing gliotransmitters that include glutamate, ATP, adenosine, or d-serine in slices. Many, although not all, of these studies have used brain slices prepared from juvenile rodent pups (reviewed in Araque et al. 1999; Nedergaard et al. 2003; Allen and Barres 2005) making it important to determine which of the observations are limited to postnatal development as opposed to physiology of mature systems. Progress in the field of neuroglia signaling has been so rapid in recent years that it requires us to take a step back and reflect on what the information generated by many groups actually tells us about the brain and how it functions.
Looking back at the literature on neuroglia signaling, it is clear that the most popular approach to studying the involvement of astrocytes in neural activity has been to record excitatory postsynaptic potentials (EPSP) or inhibitory postsynaptic potentials (IPSP) (Fig. 1). These studies have often used whole-cell patching combined with selective simulation of astrocytic Ca2+ signaling. Such analysis has shown that astrocytic Ca2+ signaling can trigger an increase in EPSP or IPSP frequencies and, in more selected cases, also increase the amplitudes of excitatory potentials. For example, in one study, mechanical-induced astrocytic Ca2+ signaling increased the frequency of EPSPs and IPSPs by 20%–50% in cocultures of astrocytes and neurons (Araque et al. 1998). Similarly, photolysis of caged IP3 triggered a transient increase in the EPSC frequency in the range of 20%–60% in hippocampal slices from 10- to 15-d-old mouse pups (Fiacco and McCarthy 2004). It is a typical finding among these and later studies on neuroglia signaling that the potentiation is transient and ceases after 20–60 sec (Table 1). One line of work has instead detected slow inward current mediated by extrasynaptic N-methyl-d-aspartate receptors (NMDARs) (NR2B) (Fellin et al. 2004). Another, albeit less popular, approach has been to evaluate the effect of astrocytic Ca2+ signaling on synapses with a high rate of failures. These studies are technically more difficult as they are based on either double patching of synaptically coupled neurons (Kang et al. 1998) or single patching combined with minimal stimulation (Perea and Araque 2007; Navarrete and Araque 2008; Wang et al. 2012a). The analysis from several laboratories shows that stimulation of Ca2+ signaling can reduce synaptic failure by 10%–30%, but the effect is short lived and does not persist beyond a few minutes (Table 1). Finally, in more recent papers, bistability of neural membrane potential has also been shown to be affected by stimulation of either cortical astrocytes (Poskanzer and Yuste 2011) or Bergmann glia (Wang et al. 2012b). In both cases, Ca2+ signaling resulted in short-lived (<1 min) changes in the stereotypical pattern of neuronal up and down states (Table 1). It is important to note that the modulatory effects of astrocytes are relatively modest and the conditions need to be optimized, including consistency of time of the day in which data are collected (see later discussion). It is therefore not surprising that several studies have failed to detect astrocytic regulation of synaptic transmission.
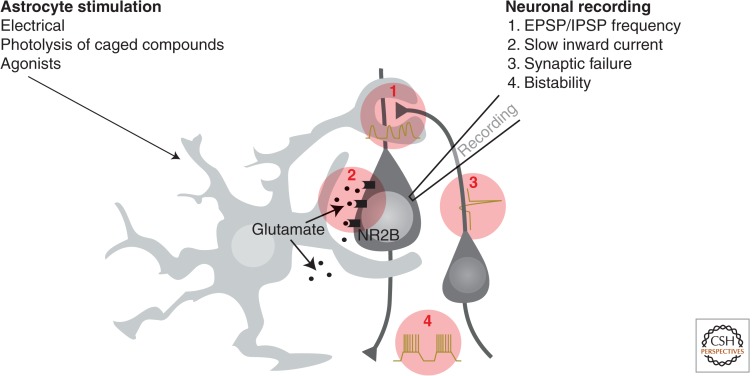
Common approaches to study astrocyte-to-neuron signaling. Astrocytic Ca2+ signaling is evoked by either photolysis of caged compounds (Ca2+ or IP3), train of depolarizations (electrical), or agonist application. The effect of astrocytic Ca2+ signaling is typically monitored by whole-cell patching of a nearby neuron. Most studies have recorded changes in either (1) the frequencies of EPSP/IPSP, (2) NR2B-mediated slow inward currents, (3) rate of synaptic failure in synaptically couple neurons, or (4) bistability of neuronal membrane potential. Recordings before, during, and after induction of astrocytic Ca2+ signaling is used to evaluate the potency by which astrocytes modulate neuronal activity. Table 1 compares the data from the many groups using these approaches.
Thus, work from multiple independent laboratories shows that astrocytes can transiently modulate synaptic transmission in slices prepared from young pups via a Ca2+-dependent pathway. One important take-home message from Table 1 is that the impact of astrocytes is limited to a timescale of tens of seconds. Astrocytic Ca2+ signaling, whether evoked by electrical stimulation, photolysis of caged compounds, or agonists, only evokes an ~10- to 60-sec-lasting modulation in the activity of nearby neurons. The similarities of the observations listed in Table 1 are remarkable considering that different approaches were used to both stimulate astrocytes and detect effects in neighboring neurons. This suggests that mobilization of astrocytic Ca2+ stores and subsequent increases in cytosolic Ca2+ may trigger a stereotypical response that can be detected as either changes in the frequency of synaptic potentials, as slow inward currents, changes in neuronal bistability of membrane potential, or as a decrease in extracellular K+ concentrations. Other lines of work indicate that Ca2+ increases are linked to a minor hyperpolarization (2–4 mV) rather than depolarizations of astrocytic membrane potential, as well as an increase in glycogenolysis. It is important to extend these observations into a full description of what intracellular processes are activated in astrocytes on increases in cytosolic Ca2+. The difficulties in developing a comprehensive understanding is that neural output measures in the past have represented the principal tool for detecting astrocytic responses to Ca2+ increases. Another important question that we address below is whether the modulation of the synapse is the sole, or primary, target of gliotransmission and whether astrocytes exert neuromodulation over different timescales.
THE IMPORTANCE OF EXTRACELLULAR K+ IN SETTING THE THRESHOLDS FOR GENERATION OF ACTION POTENTIALS
The foundation for neural activity is not limited to synaptic transmission. The ability of neurons to propagate action potentials forms the basis for all complex neural functions. What if astrocytes control or regulate the ability of neurons to generate action potentials? Modulation of the threshold by which action potentials are generated could potentially position astrocytes into a far more active role than previously recognized. The two key determinants for generation of action potentials are the resting neuronal membrane potential and the threshold for activation of voltage-gated sodium channels. Voltage-gated Na+ channels generate the steep depolarization characteristic of action potentials in neurons when the membrane potential reaches –55 mV or lower (Hounsgaard and Nicholson 1983). Thus, the voltage gap between the resting membrane potential and the threshold for activation of Na+ channels is an important factor determining the excitability of neural networks. According to the classical or “textbook” definition, it is the relative balance between excitatory and inhibitory input or the frequencies of EPSPs and IPSPs that determines when the membrane potential reaches the magical threshold of –55 mV at which an action potential is triggered. It is often forgotten that the resting membrane potential of neurons is highly sensitive to changes in extracellular K+. Even minor increases or decreases in K+ will enhance or reduce neural excitability by altering the gap between the resting membrane potential and activation of voltage-gated Na+ channels (Wang et al. 2012a). In slices, simply increasing both extracellular K+ leads to epileptiform activity (Traynelis and Dingledine 1988), and local cortical application of K+ triggers myoclonic seizures within minutes in awake wild-type mice (Rangroo Thrane et al. 2013). Not unexpectedly, the central nervous system (CNS) has invested considerable effort into maintaining extracellular K+ in a tightly controlled range of 3.5–4.0 mm. For example, the permeability of the blood–brain barrier (BBB) to K+ is low, and a bolus injection of K+ injected into blood does not change extracellular K+ concentrations in the cortex (Hansen et al. 1977). Astrocytes were identified more than 50 years ago as the primary cell type responsible for K+ buffering. Work by the Leif Hertz group (Hertz 1965) showed that astrocytes are specialized for rapid uptake of K+. Later, Kuffler showed that astrocytes are highly K+ permeable (Orkand et al. 1966). These observations, as well as other more recent studies, have led to the concept that astrocytes are the cell type chiefly responsible for buffering the increases in K+ that occur during neural firing. Some controversies have existed with regard to how astrocytes actually buffer excess K+. Multiple pathways, including Kir4.1, NKCCl, and the Na+, K+ ATPase, were originally implicated in K+ buffering (Walz 2000; D’Ambrosio et al. 2002), but later studies have refined the concepts by showing that increases in extracellular K+ are primarily eliminated by astrocytic K+ uptake via the Na+, K+ ATPase (Wallraff et al. 2006; Seifert et al. 2009) . Kir4.1 is important for setting the resting membrane potential, but appears to play a minor role, if any, in buffering local increases in K+ (Chever et al. 2010). The NKCCl cotransporter is expressed at low levels by astrocytes in the adult rodent brain and is therefore unlikely to be implicated in K+ buffering (Larsen et al. 2014). Thus, astrocytes are chiefly responsible for K+ buffering via the Na+, K+ ATPase, and, thereby, are similarly responsible for stabilizing neural membrane potential (Larsen et al. 2014)
CA2+-DEPENDENT K+ UPTAKE
In addition to uptake of excess K+, recent work shows that astrocytes also are engaged in active control of resting extracellular concentrations of K+. Analyses in vitro, in hippocampal slices, and in vivo show that astrocytic Ca2+ signaling leads to the rapid uptake of K+ and a transient lowering of extracellular K+ (Wang et al. 2012a). Pharmacological analysis indicates that Ca2+-mediated K+ uptake is driven by the Na+, K+ ATPase and is dependent on the influx of Na+ facilitated by the Na+, Ca2+ exchanger (NXC) (Wang et al. 2012a). The intracellular concentration of Na+ in many cells, including astrocytes, is rate limiting for the activity of the Na+, K+ ATPase (Pellerin and Magistretti 1997; Lupfert et al. 2001). Thus, increases in Na+ activate the Na+, K+ ATPase and, consequently, lower the levels of extracellular K+. Ca2+-regulated K+ uptake has been shown to decrease the frequency of EPSPs at baseline in a time frame concomitant with a reduction in the rate of synaptic failures (Wang et al. 2012a). The net result is that astrocytes via active uptake of K+ reduce baseline EPSP activity (noise), while enhancing the precision of signal transmission. In other words, astrocytic Ca2+ signaling increases the signal-to-noise ratio of neural processing. Ca2+-mediated K+ uptake endows astrocytes with a simple yet powerful tool for rapid and widespread manipulation of neural networks (Wang et al. 2012a). However, similar to gliotransmitter release, active K+ uptake is transient and extracellular K+ returns to baseline levels within ~60 sec.
LONG-TERM CHANGES IN NEURAL NETWORKS AND THE ROLE OF ASTROCYTES
Other lines of work have assessed the role of astrocytes in longer-lasting plasticity or more specifically in hippocampal long-term potentiation (LTP). Perhaps the most contentious area has surrounded the influence of astrocytes in the modulation of LTP in brain slice preparations. At this time, it is not clear why different results have been obtained; however, possibilities include different stimulus conditions, temperature, and age of preparations. Rather than debating pros and cons of each study, we simply report the results of a few. In most of these studies, LTP was triggered by high-frequency stimulation (HFS), and the effects of genetic or pharmacological manipulation of astrocytes assessed in parallel. In the first study of astrocytes in LTP, dominant-negative inhibition of vesicular release (soluble NSF attachment protein receptors [SNARE]) under the astrocyte-specific promoter, glial fibrillary acidic protein (GFAP), lowered baseline adenosine concentration (Pascual et al. 2005), which leads to a long-term change in postsynaptic NMDAR trafficking (Clasadonte et al. 2013), and, as a consequence, leads to a change in modulation of the magnitude of NMDAR-dependent LTP. In another study, d-serine release from astrocytes was shown to be critical for NMDAR-dependent LTP (Henneberger et al. 2010). Another group showed that engraftment of human astrocytes lowered the threshold for induction of LTP in the murine hippocampus by increasing AMPA receptor recruitment in response to HFS. This effect was shown to be mediated, at least in part, by release of tumor necrosis factor (TNF)-α (Han et al. 2013). Glia-derived TNF-α drives insertion of AMPA receptors in the postsynaptic membrane (Stellwagen and Malenka 2006). Combined, these studies show that astrocytic-derived neuromodulators, including adenosine, d-serine, or TNF-α alter neuronal responsiveness to HFS, resulting in long-lasting changes in neural excitability. It is worth noting that neither the control of adenosine nor TNF-α reports included astrocytic Ca2+ signaling as a key step in long-term neural plasticity. In contrast, significant evidence supports a role for astrocytic Ca2+ signaling in the regulation of extracellular d-serine. These studies raise the interesting potential that there is one or more Ca2+-independent glial pathway that regulates neuroglial coupling and deserves further attention. This is a consideration we return to later when we discuss time of day and vigilance state-dependent regulation of gliotransmission.
Thus, looking back on reports published over the last several decades, it is clear that single episodes of astrocytic Ca2+ signaling exert a transient effect on synaptic transmission and that more sustained effects, either mediated via modulation of receptor trafficking or excitability, may act independent of Ca2+ excitability. This conclusion is supported by other lines of work showing that astrocytes are instrumental for synapse formation, maintenance, and elimination (Ullian et al. 2004; Allen and Barres 2005; Chung and Barres 2012).
IN VIVO STUDIES
To develop a framework for how astrocytes participate in complex neural processes, it is necessary to include in vivo studies. Does the analysis of live animals contain the information that will decipher how astrocytes contribute to neural function beyond their metabolic and homeostatic functions? Maybe. Several laboratories have studied astrocytic Ca2+ signaling in anesthetized or awake mice after loading the exposed cortex with fluorescent Ca2+ indicators. The most popular approaches have been to characterize astrocytic Ca2+ signaling evoked by sensory input, including whisker (Wang et al. 2006), visual (Schummers et al. 2008), or olfactory stimulation (Gurden et al. 2006). Other groups have studied astrocytic Ca2+ signaling during locomotion in awake animals (Dombeck et al. 2007; Hoogland et al. 2009; Nimmerjahn et al. 2009). So what have they found? Two main findings have so far emerged from these studies: (1) Astrocytic Ca2+ signals are coordinated and displayed as widespread synchronous Ca2+ increases that engage essentially all astrocytes within the field of view (typically 20–80 cells across a 200- to 600-µm-wide field). Widespread synchronized Ca2+ increases are a consistent finding across many laboratories in in vivo preparations, but surprisingly, widespread synchronized Ca2+ signaling has never been observed in slices. Pathological conditions, such as acute ammonia toxicity, lead to a disappearance of global astrocytic Ca2+ increases, which are replaced with oscillatory increases of Ca2+ in single astrocytes (Rangroo Thrane et al. 2013). Similar observations have been made in more chronic disease states, such as mouse models of Alzheimer’s disease in which astrocytes display abnormal oscillatory increases in Ca2+ (Takano et al. 2007; Kuchibhotla et al. 2009). (2) Astrocytes respond slowly to sensory stimulation with Ca2+ increases that first peak 3–10 sec after initiating the stimulation (Wang et al. 2009). The slow engagement of astrocytes in response to sensory stimulation is also a general phenomenon observed across different laboratories and corresponding to the slow speed at which astrocytic Ca2+ signaling is triggered in acute slices. A recent study based on a clever analysis of staggered imaging processing found that astrocytes also may display very fast Ca2+ transients, which peak within milliseconds after whisker stimulation (Lind et al. 2013). This observation is interesting but awaits confirmation from other groups. Notably, anesthesia has been shown to potently dampen the spontaneous widespread astrocytic Ca2+ signal, suggesting that mediators of wakefulness, including catecholamines, may play a role in spontaneous astrocytic Ca2+ signaling (Thrane et al. 2012).
Of note, the original in vivo studies attributed neuroglia Ca2+ signaling to activation of astrocytic metabotropic glutamate (mGlu) receptors by synaptic release of glutamate (Gurden et al. 2006; Wang et al. 2006; Schummers et al. 2008); glutamate acting as a mediator of neuroglia signaling has later been challenged by the finding that adult cortical and hippocampal astrocytes have significantly reduced expression of Gq-linked mGlu receptors and that mGlu receptor agonists only trigger astrocytic Ca2+ signaling during early development stages (before 3 wk of age) (Sun et al. 2013). Because mGlu receptors are expressed by excitatory neurons, it is possible that the mGlu receptor agonists directly interfered with local transmission, but more studies are obviously needed. By comparing in vivo studies to observation in slices, it is clear that astrocytic Ca2+ signaling in intact adult brain and in acute slice preparations from young pups differ fundamentally with regard to both spatial extension and mode of initiation.
NEUROMODULATORS AS A KEY MEDIATOR OF ASTROCYTIC CA2+ SIGNALING IN AWAKE MICE
More recent observations point to the novel concept that both spontaneous and evoked astrocytic Ca2+ signaling are mediated by norepinephrine in response to locus coeruleus (LC) activation in awake mice. Direct stimulation of LC triggers coordinated and synchronous astrocytic Ca2+ signaling similar to spontaneous and evoked Ca2+ signaling (Bekar et al. 2008b; Ding et al. 2013). LC is the sole source of norepinephrine in forebrain. LC is located in pons, and LC neurons extend highly branched unmyelinated projections that form a large number of varicosities containing norepinephrine-containing vesicles (Levitt and Moore 1978). Less than 10% of these varicosities can be characterized as conventional synapses apposed to a postsynaptic membrane (Cohen et al. 1997). The remainder of these varicosities form open synapses in which norepinephrine is released broadly into the interstitial space. LC varicosities have been shown to be present in highest density close to blood vessels, but are also often apposed to axons, dendrites, and glial processes (Paspalas and Papadopoulos 1996; Cohen et al. 1997) The α1 receptor antagonist, prazosin, effectively suppresses both evoked and spontaneous astrocytic Ca2+ signaling (Ding et al. 2013). A recent beautiful study added additional support to the idea that α1 adrenergic receptors are primarily responsible for spontaneous astrocytic Ca2+ signaling in awake mice, and extended the concept by proposing that norepinephrine shifts the gain of astrocyte networks according to behavioral state, enabling astrocytes to respond to local changes in neuronal activity (Paukert et al. 2014). In retrospect, it is possible that prior reports on sensory-induced Ca2+ signaling, in fact, represented norepinephrine release in response to a salient stimulation. For example, arousal evoked by an unexpected sensory stimulation, such as sudden whisker stimulation, is associated with the concerted release of multiple neuromodulators. An analysis of the success rate and delay of whisker stimulation suggested that induced astrocytic Ca2+ signaling is indeed mediated by norepinephrine release (Ding et al. 2013). Moving from whisker stimulation to a classical startle response triggered by a puff of air to the eye and tail increased the success rate and variability, as well as reduced the delay of stimulation-induced astrocytic Ca2+ signaling. These observations support the idea that whisker-induced Ca2+ signaling is a response to arousal rather than to the local increase in excitatory transmission (Ding et al. 2013). The slow and more widespread responses of astrocytes fit well with such an action of neuromodulators (Bekar et al. 2008b).
DO NEUROMODULATORS ACT THROUGH ASTROCYTES TO EVOKE LONG-LASTING PLASTICITY OF NEURAL CIRCUITS?
In regard to this question, work performed in both slices and in vivo has shown that when combined with sensory glutamatergic input, cholinergic signaling can trigger long-lasting changes in synaptic strength (LTP). Findings from three independent laboratories show that mice in which astrocytes cannot mobilize cytosolic Ca2+ stores (IP3R2 knockout mice) failed to develop long-term enhancement of excitatory transmission in response to combined stimulation of cholinergic and sensory inputs. In these studies, sensory input (whisker [Takata et al. 2011], visual stimulation [Chen et al. 2012], or tail pinch [Navarrete et al. 2012]) in combination with stimulation of nucleus basalis or cholinergic fibers resulted in a sustained potentiation of excitatory transmission. Thus, emerging evidence suggests that neuromodulators may not only be responsible for spontaneous and evoked astrocytic Ca2+ signaling in awake mice, but also for long-term neural plasticity (Parpura and Verkhratsky 2012).
Astrocytes express Gq-linked, as well as Gi- and Gs-linked metabotropic receptors that are activated by norepinephrine, acetylcholine, serotonin, and likely other neuromodulators (Hertz et al. 2004; Verkhratsky et al. 2012). One of the characteristics of neuromodulators is that they typically target multiple cells via volume transmission and diffusion across considerable distances before receptor binding (Callado and Stamford 2000; Cragg et al. 2001). Another typical feature of neuromodulators is that they act in a slow time frame of seconds, typically affecting baseline neuronal activity rather than mediating specific responses (Izumi and Zorumski 1999; Huang et al. 2012). In fact, a rich literature suggests that neuromodulators are potent modulators of long-term synaptic plasticity and involved in both LTP and long-term depression (LTD) (Brocher et al. 1992; Otmakhova and Lisman 1996; Katsuki et al. 1997). This raises the question as to whether astrocytic Ca2+ is a key intermediary of the effect of neuromodulators. The widespread and slow mode of action certainly is shared between astrocytes and neuromodulators. The observation that anesthesia potently inhibits spontaneous astrocytic Ca2+ signaling (Thrane et al. 2012) is consistent with other observations that anesthesia potently suppresses the activity of both LC and nucleus basalis (Laalou et al. 2008; Bekar et al. 2012).
SLEEP, AROUSAL, AND VIGILANCE
The observation of widespread astrocytic Ca2+ signals in vivo together with the ability of norepinephrine to “gate” these glial Ca2+ signals raises the intriguing potential that the astrocyte could mediate long-term modulation of selected circuits to promote different behavioral states. This notion is supported by in vivo studies that used molecular genetics targeted to the astrocyte and showed that astrocytes modulate sleep homeostasis (Halassa et al. 2009; Blutstein and Haydon 2013). Sleep is controlled by at least two substantial processes: the circadian oscillator and the sleep homeostat. The process of sleep homeostasis regulates the daily pressure to sleep and homeostatic responses to sleep deprivation. When dominant-negative (dn)SNARE is expressed in astrocytes, under the control of the GFAP promoter, sleep homeostasis is impaired. Pharmacological and molecular genetic studies have shown that this is mediated by adenosine, which accumulates from an astrocytic source during wakefulness that acts through neuronal A1R to mediate these effects. Whole-cell pyramidal neuron recordings in vivo as well as local field potential recordings showed that this glial manipulation leads to a sustained change in the cortical slow oscillations (up and down states) that underlie slow wave activity of nonrapid eye movement (NREM) sleep.
These slow oscillations critically depend on the expression of neuronal NMDARs. Because astrocytic adenosine is known to modulate neuronal NMDAR trafficking and, consequently, synaptic NMDAR-mediated currents, this pathway provides the opportunity for the astrocyte to cause a sustained modulation of a neuronal circuit that underlies behavioral states—sleep and wakefulness. One caution regarding use of the GFAP-driven dnSNARE expression is that the selective expression by astrocytes versus other cell types has not been proven beyond the coexpression of enhanced green fluorescent protein (EGFP) and LacZ reporter genes. Because the genes for dnSNARE, LacZ, and EGFP were injected as independent genes when the transgenic mouse was generated, a valid critique might be that neither LacZ nor EGFP necessarily reflect dnSNARE expression (Pascual et al. 2005). However, because the expression of dnSNARE was directly monitored in mixed cultures and only found to be present in astrocytes, such a concern is mitigated.
These studies prompted us to ask whether there might be time-of-day differences in the “state” of a brain slice given the importance of the astrocyte in the control of sleep homeostasis. To address this question, slices were isolated from mice at 4-h intervals during the 24-h day as well as from mice that had been sleep deprived. Astrocyte-derived adenosine was assessed using biosensors, as well as pharmacological enhancement of fEPSPs (brain slice) and local field potentials (in vivo) in response to the A1R antagonist CPT (Schmitt et al. 2012). Results of each of these three approaches were qualitatively in accordance with one another. Surprisingly, we observed differences in extracellular adenosine levels in accordance with the vigilance state of the animal. For example, if animals were euthanized and slices cut at the end of the dark phase (subjective daytime), then adenosine was elevated, whereas if slices were obtained during the light phase (subjective nighttime), adenosine levels were reduced. Moreover, this effect was mediated by the behavioral state because, when mice were sleep-deprived before isolating slices, we found that adenosine was elevated in comparison to the level recorded in slices prepared at the same time from undisturbed mice.
These studies provide several insights and raise many questions about the astrocyte and brain state. First, it becomes extremely important to document animal state and time of day that slices are obtained to allow comparisons of data between laboratories. Second, most animal facilities are maintained on a light cycle that corresponds with the animal technician’s light cycle. However, because mice are nocturnal, this means we are often studying mice when they would normally be sleeping. Third, many of us study processes, such as plasticity and learning and memory, yet we are taking samples at a time when the brain (and the astrocyte) is in sleep mode. Do the data correspond to what would be obtained if studied during the dark phase? Are we missing astrocyte-mediated processes by studying brain samples during the light phase?
An additional question that arises is how does the astrocyte and a brain slice have a memory of the time of day and state (e.g., sleep derived) that the animal was euthanized? Presumably, there is little neural activity in a brain slice that could be driving different amounts of glial-derived adenosine. Could there be sustained behavioral state-dependent changes in astrocytic Ca2+ signals that are maintained in the dish? We doubt it. We are left to ponder the possibility, as discussed earlier, that there are many Ca2+-independent signals that regulate the astrocytic compartment that have gone unexplored to date, which might account for these differences. For example, the recently described glymphatic system—a macroscopic clearance system—is primarily active in the sleeping animals (Xie et al. 2013). Does astrocytic Ca2+ signaling suppress glymphatic activity? Indirect evidence supports that idea. Astrocytic Ca2+ signaling is primarily induced by activation of astrocytic α1 adrenergic receptors in awake-behaving mice (Ding et al. 2013). Because antagonists of adrenergic receptors inhibit astrocytic Ca2+ signaling (Ding et al, 2013) and enhance glymphatic activity in awake mice (Xie et al. 2013), it is possible that astrocytic Ca2+ signaling regulates the water and solute transport that drives glymphatic exchange of cerebrospinal fluid (CSF) and interstitial fluid. Additional studies are needed to prove this point, but it is clear that astrocytes depend heavily on the water channel AQP4, because global or conditional deletion of AQP4 reduces glymphatic activity by ~60% (Iliff et al. 2012).
IS IT POSSIBLE TO PUT IT ALL TOGETHER?
Taking a step back, one may conclude that analysis of neuroglia signaling in slice preparation in many ways does not reflect in vivo observations. Slices have traditionally been prepared from young rodent brains in which neither astrocytic nor neuromodulator signaling are mature (Takano et al. 2014). This has been a necessity because fluorescent Ca2+ indicators cannot be loaded in slices prepared from older animals (Nedergaard and Verkhratsky 2012). Approaches to stimulate astrocytes (or neurons) in slices also differ fundamentally from those used to assess operational transmission of sensory input in the intact brain. High-frequency stimulation triggers artificial ATP release (Bekar et al. 2008a), and uncaging of caged Ca2+ does not mimic receptor-mediated events and has fundamentally different impacts on neural activity (Wang et al. 2013). Because astrocytes do not express any receptors that are not expressed by neurons or other cell types in the CNS, it has not been possible to use a selective agonist to activate Ca2+ signaling without affecting other cell types, including neurons. The use of transgenic mice expressing Mrg1A receptors, which are normally not expressed in the forebrain, has been criticized because it has not been established whether the Mrg1A receptors can activate physiologically relevant intracellular signaling pathways (Agulhon et al. 2010). Another problem with slices is that astrocytes almost immediately undergo a classical sign of reactive gliosis with expression of the developmental marker nestin, increases in GFAP, and mislocation of AQP4 (Takano et al. 2014). Astrocytes in the surface area of slices also rapidly lose their fine perisynaptic processes and may not support synaptic function optimally (Takano et al. 2014). In vivo preparations are also subjected to artifacts, including reduced capillary perfusion, anesthesia, microglial cell activation, and more (Xu et al. 2007).
All of these caveats might give the impression that it will be impossible to experimentally decode the roles of astrocytes in brain function. It is not our intention to cast a shadow of despair by making these comments; rather, we wish to point out some of the areas of caution that need to be exerted in interpreting past studies and in the design of future experiments. What is clear is that given the sensitivity of the astrocyte to age, time of day, anesthesia, and injury, great caution must be taken to design interpretable studies. To assist in this regard, considerable emphasis on the development of new astrocyte-specific molecular genetic manipulations is needed so that, when possible, observations and manipulations can be performed in intact, adult specimens. Toward this goal, genetically encoded Ca2+ indicators, such as GCam3–6, are expected to revolutionize our view on astrocytic involvement in cortical function. Additionally, mice expressing indicators of glucose and second messenger pathways beyond IP3/Ca2+ will become invaluable. For example, astrocytes express high levels of mGluR3, but we do not understand the functional consequences of activation of this Gi-coupled receptor. Current in vivo imaging studies have been focused on observing Ca2+ signals in response to sensory stimuli. Perhaps there are dramatic changes in intracellular cyclic adenosine monophosphate (cAMP) dynamics caused by glutamatergic activation of astrocytic mGluR3.
In addition to the development of models based on existing concepts and knowledge, a new opportunity awaits us in which we can use the human genetics and genome-wide association studies (GWAS) combined with existing mouse astrocyte gene expression databases to identify new targets for experimental intervention. For example, in a recent human autopsy study of patients suffering from major depressive and bipolar disorder, G protein–coupled receptors were identified in which expression was changed in these patients. By referencing the mouse astrocyte databases, these receptors are highly enriched in astrocytes. For example, the orphan GPCR GPRC5B is highly enriched in astrocytes and is down-regulated in tissue from patients with major depressive disorder (MDD) and up-regulated in patients with bipolar disorder (Tomita et al. 2013). A GPRC5 knockout mouse has been generated and shows significant behavioral deficits (Sano et al. 2011). Whether such deficits arise from alterations in astrocytic signaling remains to be determined, but it will be intriguing to unravel given the potential links between this receptor and psychiatric disorders.
As we begin to decipher astroglial function, it will be important to turn to disorders of the nervous system to ask when and how the astrocyte contributes to the many societal burdens of psychiatric and neurological disorders of the brain. Although it is difficult to predict which of these disorders will have as a primary etiology an astroglial malfunction, it is of immediate interest to examine disorders with a sleep comorbidity. Studies from both of our laboratories have highlighted the importance of the astrocyte in sleep-related processes. On the one hand, the astrocyte modulates sleep homeostasis, and, on the other hand, this glial cell plays an important role in the clearance of amyloid β from the brain during sleep. Could disorders in the astrocyte contribute to disorders, such as Alzheimer’s disease and sleep comorbidities? As discussed in our concerns about the study of the astrocyte is the observation that astrocytes can become reactive extremely quickly. The term “reactive” was coined to express the notion that the astrocytic change is secondary to a primary pathology in neuronal function. However, this may not necessarily be the case. In epilepsy, astrocytes become reactive and lose the expression of glutamine synthetase, a key enzyme responsible for the conversation of glutamate to glutamine and the subsequent supply of a GABA precursor. Using viral strategies, it has been possible to induce gliosis in astrocytes. Murine-transduced astrocytes lose glutamine synthetase expression, like their human counterparts in medial temporal lobe epilepsy, which subsequently leads to a substantial deficit in GABAergic inhibition. As a consequence, preparations are hyperexcitable (Ortinski et al. 2010). Although this does not mean that epilepsy is caused by reactive astrocytes, it points to the potential for primary dysfunctions in astrocyte signaling to lead to changes in neuronal and consequently brain function.
Sleep disorders are comorbid with numerous disorders of the brain including depression, schizophrenia, Alzheimer’s, and Parkinson’s disease, to name a few. Additionally, sleep deprivation can lead to a reduction in the threshold for seizures. Given the importance of the astrocyte in modulating sleep-related processes, there is an exciting period ahead in which the introduction of newly refined molecular genetic approaches, together with animal models of diseases, will offer new insights into the roles of astrocytes in brain dysfunction.
CONCLUSION
Over the past 20 years, we have come a long way since the initial discoveries that astrocytic Ca2+ signals can impact neurons. During this period, members of the field have not always agreed about experimental design and interpretation, but what is clear is that we have made tremendous strides toward appreciating important roles for these dynamic glial cells in brain function. To be poised after only 20 years to make insights into how the astrocyte contributes to brain dysfunction is exciting and has the potential to offer new insights that might be translated into new treatments for at least some of these disorders.
ACKNOWLEDGMENTS
The authors are grateful to Takahiro Takano for assistance in generating the graphic used in Figure 1.
Footnotes
Editors: Ben A. Barres, Marc R. Freeman, and Beth Stevens
Additional Perspectives on Glia available at www.cshperspectives.org
REFERENCES
- Agulhon C, Fiacco TA, McCarthy KD 2010. Hippocampal short- and long-term plasticity are not modulated by astrocyte Ca2+ signaling. Science 327: 1250–1254. [Abstract] [Google Scholar]
- Allen NJ, Barres BA 2005. Signaling between glia and neurons: Focus on synaptic plasticity. Curr Opin Neurobiol 15: 542–548. [Abstract] [Google Scholar]
- Araque A, Sanzgiri RP, Parpura V, Haydon PG 1998. Calcium elevation in astrocytes causes an NMDA receptor-dependent increase in the frequency of miniature synaptic currents in cultured hippocampal neurons. J Neurosci 18: 6822–6829. [Abstract] [Google Scholar]
- Araque A, Parpura V, Sanzgiri RP, Haydon PG 1999. Tripartite synapses: Glia, the unacknowledged partner. Trends Neurosci 22: 208–215. [Abstract] [Google Scholar]
- Bekar L, Libionka W, Tian GF, Xu Q, Torres A, Wang X, Lovatt D, Williams E, Takano T, Schnermann J, et al. 2008a. Adenosine is crucial for deep brain stimulation-mediated attenuation of tremor. Nat Med 14: 75–80. [Abstract] [Google Scholar]
- Bekar LK, He W, Nedergaard M 2008b. Locus coeruleus α-adrenergic-mediated activation of cortical astrocytes in vivo. Cereb Cortex 18: 2789–2795. [Abstract] [Google Scholar]
- Bekar LK, Wei HS, Nedergaard M 2012. The locus coeruleus-norepinephrine network optimizes coupling of cerebral blood volume with oxygen demand. J Cereb Blood Flow Metab 32: 2135–2145. [Europe PMC free article] [Abstract] [Google Scholar]
- Blutstein T, Haydon PG 2013. The importance of astrocyte-derived purines in the modulation of sleep. Glia 61: 129–139. [Europe PMC free article] [Abstract] [Google Scholar]
- Brocher S, Artola A, Singer W 1992. Agonists of cholinergic and noradrenergic receptors facilitate synergistically the induction of long-term potentiation in slices of rat visual cortex. Brain Res 573: 27–36. [Abstract] [Google Scholar]
- Callado LF, Stamford JA 2000. Spatiotemporal interaction of α2 autoreceptors and noradrenaline transporters in the rat locus coeruleus: Implications for volume transmission. J Neurochem 74: 2350–2358. [Abstract] [Google Scholar]
- Chen N, Sugihara H, Sharma J, Perea G, Petravicz J, Le C, Sur M 2012. Nucleus basalis-enabled stimulus-specific plasticity in the visual cortex is mediated by astrocytes. Proc Natl Acad Sci 109: E2832–E2841. [Europe PMC free article] [Abstract] [Google Scholar]
- Chever O, Djukic B, McCarthy KD, Amzica F 2010. Implication of Kir4.1 channel in excess potassium clearance: An in vivo study on anesthetized glial-conditional Kir4.1 knock-out mice. J Neurosci 30: 15769–15777. [Abstract] [Google Scholar]
- Chung WS, Barres BA 2012. The role of glial cells in synapse elimination. Curr Opin Neurobiol 22: 438–445. [Europe PMC free article] [Abstract] [Google Scholar]
- Clasadonte J, Dong J, Hines DJ, Haydon PG 2013. Astrocyte control of synaptic NMDA receptors contributes to the progressive development of temporal lobe epilepsy. Proc Natl Acad Sci 110: 17540–17545. [Europe PMC free article] [Abstract] [Google Scholar]
- Cohen Z, Molinatti G, Hamel E 1997. Astroglial and vascular interactions of noradrenaline terminals in the rat cerebral cortex. J Cereb Blood Flow Metab 17: 894–904. [Abstract] [Google Scholar]
- Cornell-Bell AH, Thomas PG, Smith SJ 1990. The excitatory neurotransmitter glutamate causes filopodia formation in cultured hippocampal astrocytes. Glia 3: 322–334. [Abstract] [Google Scholar]
- Cragg SJ, Nicholson C, Kume-Kick J, Tao L, Rice ME 2001. Dopamine-mediated volume transmission in midbrain is regulated by distinct extracellular geometry and uptake. J Neurophysiol 85: 1761–1771. [Abstract] [Google Scholar]
- D’Ambrosio R, Gordon DS, Winn HR 2002. Differential role of KIR channel and Na+/K+-pump in the regulation of extracellular K+ in rat hippocampus. J Neurophysiol 87: 87–102. [Abstract] [Google Scholar]
- Ding F, O’Donnell J, Thrane AS, Zeppenfeld D, Kang H, Xie L, Wang F, Nedergaard M 2013. α1-Adrenergic receptors mediate coordinated Ca2+ signaling of cortical astrocytes in awake, behaving mice. Cell Calcium 54: 387–394. [Europe PMC free article] [Abstract] [Google Scholar]
- Dombeck DA, Khabbaz AN, Collman F, Adelman TL, Tank DW 2007. Imaging large-scale neural activity with cellular resolution in awake, mobile mice. Neuron 56: 43–57. [Europe PMC free article] [Abstract] [Google Scholar]
- Fellin T, Pascual O, Gobbo S, Pozzan T, Haydon PG, Carmignoto G 2004. Neuronal synchrony mediated by astrocytic glutamate through activation of extrasynaptic NMDA receptors. Neuron 43: 729–743. [Abstract] [Google Scholar]
- Fiacco TA, McCarthy KD 2004. Intracellular astrocyte calcium waves in situ increase the frequency of spontaneous AMPA receptor currents in CA1 pyramidal neurons. J Neurosci 24: 722–732. [Abstract] [Google Scholar]
- Gurden H, Uchida N, Mainen ZF 2006. Sensory-evoked intrinsic optical signals in the olfactory bulb are coupled to glutamate release and uptake. Neuron 52: 335–345. [Abstract] [Google Scholar]
- Halassa MM, Florian C, Fellin T, Munoz JR, Lee SY, Abel T, Haydon PG, Frank MG 2009. Astrocytic modulation of sleep homeostasis and cognitive consequences of sleep loss. Neuron 61: 213–219. [Europe PMC free article] [Abstract] [Google Scholar]
- Han X, Chen M, Wang F, Windrem M, Wang S, Shanz S, Xu Q, Oberheim NA, Bekar L, Betstadt S, et al. 2013. Forebrain engraftment by human glial progenitor cells enhances synaptic plasticity and learning in adult mice. Cell Stem Cell 12: 342–353. [Europe PMC free article] [Abstract] [Google Scholar]
- Hansen AJ, Lund-Andersen H, Crone C 1977. K+-permeability of the blood–brain barrier, investigated by aid of a K+-sensitive microelectrode. Acta Physiol Scand 101: 438–445. [Abstract] [Google Scholar]
- Henneberger C, Papouin T, Oliet SH, Rusakov DA 2010. Long-term potentiation depends on release of d-serine from astrocytes. Nature 463: 232–236. [Europe PMC free article] [Abstract] [Google Scholar]
- Hertz L 1965. Possible role of neuroglia: A potassium-mediated neuronal–neuroglial–neuronal impulse transmission system. Nature 206: 1091–1094. [Abstract] [Google Scholar]
- Hertz L, Chen Y, Gibbs ME, Zang P, Peng L 2004. Astrocytic adrenoceptors: A major drug target in neurological and psychiatric disorders? Curr Drug Targets CNS Neurol Disord 3: 239–267. [Abstract] [Google Scholar]
- Hoogland TM, Kuhn B, Gobel W, Huang W, Nakai J, Helmchen F, Flint J, Wang SS 2009. Radially expanding transglial calcium waves in the intact cerebellum. Proc Natl Acad Sci 106: 3496–3501. [Europe PMC free article] [Abstract] [Google Scholar]
- Hounsgaard J, Nicholson C 1983. Potassium accumulation around individual purkinje cells in cerebellar slices from the guinea-pig. J Physiol 340: 359–388. [Abstract] [Google Scholar]
- Huang S, Trevino M, He K, Ardiles A, Pasquale R, Guo Y, Palacios A, Huganir R, Kirkwood A 2012. Pull-push neuromodulation of LTP and LTD enables bidirectional experience-induced synaptic scaling in visual cortex. Neuron 73: 497–510. [Europe PMC free article] [Abstract] [Google Scholar]
- Iliff JJ, Wang M, Liao Y, Plogg BA, Peng W, Gundersen GA, Benveniste H, Vates GE, Deane R, Goldman SA, et al. 2012. A paravascular pathway facilitates CSF flow through the brain parenchyma and the clearance of interstitial solutes, including amyloid β. Sci Transl Med 4: 147ra111. [Europe PMC free article] [Abstract] [Google Scholar]
- Izumi Y, Zorumski CF 1999. Norepinephrine promotes long-term potentiation in the adult rat hippocampus in vitro. Synapse 31: 196–202. [Abstract] [Google Scholar]
- Jourdain P, Bergersen LH, Bhaukaurally K, Bezzi P, Santello M, Domercq M, Matute C, Tonello F, Gundersen V, Volterra A 2007. Glutamate exocytosis from astrocytes controls synaptic strength. Nat Neurosci 10: 331–339. [Abstract] [Google Scholar]
- Kang J, Jiang L, Goldman SA, Nedergaard M 1998. Astrocyte-mediated potentiation of inhibitory synaptic transmission. Nat Neurosci 1: 683–692. [Abstract] [Google Scholar]
- Katsuki H, Izumi Y, Zorumski CF 1997. Noradrenergic regulation of synaptic plasticity in the hippocampal CA1 region. J Neurophysiol 77: 3013–3020. [Abstract] [Google Scholar]
- Kuchibhotla KV, Lattarulo CR, Hyman BT, Bacskai BJ 2009. Synchronous hyperactivity and intercellular calcium waves in astrocytes in Alzheimer mice. Science 323: 1211–1215. [Europe PMC free article] [Abstract] [Google Scholar]
- Laalou FZ, de Vasconcelos AP, Oberling P, Jeltsch H, Cassel JC, Pain L 2008. Involvement of the basal cholinergic forebrain in the mediation of general (propofol) anesthesia. Anesthesiology 108: 888–896. [Abstract] [Google Scholar]
- Larsen BR, Assentoft M, Cotrina ML, Hua SZ, Nedergaard M, Kaila K, Voipio J, Macaulay N 2014. Contributions of the Na+/K+ -ATPase, NKCC1, and Kir4.1 to hippocampal K+ clearance and volume responses. Glia 62: 608–622. [Europe PMC free article] [Abstract] [Google Scholar]
- Levitt P, Moore RY 1978. Noradrenaline neuron innervation of the neocortex in the rat. Brain Res 139: 219–231. [Abstract] [Google Scholar]
- Lind BL, Brazhe AR, Jessen SB, Tan FC, Lauritzen MJ 2013. Rapid stimulus-evoked astrocyte Ca2+ elevations and hemodynamic responses in mouse somatosensory cortex in vivo. Proc Natl Acad Sci 110: E4678–E4687. [Europe PMC free article] [Abstract] [Google Scholar]
- Lupfert C, Grell E, Pintschovius V, Apell HJ, Cornelius F, Clarke RJ 2001. Rate limitation of the Na+, K+-ATPase pump cycle. Biophys J 81: 2069–2081. [Europe PMC free article] [Abstract] [Google Scholar]
- Navarrete M, Araque A 2008. Endocannabinoids mediate neuron–astrocyte communication. Neuron 57: 883–893. [Abstract] [Google Scholar]
- Navarrete M, Perea G, Fernandez de Sevilla D, Gomez-Gonzalo M, Nunez A, Martin ED, Araque A 2012. Astrocytes mediate in vivo cholinergic-induced synaptic plasticity. PLoS Biol 10: e1001259. [Europe PMC free article] [Abstract] [Google Scholar]
- Nedergaard M 1994. Direct signaling from astrocytes to neurons in cultures of mammalian brain cells. Science 263: 1768–1771. [Abstract] [Google Scholar]
- Nedergaard M, Verkhratsky A 2012. Artifact versus reality—How astrocytes contribute to synaptic events. Glia 60: 1013–1023. [Europe PMC free article] [Abstract] [Google Scholar]
- Nedergaard M, Ransom B, Goldman SA 2003. New roles for astrocytes: Redefining the functional architecture of the brain. Trends Neurosci 26: 523–530. [Abstract] [Google Scholar]
- Newman EA, Zahs KR 1998. Modulation of neuronal activity by glial cells in the retina. J Neurosci 18: 4022–4028. [Europe PMC free article] [Abstract] [Google Scholar]
- Nimmerjahn A, Mukamel EA, Schnitzer MJ 2009. Motor behavior activates Bergmann glial networks. Neuron 62: 400–412. [Europe PMC free article] [Abstract] [Google Scholar]
- Orkand RK, Nicholls JG, Kuffler SW 1966. Effect of nerve impulses on the membrane potential of glial cells in the central nervous system of amphibia. J Neurophysiol 29: 788–806. [Abstract] [Google Scholar]
- Ortinski PI, Dong J, Mungenast A, Yue C, Takano H, Watson DJ, Haydon PG, Coulter DA 2010. Selective induction of astrocytic gliosis generates deficits in neuronal inhibition. Nat Neurosci 13: 584–591. [Europe PMC free article] [Abstract] [Google Scholar]
- Otmakhova NA, Lisman JE 1996. D1/D5 dopamine receptor activation increases the magnitude of early long-term potentiation at CA1 hippocampal synapses. J Neurosci 16: 7478–7486. [Abstract] [Google Scholar]
- Parpura V, Verkhratsky A 2012. Neuroglia at the crossroads of homoeostasis, metabolism and signalling: Evolution of the concept. ASN Neuro 4: 201–205. [Europe PMC free article] [Abstract] [Google Scholar]
- Parpura V, Basarsky TA, Liu F, Jeftinija K, Jeftinija S, Haydon PG 1994. Glutamate-mediated astrocyte-neuron signalling. Nature 369: 744–747. [Abstract] [Google Scholar]
- Pascual O, Casper KB, Kubera C, Zhang J, Revilla-Sanchez R, Sul JY, Takano H, Moss SJ, McCarthy K, Haydon PG 2005. Astrocytic purinergic signaling coordinates synaptic networks. Science 310: 113–116. [Abstract] [Google Scholar]
- Paspalas CD, Papadopoulos GC 1996. Ultrastructural relationships between noradrenergic nerve fibers and non-neuronal elements in the rat cerebral cortex. Glia 17: 133–146. [Abstract] [Google Scholar]
- Paukert M, Agarwal A, Cha J, Doze VA, Kang JU, Bergles DE 2014. Norepinephrine controls astroglial responsiveness to local circuit activity. Neuron 82: 1263–1270. [Europe PMC free article] [Abstract] [Google Scholar]
- Pellerin L, Magistretti PJ 1997. Glutamate uptake stimulates Na+, K+-ATPase activity in astrocytes via activation of a distinct subunit highly sensitive to ouabain. J Neurochem 69: 2132–2137. [Abstract] [Google Scholar]
- Perea G, Araque A 2007. Astrocytes potentiate transmitter release at single hippocampal synapses. Science 317: 1083–1086. [Abstract] [Google Scholar]
- Poskanzer KE, Yuste R 2011. Astrocytic regulation of cortical UP states. Proc Natl Acad Sci 108: 18453–18458. [Europe PMC free article] [Abstract] [Google Scholar]
- Rangroo Thrane V, Thrane AS, Wang F, Cotrina ML, Smith NA, Chen M, Xu Q, Kang N, Fujita T, Nagelhus EA, et al. 2013. Ammonia triggers neuronal disinhibition and seizures by impairing astrocyte potassium buffering. Nat Med 19: 1643–1648. [Europe PMC free article] [Abstract] [Google Scholar]
- Robitaille R 1998. Modulation of synaptic efficacy and synaptic depression by glial cells at the frog neuromuscular junction. Neuron 21: 847–855. [Abstract] [Google Scholar]
- Sano T, Kim YJ, Oshima E, Shimizu C, Kiyonari H, Abe T, Higashi H, Yamada K, Hirabayashi Y 2011. Comparative characterization of GPRC5B and GPRC5CLacZ knockin mice; behavioral abnormalities in GPRC5B-deficient mice. Biochem Biophys Res Commun 412: 460–465. [Abstract] [Google Scholar]
- Schmitt LI, Sims RE, Dale N, Haydon PG 2012. Wakefulness affects synaptic and network activity by increasing extracellular astrocyte-derived adenosine. J Neurosci 32: 4417–4425. [Europe PMC free article] [Abstract] [Google Scholar]
- Schummers J, Yu H, Sur M 2008. Tuned responses of astrocytes and their influence on hemodynamic signals in the visual cortex. Science 320: 1638–1643. [Abstract] [Google Scholar]
- Seifert G, Huttmann K, Binder DK, Hartmann C, Wyczynski A, Neusch C, Steinhauser C 2009. Analysis of astroglial K+ channel expression in the developing hippocampus reveals a predominant role of the Kir4.1 subunit. J Neurosci 29: 7474–7488. [Abstract] [Google Scholar]
- Shigetomi E, Bushong EA, Haustein MD, Tong X, Jackson-Weaver O, Kracun S, Xu J, Sofroniew MV, Ellisman MH, Khakh BS 2013. Imaging calcium microdomains within entire astrocyte territories and endfeet with GCaMPs expressed using adeno-associated viruses. J Gen Physiol 141: 633–647. [Europe PMC free article] [Abstract] [Google Scholar]
- Stellwagen D, Malenka RC 2006. Synaptic scaling mediated by glial TNF-α. Nature 440: 1054–1059. [Abstract] [Google Scholar]
- Sun W, McConnell E, Pare JF, Xu Q, Chen M, Peng W, Lovatt D, Han X, Smith Y, Nedergaard M 2013. Glutamate-dependent neuroglial calcium signaling differs between young and adult brain. Science 339: 197–200. [Europe PMC free article] [Abstract] [Google Scholar]
- Takano T, Han X, Deane R, Zlokovic B, Nedergaard M 2007. Two-photon imaging of astrocytic Ca2+ signaling and the microvasculature in experimental mice models of Alzheimer’s disease. Ann NY Acad Sci 1097: 40–50. [Abstract] [Google Scholar]
- Takano T, He W, Han X, Wang F, Xu Q, Wang X, Oberheim Bush NA, Cruz N, Dienel GA, Nedergaard M 2014. Rapid manifestation of reactive astrogliosis in acute hippocampal brain slices. Glia 62: 78–95. [Europe PMC free article] [Abstract] [Google Scholar]
- Takata N, Mishima T, Hisatsune C, Nagai T, Ebisui E, Mikoshiba K, Hirase H 2011. Astrocyte calcium signaling transforms cholinergic modulation to cortical plasticity in vivo. J Neurosci 31: 18155–18165. [Abstract] [Google Scholar]
- Thrane AS, Rangroo Thrane V, Zeppenfeld D, Lou N, Xu Q, Nagelhus EA, Nedergaard M 2012. General anesthesia selectively disrupts astrocyte calcium signaling in the awake mouse cortex. Proc Natl Acad Sci 109: 18974–18979. [Europe PMC free article] [Abstract] [Google Scholar]
- Tomita H, Ziegler ME, Kim HB, Evans SJ, Choudary PV, Li JZ, Meng F, Dai M, Myers RM, Neal CR, et al. 2013. G protein-linked signaling pathways in bipolar and major depressive disorders. Front Genet 4: 297. [Europe PMC free article] [Abstract] [Google Scholar]
- Traynelis SF, Dingledine R 1988. Potassium-induced spontaneous electrographic seizures in the rat hippocampal slice. J Neurophysiol 59: 259–276. [Abstract] [Google Scholar]
- Ullian EM, Christopherson KS, Barres BA 2004. Role for glia in synaptogenesis. Glia 47: 209–216. [Abstract] [Google Scholar]
- Verkhratsky A, Rodriguez JJ, Parpura V 2012. Calcium signalling in astroglia. Mol Cell Endocrinol 353: 45–56. [Abstract] [Google Scholar]
- Wallraff A, Kohling R, Heinemann U, Theis M, Willecke K, Steinhauser C 2006. The impact of astrocytic gap junctional coupling on potassium buffering in the hippocampus. J Neurosci 26: 5438–5447. [Abstract] [Google Scholar]
- Walz W 2000. Role of astrocytes in the clearance of excess extracellular potassium. Neurochem Int 36: 291–300. [Abstract] [Google Scholar]
- Wang X, Lou N, Xu Q, Tian GF, Peng WG, Han X, Kang J, Takano T, Nedergaard M 2006. Astrocytic Ca2+ signaling evoked by sensory stimulation in vivo. Nat Neurosci 9: 816–823. [Abstract] [Google Scholar]
- Wang X, Takano T, Nedergaard M 2009. Astrocytic calcium signaling: Mechanism and implications for functional brain imaging. Methods Mol Biol 489: 93–109. [Europe PMC free article] [Abstract] [Google Scholar]
- Wang F, Smith NA, Xu Q, Fujita T, Baba A, Matsuda T, Takano T, Bekar L, Nedergaard M 2012a. Astrocytes modulate neural network activity by Ca2+-dependent uptake of extracellular K+. Sci Signal 5: ra26. [Europe PMC free article] [Abstract] [Google Scholar]
- Wang F, Xu Q, Wang W, Takano T, Nedergaard M 2012b. Bergmann glia modulate cerebellar Purkinje cell bistability via Ca2+-dependent K+ uptake. Proc Natl Acad Sci 109: 7911–7916. [Europe PMC free article] [Abstract] [Google Scholar]
- Wang F, Smith NA, Xu Q, Goldman S, Peng W, Huang JH, Takano T, Nedergaard M 2013. Photolysis of caged Ca2+ but not receptor-mediated Ca2+ signaling triggers astrocytic glutamate release. J Neurosci 33: 17404–17412. [Europe PMC free article] [Abstract] [Google Scholar]
- Xie L, Kang H, Xu Q, Chen MJ, Liao Y, Thiyagarajan M, O’Donnell J, Christensen DJ, Nicholson C, Iliff JJ, et al. 2013. Sleep drives metabolite clearance from the adult brain. Science 342: 373–377. [Europe PMC free article] [Abstract] [Google Scholar]
- Xu HT, Pan F, Yang G, Gan WB 2007. Choice of cranial window type for in vivo imaging affects dendritic spine turnover in the cortex. Nat Neurosci 10: 549–551. [Abstract] [Google Scholar]
Articles from Cold Spring Harbor Perspectives in Biology are provided here courtesy of Cold Spring Harbor Laboratory Press
Full text links
Read article at publisher's site: https://doi.org/10.1101/cshperspect.a020438
Read article for free, from open access legal sources, via Unpaywall:
http://cshperspectives.cshlp.org/content/7/3/a020438.full.pdf
Citations & impact
Impact metrics
Citations of article over time
Article citations
Astrocytic neuroligin 3 regulates social memory and synaptic plasticity through adenosine signaling in male mice.
Nat Commun, 15(1):8639, 05 Oct 2024
Cited by: 0 articles | PMID: 39366972 | PMCID: PMC11452673
A human proteogenomic-cellular framework identifies KIF5A as a modulator of astrocyte process integrity with relevance to ALS.
Commun Biol, 6(1):678, 29 Jun 2023
Cited by: 2 articles | PMID: 37386082 | PMCID: PMC10310856
Activation of Gq-Coupled Receptors in Astrocytes Restores Cognitive Function in Alzheimer's Disease Mice Model.
Int J Mol Sci, 24(12):9969, 09 Jun 2023
Cited by: 1 article | PMID: 37373117 | PMCID: PMC10298315
Decreased Electroencephalographic Alpha Power During Anesthesia Induction Is Associated With EEG Discontinuity in Human Infants.
Anesth Analg, 135(6):1207-1216, 16 Nov 2022
Cited by: 9 articles | PMID: 35041633 | PMCID: PMC9276847
Photobiomodulation Attenuated Cognitive Dysfunction and Neuroinflammation in a Prenatal Valproic Acid-Induced Autism Spectrum Disorder Mouse Model.
Int J Mol Sci, 23(24):16099, 17 Dec 2022
Cited by: 6 articles | PMID: 36555737 | PMCID: PMC9785820
Go to all (67) article citations
Similar Articles
To arrive at the top five similar articles we use a word-weighted algorithm to compare words from the Title and Abstract of each citation.
Is astrocyte calcium signaling relevant for synaptic plasticity?
Neuron Glia Biol, 6(3):147-155, 01 Aug 2010
Cited by: 23 articles | PMID: 21122195
Review
Astrocytes in Alzheimer's disease: emerging roles in calcium dysregulation and synaptic plasticity.
J Alzheimers Dis, 22(3):699-714, 01 Jan 2010
Cited by: 51 articles | PMID: 20847426
Review
Astrocyte calcium signaling: the third wave.
Nat Neurosci, 19(2):182-189, 01 Feb 2016
Cited by: 458 articles | PMID: 26814587
Review
Does Global Astrocytic Calcium Signaling Participate in Awake Brain State Transitions and Neuronal Circuit Function?
Neurochem Res, 42(6):1810-1822, 16 Feb 2017
Cited by: 21 articles | PMID: 28210958 | PMCID: PMC5451293
Review Free full text in Europe PMC
Funding
Funders who supported this work.
NIA NIH HHS (1)
Grant ID: R01 AG048769
NINDS NIH HHS (2)
Grant ID: R01 NS078304
Grant ID: R01 NS075177