Abstract
Free full text

Efficient neuroplasticity induction in chronic stroke patients by an associative brain-computer interface
Abstract
Brain-computer interfaces (BCIs) have the potential to improve functionality in chronic stoke patients when applied over a large number of sessions. Here we evaluated the effect and the underlying mechanisms of three BCI training sessions in a double-blind sham-controlled design. The applied BCI is based on Hebbian principles of associativity that hypothesize that neural assemblies activated in a correlated manner will strengthen synaptic connections. Twenty-two chronic stroke patients were divided into two training groups. Movement-related cortical potentials (MRCPs) were detected by electroencephalography during repetitions of foot dorsiflexion. Detection triggered a single electrical stimulation of the common peroneal nerve timed so that the resulting afferent volley arrived at the peak negative phase of the MRCP (BCIassociative group) or randomly (BCInonassociative group). Fugl-Meyer motor assessment (FM), 10-m walking speed, foot and hand tapping frequency, diffusion tensor imaging (DTI) data, and the excitability of the corticospinal tract to the target muscle [tibialis anterior (TA)] were quantified. The TA motor evoked potential (MEP) increased significantly after the BCIassociative intervention, but not for the BCInonassociative group. FM scores (0.8 ± 0.46 point difference, P = 0.01), foot (but not finger) tapping frequency, and 10-m walking speed improved significantly for the BCIassociative group, indicating clinically relevant improvements. Corticospinal tract integrity on DTI did not correlate with clinical or physiological changes. For the BCI as applied here, the precise coupling between the brain command and the afferent signal was imperative for the behavioral, clinical, and neurophysiological changes reported. This association may become the driving principle for the design of BCI rehabilitation in the future. Indeed, no available BCIs can match this degree of functional improvement with such a short intervention.
brain-computer interfaces (BCIs) intended for the restoration of lost motor function have been explored only recently. The activation of relevant brain areas generated by a patient's intention to move is detected, interpreted, and used to drive an external device, e.g., electrical stimulator or robot. The hypothesis is that the afferent signal generated artificially induces central nervous system plasticity because it is in causal association with the cortical activity associated with the intention to move. Several research groups have recently provided evidence that this type of BCI leads to functional improvements in upper limb or hand function (Ang et al. 2010; Broetz et al. 2010; Cincotti et al. 2012; Daly et al. 2009; Kasashima-Shindo et al. 2015; Li et al. 2014; Mukaino et al. 2014; Pichiorri et al. 2015; Ramos-Murguialday et al. 2013; Young et al. 2014), although others have found no changes (Ang et al. 2014; Buch et al. 2008) and data are lacking for the use of such a BCI for altering lower limb function (Teo and Chew 2014). These studies report evidence for neuroplasticity, typically inferred from an improved performance of the BCI (Buch et al. 2008; Li et al. 2014), from alterations in the amplitude (Broetz et al. 2010; Cincotti et al. 2012; Li et al. 2014; Pichiorri et al. 2015) or latency (Yilmaz et al. 2013) of the extracted electroencephalographic (EEG) signal, or by functional magnetic resonance imaging (fMRI) (Mukaino et al. 2014; Song et al. 2014; Young et al. 2014). However, there are no reports on the alterations in the excitability of the cortical projections to the target muscle following BCI interventions, although this is a natural consequence of learning new motor skills (Pascual-Leone et al. 1995; Perez et al. 2004). In addition, only a few previous studies have explored the underlying mechanisms of the induced plasticity using fMRI (Ramos-Murguialday et al. 2013) or EEG connectivity (Pichiorri et al. 2015), while no studies have implemented transcranial magnetic stimulation (TMS). According to Hebb, synapses are strengthened through correlated activation of two different inputs to a postsynaptic neuron yet weakened by uncorrelated activity (Hebb 1949). This implies that the sensory signal artificially induced through the BCI must be associated to relevant brain activation with specific timing in order to induce plasticity.
In a recent series of studies, we have introduced an innovative noninvasive BCI designed specifically for neuromodulation (Mrachacz-Kersting et al. 2012a, 2012b; Niazi et al. 2012; Xu et al. 2014) based on the principle of Hebbian associativity. In this BCI, the slow component of the scalp EEG, i.e., the movement-related cortical potential (MRCP), extracted with optimized temporal-spatial filtering (Niazi et al. 2011) and manifold learning algorithms (Xu et al. 2014), was used to detect the intent of the user's movement execution. The MRCP is a cortical potential generated when a movement is either imagined or performed and occurs for both self-initiated (Kornhuber and Deecke 1965) and cue-based (Walter et al. 1964) movements. The MRCP is comprised of several well-defined components, linked to specific neurophysiological mechanisms where the peak negativity (PN) is associated to movement execution. Once the MRCP is detected, the artificial activation of somatosensory afferents projecting onto the motor cortex is triggered by means of noninvasive direct nerve stimulation. The signal is timed to arrive at the cortex during the peak negative phase of the MRCP and therefore induces a causal and systematic relationship between the sensory signals arising from muscles involved in the movement and the volitionally generated brain wave during imagination, attempt, or execution of that movement. This timing is critical, as we have shown that neither earlier nor later arrival can induce plasticity (Mrachacz-Kersting et al. 2012a). In healthy subjects, this BCI intervention induces plasticity that is long-lasting and specific to the muscle targeted (Mrachacz-Kersting et al. 2012a; Niazi et al. 2012; Xu et al. 2014). Previous studies have timed the sensory stimulus to arrive at the motor cortex at the precise time that a transcranial magnetic stimulus (TMS) was applied over the motor cortex representation of the target muscle (Stefan et al. 2002). This type of intervention, termed paired associative stimulation (PAS), induces plasticity similar to what we have shown in our BCIassociative intervention in healthy participants. Since the PN of the MRCP represents the maximal activation of the motor cortex, our intervention is designed to replace the volley induced by TMS.
In the present proof-of-concept study, we present for the first time the application of this novel associative BCI to chronic stroke patients with motor disability and hypothesize that it would lead to an increased output of the motor cortex to the target muscle. The demonstration of this hypothesis would strongly support the theory that timing is critical in neuromodulatory BCIs and that associativity is the main physiological mechanism underlying the induced plasticity. In an additional control experiment, a control group was exposed to the same protocol, with the exception that the afferents were activated randomly in relation to the user's intent (BCInonassociative intervention). This is, to our best knowledge, the first systematic study on chronic stroke patients that explicitly explores the associative long-term potentiation theory within a BCI rehabilitation approach that includes patients' volition.
METHODS
Ethical approval.
Patient demographical data and baseline clinical evaluation are shown in Table 1. Nineteen male and three female patients (49.57 ± 12.52 yr) underwent neuropsychological assessment, with none meeting DSM-IV criteria for diagnosis of dementia. Inclusion criteria encompassed age over 18 yr having suffered from superior division middle cerebral artery (MCA) stroke 3–24 mo before recruitment and ability to follow commands (no or limited cognitive impairment). Patients were excluded if they presented with concomitant neurological or other severe medical problems, seizure history, cognitive impairments, treatment with drugs that act on central nervous system, complete paralysis of legs, cardiovascular or respiratory symptoms contraindicative of walking, contraindications to TMS or MRI, and any other significant non-stroke-related impairment affecting walking. Procedures were approved by the Ethics Committee of the Clinical Center of Serbia, and all patients provided their written informed consent.
Table 1.
Patient demographic data for main BCIassociative experiment and control BCInonassociative experiment
Patient No. | Age, yr | Sex | Time After Stroke, mo | MCA Stroke Side | Type of Ischemic Lesion |
---|---|---|---|---|---|
BCIassociative patient group | |||||
1 | 29 | M | 10 | L | Cortico-subc |
2 | 61 | M | 24 | L | Cortico-subc |
3 | 30 | F | 23 | L | Cortico-subc |
4 | 41 | F | 23 | L | Cortical |
5 | 53 | M | 11 | L | Cortical |
6 | 52 | M | 24 | L | Cortical |
7 | 58 | M | 9 | L | Cortico-subc |
8 | 43 | M | 19 | R | Cortico-subc |
9 | 57 | M | 12 | L | Cortico-subc |
10 | 23 | M | 12 | R | Cortical |
11 | 45 | M | 9 | L | Cortico-subc |
12 | 51 | M | 14 | R | Cortico-subc |
13 | 59 | M | 10 | L | Cortico-subc |
Mean | 46.31 | 15.38 | |||
SD | 12.51 | 6.2 | |||
BCInonassociative patient group | |||||
14 | 55 | M | 20 | L | Cortico-subc |
15 | 58 | M | 22 | R | Cortico-subc |
16 | 63 | F | 15 | R | Cortico-subc |
17 | 51 | M | 23 | R | Cortico-subc |
18 | 59 | M | 14 | L | Cortico-subc |
19 | 42 | M | 21 | L | Cortico-subc |
20 | 36 | M | 22 | R | Cortico-subc |
21 | 65 | M | 14 | L | Cortico-subc |
22 | 68 | M | 11 | R | Cortico-subc |
Mean | 55.22 | 18 | |||
SD | 10.63 | 4.47 |
Patient demographic data for the main BCIassociative (patients 1–13) and control BCInonassociative (patients 14–22) experiments are shown. MCA, middle cerebral artery.
Movement-related cortical potential.
Monopolar EEG signals were recorded by an EEG amplifier (Nicolet 1, VIASYS Healthcare) at 1,024 Hz (0.016–70 Hz). Ag/AgCl scalp electrodes were placed according to the International 10-20 System in positions FP1, F3, F4, Fz, Pz, P3, P4, C3, C4, and Cz. Impedance was maintained below 5 KΩ. Both earlobes were used as a reference, and the ground electrode was placed at the nasion.
Patients were asked to attempt a dorsiflexion of the foot contralateral to the lesion site 30–50 times. The experimental setup is depicted in Fig. 1B. A custom-made MATLAB script provided visual information via a screen positioned 2 m in front of the subject on when to mentally prepare, execute, and release the movement (Fig. 1D). Patients were instructed to attempt to perform a single dorsiflexion movement as fast as possible when the cursor had reached the upward turn (Fig. 1D) and to maintain the new position for 2 s, following which they relaxed again 4–5 s prior to the next cue being provided. Data from recorded EEG trials were used to quantify the time of PN of the MRCPs before proceeding to the intervention described in Interventions.
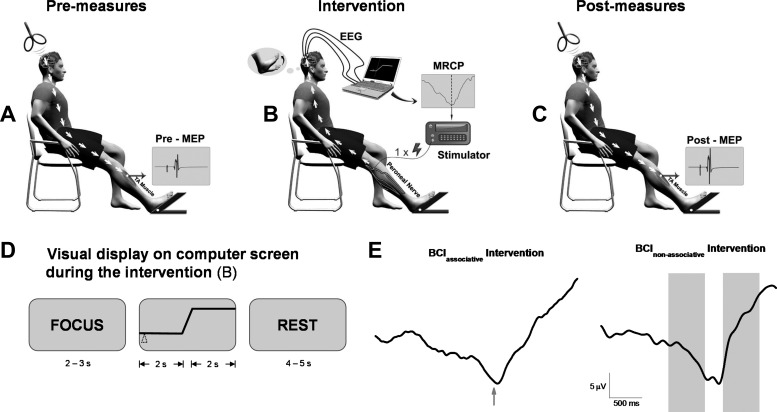
Schematic of the experimental setup. A: preintervention quantification of the excitability of the cortical projections to the target muscle, tibialis anterior (TA), with noninvasive transcranial magnetic stimulation (TMS). Subjects were seated with the TA relaxed while 60 or 72 stimuli at 5 or 6 different intensities were applied. MEP, motor evoked potential. B: schematic of the brain-controlled electrical stimulation of the target muscle. Subjects watched a screen placed 2 m in front of them on which a cue provided information on when to attempt the dorsiflexion movement. Relevant brain activity was measured, detected, and converted into an output command for an electrical stimulator. The stimulator applied a single pulse (1-ms duration at an intensity of motor threshold) to the deep branch of the common peroneal nerve. The induced sensory signal produced was timed to arrive at the motor cortex during the time of maximum activation of the motor cortex as seen in the EEG signal for the BCIasscociative group and randomly for the BCInonassociative group. MRCP, movement-related cortical potential. Fifty such pairs were performed in 2 sets of 25. C: immediately after intervention and 30 min later; measures as for A. D: the visual display shown to the patients during the intervention. The word “FOCUS” appeared on the screen initially, followed by the schematic of a step function. Subjects were required to start the attempted movement once the moving cursor (triangle) reached the upward slope. The word “REST” appeared last on the screen. E: illustration of the timing of the single electrical stimulus in relation to the MRCP during the BCIassociative intervention (arrow, time of peak negativity) and during the BCInonassociative intervention (shaded areas represent time window where the single electrical stimulus was triggered). Data are from 2 different patients of each intervention group.
Feature extraction from MRCP.
EEG data were divided into epochs of 3 s (from 2 s before to 1 s after the visual cue) for each attempted movement and band-pass filtered from 0.05 Hz to 10 Hz, and subsequently a Laplacian channel (McFarland et al. 1997) was used to enhance the MRCP in each epoch. Next, a window of 500 ms on either side of task onset was chosen. If any epoch's PN was outside the selected window it was discarded. Epochs with EOG activity exceeding 125 μV were also discarded. Based on these remaining epochs, the mean PN was defined as the time of occurrence of the minimum value of the averaged MRCP in relation to the visual cue. The mean PN was used to calculate the points in time for when to apply the peripheral stimulation in the subsequent intervention session.
Recording and stimulation.
Surface electrodes (20 mm Blue Sensor Ag/AgCl, AMBU) were used to record the electromyographic (EMG) activity of the tibialis anterior (TA) muscle of the affected leg. EMG data were sampled at 4 kHz, amplified, and band-pass filtered at 10 Hz to 1 kHz.
A monophasic transcranial magnetic stimulator (Magstim 200, Magstim) with a focal figure of eight double-coned coil (110-mm diameter) was used to apply single pulses (inducing a posterior to anterior directed current in the brain) to elicit a motor evoked potential (MEP) in the TA muscle. MEPs were elicited before, after, and 30 min after the cessation of the intervention (for procedure see Experimental procedures) and served as the main outcome measure.
Peripheral nerve stimulation was performed during the interventions only. The deep branch of the common peroneal nerve (CPN—L4 and L5) was stimulated with an external stimulator (Noxitest IES 230, Aalborg, Denmark) with the cathode proximal. A suitable position for the stimulation electrodes (Pals Platinum, Axelgaard Manufacturing) was located where a palpable response was produced in the distal tendon of the TA muscle with no activity from the synergistic peroneal muscles and no activity from the antagonist soleus. This site corresponded to a point just anterior to the level of the caput fibulae. The pulse width was set to 1 ms and the stimulation intensity to motor threshold (MT).
Experimental procedures.
Details of the experimental procedures are shown in Fig. 1, A and C. Subjects were seated with their right and left feet resting on a footplate. The intensity of the magnetic stimulation was set to 50% of stimulator output to find the optimal site for evoking a MEP in the target muscle. In some patients (n = 3) this had to be increased to 90% in order to evoke a MEP. Initially we delivered three consecutive stimuli over Cz and proceeded to move in ~1-cm steps laterally. The hot spot was identified as that area where the most consistent MEPs were elicited in the three trials. This position was marked on the patient's head with a felt pen to ensure that the stimuli were consistently delivered over the same area of the motor cortex during the experimental session. Subsequently, the resting MT (RMT), defined as the highest stimulus intensity that in no more than 5 of 10 consecutive stimuli evoked a MEP with amplitude of ~50 μV while the muscle was at rest, was identified. Ten MEPs were subsequently elicited in the resting TA at five TMS intensities: 90%, 100%, 110%, 120%, and 130% of RMT. The stimuli were delivered randomly every 5–7 s. The mean peak-to-peak TA MEP amplitudes were extracted before, after, and 30 min after the cessation of the intervention. In some patients it was not possible to evoke a MEP in the TA at intensities below 90% of the stimulator output. In these cases (n = 3) the input-output relation was obtained by stimulating at 87%, 90%, 93%, 95%, and 98% of the stimulator output.
Interventions.
The BCIassociative intervention protocol (n = 13) consisted of a single electrical stimulation (ES) timed so that the artificially generated afferent flow arrived at the PN of the MRCP as outlined in detail in our previous publication (Mrachacz-Kersting et al. 2012a). In that study, we provided conclusive proof that only if the ES was timed so that the resulting afferent inflow would coincide with PN was significant plasticity induced. If it arrived before or after PN, no significant changes were observed (Mrachacz-Kersting et al. 2012a). The timing was calculated according to the following equation: mean PN − 50 ms. The 50 ms represents the mean latency for the afferent inflow resulting from the peripheral stimulus to reach the somatosensory cortex plus a cortical processing delay and is based on previous work (Mrachacz-Kersting et al. 2007). Figure 1E, left, shows an example from one patient, where the arrow denotes the instant of the ES being triggered with a delay of 50 ms in relation to the PN. A second intervention termed the BCInonassociative intervention (n = 9) served as a control condition. Here, the ES was delivered randomly without any association to the PN of the MRCP. Figure 1E, right, shows an example from one patient, where the two shaded areas represent the time window of where the ES was triggered in a randomized order across the two windows. A total of 30–50 pairings (every 10–12 s) were applied. Subjects attended three separate testing sessions within 1 wk. A minimum of 24 h elapsed between sessions. Patients were blinded as to the intervention they received, and the two interventions were performed at different time points, with the BCIassociative intervention conducted first. A posteriori validation verified that the groups matched (Table 2).
Table 2.
Patient clinical and behavioral data for main BCIassociative experiment and control BCInonassociative experiment
10-m Walk Test, m/s | mRS | LE-FM | ASS | |||||
---|---|---|---|---|---|---|---|---|
Patient No. | Pre | Post | Pre | Post | Pre | Post | Pre | Post |
BCIassociative patient group | ||||||||
1 | 0.99 | 1.08 | 2 | 2 | 24 | 26 | 2 | 2 |
2 | 0.36 | 0.37 | 3 | 3 | 11 | 11 | 5 | 5 |
3 | 1.37 | 1.39 | 1 | 1 | 32 | 32 | 0 | 0 |
4 | 0.88 | 1.01 | 2 | 2 | 30 | 30 | 2 | 2 |
5 | 0.33 | 0.34 | 3 | 3 | 18 | 18 | 4 | 3 |
6 | 0.85 | 0.98 | 2 | 2 | 25 | 27 | 2 | 2 |
7 | 0.65 | 0.73 | 2 | 2 | 22 | 24 | 2 | 2 |
8 | 0.65 | 0.95 | 2 | 1 | 30 | 32 | 0 | 0 |
9 | 0.78 | 0.79 | 2 | 2 | 31 | 31 | 0 | 0 |
10 | 0.93 | 0.97 | 1 | 1 | 32 | 32 | 0 | 0 |
11 | 0.74 | 0.76 | 2 | 2 | 26 | 27 | 2 | 2 |
12 | 0.99 | 1.09 | 2 | 2 | 26 | 27 | 3 | 3 |
13 | 0.39 | 0.40 | 3 | 3 | 17 | 17 | 4 | 3 |
Mean | 0.76 | 0.84 | 2.08 | 2.00 | 24.92 | 25.69 | 2.00 | 1.85 |
SD | 0.29 | 0.31 | 0.64 | 0.71 | 6.49 | 6.61 | 1.68 | 1.52 |
BCInonassociative patient group | ||||||||
14 | 1.24 | 1.25 | 2 | 2 | 22 | 22 | 2 | 2 |
15 | 1.21 | |||||||
16 | 0.98 | 0.96 | 1 | 1 | 32 | 23.5 | 0 | 0 |
17 | 1.09 | 0.98 | 2 | 2 | 22 | 22 | 2 | 2 |
18 | 1.09 | 1.12 | 2 | 2 | 32 | 32 | 0 | 0 |
19 | 1.32 | 1.29 | 1 | 1 | 27 | 27 | 2 | 2 |
20 | 1.80 | 1.51 | 1 | 1 | 33 | 33 | 0 | 0 |
21 | 0.82 | 0.79 | 2 | 2 | 21 | 21 | 2 | 2 |
22 | 0.87 | 0.84 | 3 | 3 | 22 | 22 | 3 | 3 |
Mean | 1.16 | 1.09 | 1.75 | 1.76 | 26.44 | 24.44 | 1.38 | 1.39 |
SD | 0.28 | 023 | 0.71 | 0.69 | 5.21 | 5.34 | 1.19 | 1.17 |
Patient clinical and behavioral data for the main BCIassociative (patients 1–13) and control BCInonassociative (patients 14–22) experiments are shown. mRS, modified Rankin scale score; NIHSS, National Institutes of Health Stroke Scale score; LE-FM, Lower-Extremity Fugl-Meyer assessment—motor performance; ASS, Ashworth scale for spasticity of the affected leg.
Clinical and behavioral measures.
Upon the patients' first and last visit, they were assessed with several clinical scales by a clinician blinded to the protocol: modified Rankin scale score (mRS) (Bonita and Beaglehole 1988), National Institutes of Health Stroke Scale (NIHSS) score (Lyden et al. 2001), Hamilton Depression Rating Scale (HDRS) score (Hamilton 1967), the Lower-Extremity Fugl-Meyer assessment (LE-FM)—motor performance (Fugl-Meyer et al. 1975), and the Ashworth scale for spasticity (ASS) of the affected leg (Bohannon and Smith 1987).
All subjects performed the 10-m walk test at their fastest pace. A subgroup of seven patients from the BCIassociative group and all patients from the BCInonassociative group performed two additional motor tasks: index finger and foot tapping of the affected side at the fastest possible pace in the sagittal plane. This was to establish the specificity of the protocol on inducing functional changes. Tapping data were recorded for 14–27 s depending on the patient's ability with a wireless inertial sensor system, as described in Djuric-Jovicic et al. (2011). A high-performance 12-bit digital accelerometer LIS3LV02 (SGS-Thomson Microelectronics), with ±6g range of sensors was modified by displacing one triaxial accelerometer from the inertial unit box. This was placed on the middle phalanges of the hand or on the metatarsal bone of the foot and secured with elastic Velcro bands. The number of finger or foot taps was quantified during the patient-specific time interval.
Magnetic resonance imaging parameters and analysis.
Diffusion tensor imaging (DTI) MRI scans were obtained on a 1.5-T Achieva system (Philips) using a pulsed gradient spin-echo single-shot echo-planar sequence (TR = 6,714, TE = 86, flip angle = 90°, matrix size = 112 × 110; 50 axial slices, thickness = 2.6 mm with no gap), with diffusion-encoding gradients applied in 65 noncollinear directions (b factor = 1,000 s/mm2, 1 average). These data were collected only for the BCIassociative group, as we were interested in quantifying whether DT MRI measures of the corticospinal tracts (CST) and corpus callosum could predict alterations induced by the BCIassociative intervention.
Evaluation of DTI data.
DT MRI analysis was performed with FSLv4.1.7 (http://www.fmrib.ox.ac.uk/fsl/). Diffusion-weighted images were first corrected for distortions induced by eddy currents and then registered to the non-diffusion-weighted volume (b = 0) with 6 degrees of freedom transformation to correct for head movements. The DT was estimated on a voxel-by-voxel basis with the DTI fit toolbox, part of the FMRIB Diffusion Toolbox within FSL. Maps of mean diffusivity (MD) and fractional anisotropy (FA) were obtained. To transform diffusion data from DT MRI native space to the Montreal Neurological Institute (MNI) space, the following procedure was performed: 1) FA volumes were aligned to the FA template in MNI space with FMRIB's linear Image Registration Tool (FLIRT) with 12 degrees of freedom, 2) the nonlinear transformation to FSL's FA template was then computed with FMRIB's nonlinear Image Registration Tool entering the information from the linear transformation and obtaining a coefficient file with both the linear and nonlinear components of the transformation to MNI space, 3) inverse transformations were calculated from the coefficient file obtained from FNIRT. Seeds for tractography of the CST and corpus callosum were defined in the MNI space on the FA template provided by FSL. Regions of interest (ROIs) were defined manually on sagittal or axial slices based on a priori knowledge of the anatomy of the tracts. Seeds were drawn where these tracts pass through a bottleneck in order to include the highest number of fibers constituting the tract in the starting seed for tractography. Seeds were then transformed back to the native diffusion space, and tractography was performed with a single-seed approach. Masks were used to exclude fibers from neighboring tracts. The seed for the corpus callosum was a sagittal ROI including the four median slices on which the corpus callosum is clearly visible. For the CST, an axial ROI was drawn at the top of the bulbar pyramids of each side and included four slices. Average FA and MD values were obtained from the CST and the corpus callosum. Relative asymmetry indexes for FA (reflecting the preferential directionality of water diffusion along the white matter tracts) were calculated according to the following formula: (FAunaffected − FAaffected)/(FAunaffected + FAaffected). Asymmetry indexes for MD (reflecting the magnitude of diffusion) were calculated according to the following formula: (MDunaffected − MDaffected)/(MDunaffected + MDaffected). Asymmetry indexes of FA and MD values comparing lesional with nonlesional hemispheres were correlated with motor impairment scores, functional changes, and neurophysiological measures.
Statistical procedures.
The main outcome measures were the changes in MEP size as well as all the clinical tests. One-way repeated-measures analysis of variance (ANOVA) with the factor “day” (day 1, day 2, and day 3 of the intervention) was used to establish the repeatability of PN, the RMT, and ES intensity. Separate two-way ANOVAs were performed to evaluate the premeasures of TMS-evoked MEPs across all testing days for both groups, with “day” (intervention day 1, day 2, and day 3) and “stimulus intensity” (90%, 100%, 110%, 120%, and 130% RMT) as the repeated-measures factors. Furthermore, separate three-way repeated measures ANOVAs with factors “time” (pre, post 0, and post 30), “day” (intervention day 1, day 2, and day 3), and “stimulus intensity” (90%, 100%, 110%, 120%, and 130% RMT) were used to investigate the effects of the days and interventions on changes in MEP amplitude. Student's t-tests were used to compare clinical and behavioral measures before and after all interventions. Correlation coefficients were determined for DT MRI measures (average FA and MD values from CST and corpus callosum) and MEP changes as well as between DT MRI measures and behavioral changes. Statistical significance was set to P < 0.05. Tukey's honestly significant difference with Bonferroni correction post hoc tests were administered to determine the locus of the differences. All statistical analyses were conducted with SPSS.
RESULTS
Reliability of MRCP.
Patients performed 30–50 attempted movements per session, where an average of 12 ± 3 attempts were rejected because of eyeblinks or movement artifacts. For seven patients we investigated the repeatability of the MRCP across days. The PN occurred at −20.49 ± 77.71 ms in relation to the cue indicating to attempt the dorsiflexion task. The time and standard deviation of the occurrence of the PN for each of the seven subjects across the three sessions were not statistically different [F(6,12) = 1.76; P = 0.29].
Changes in output properties of motor cortex for BCIassociative intervention group.
Three patients were excluded from the MEP analysis as it was not possible to record data for all days and time points. The size of the MEP evoked at the highest stimulation intensity before the application of the interventions across all patients attained values of 191 ± 148 μV, 284 ± 275 μV, and 331 ± 351 μV for intervention days 1–3, respectively. Two-way ANOVA on the presession measures found no significant interaction between days and stimulation intensity [F(8,80) = 1.21, P = 0.299]. After pooling the interaction term, “days” was not significant [F(2,20) = 0.77, P = 0.473], indicating that for all days the experiment started with similar baseline excitability across all subjects (Table 3).
Table 3.
Results of separate two-way repeated-measures ANOVAs for each group investigating pretest MEP amplitudes across days
Effect | F Statistic | df | P Value |
---|---|---|---|
BCIassociative patient group | |||
Day | 0.78 | 2,20 | 0.473 |
Intensity | 11.56 | 4,40 | <0.001 |
Day × intensity | 1.22 | 8,80 | 0.299 |
BCInonassociative patient group | |||
Day | 0.48 | 2,14 | 0.629 |
Intensity | 6.65 | 4,28 | 0.001 |
Day × intensity | 0.77 | 8,56 | 0.631 |
Values are results of the separate 2-way repeated-measures ANOVAs for each group investigating the pretest motor evoked potential (MEP) amplitudes across days. The 2 repeated-measures factors were “day” (intervention day 1, day 2, and day 3) and “stimulus intensity” (90%, 100%, 110%, 120%, and 130% of resting motor threshold). All significant results are in bold. Note that the 2-way interaction for both groups was not significant, so the 2-way interaction term was pooled.
Figure 2A shows the averaged MEP data for one subject before, after, and 30 min after each of the 3 days in relation to the TMS intensity; Fig. 2A, inset, represents the raw MEP at 120% RMT for day 1 for this subject. Also shown are the respective averaged MRCP signals for this subject at the start of each session (Fig. 2B). Figure 3, A–C, contains the averaged TA MEP size for all subjects plotted against RMT for each intervention session. Data are expressed as a fraction of the maximum TA MEP prior to the intervention.
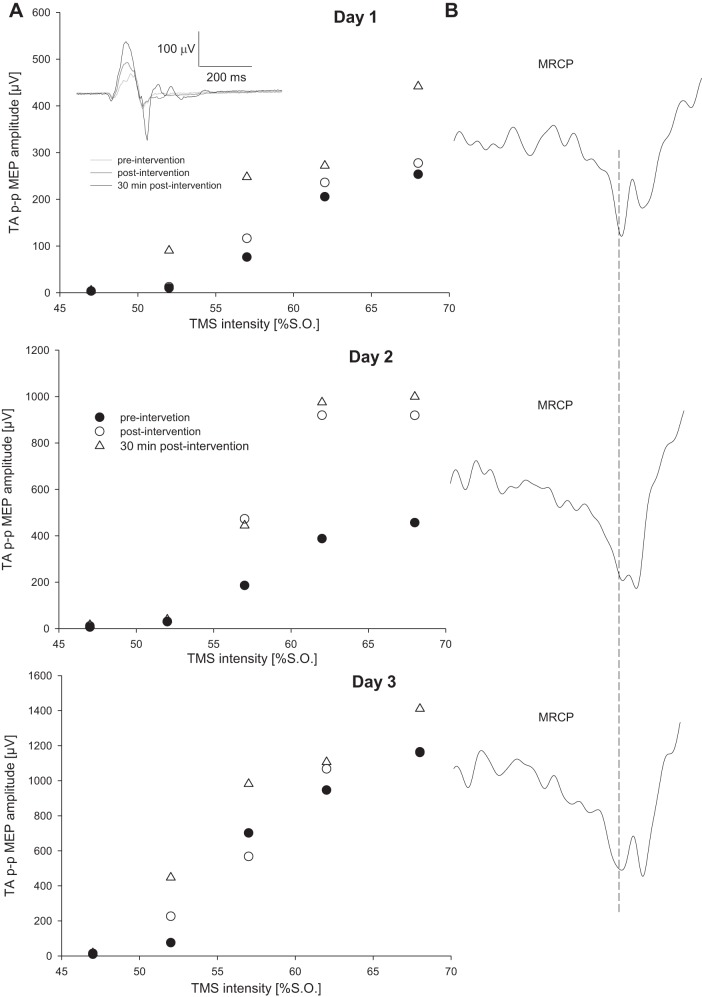
Changes in motor output after the BCIassociative intervention for a single representative participant. A: TA MEP recruitment curve before and after the 3 interventions. TA MEP size is expressed as the peak-to-peak amplitude (p-p MEP) and TMS intensity as % of the stimulator output (S.O.). Each data point represents the average of 12 stimuli. Inset: representative MEP for this subject for a stimulation intensity of 62% S.O. at day 1. B: slow MRCP recorded during imaginary movement during the intervention. Data are the average of 30 trials. Vertical dashed line indicates when the cue was presented indicating when the patient should start to attempt the dorsiflexion movement. All data are for n = 1.
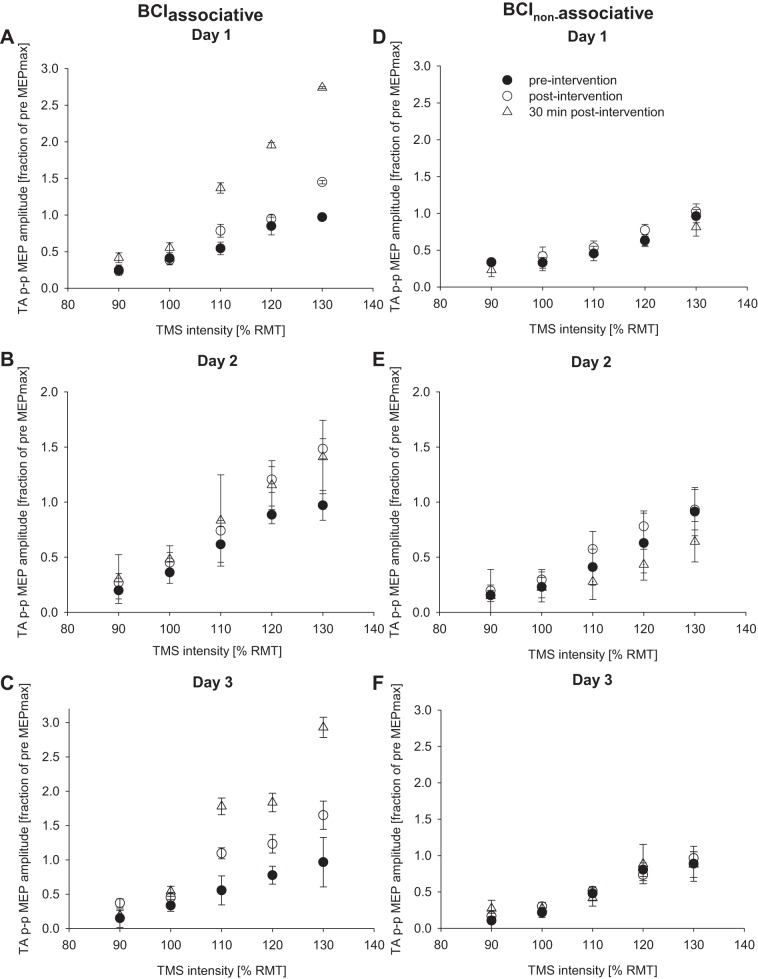
Input-output properties of the TA MEP before, immediately after, and 30 min after the cessation of the BCIassociative (A–C) and BCInonassociative (D–F) interventions across all subjects. TA MEP size is expressed as a fraction of the maximum peak-to-peak TA MEP (TA p-p MEP) amplitude before any intervention. Each graph represents a different day of the intervention, and each data point represents the average of 11 subjects (A–C) or 8 subjects (D–F). Error bars show SE. RMT, resting motor threshold.
In the full-model three-way ANOVA, the three-way interaction was not significant (P = 0.928). After pooling the three-way interaction term, there was a significant time × stimulation intensity interaction [F(8,72) = 4.06, P < 0.001]. Simple main effects post hoc analyses revealed that, at the 90%, 110%, and 130% intensities, MEPs were significantly larger 30 min after the intervention than before the intervention (P = 0.016, P = 0.030, and P = 0.022, respectively). Furthermore, MEPs at the 130% intensity were significantly larger immediately after the intervention compared with before the intervention. The day × time and day × intensity interactions, along with the day main effect, were not significant (all P > 0.21).
These analyses demonstrate the effectiveness of the proposed intervention in inducing significant neurophysiological changes. The intervention resulted in a significant increase of the TA MEP size, and this outlasted the intervention time by at least 30 min.
Control experiment: changes in output properties of motor cortex for BCInonassociative intervention group.
The size of the MEP evoked at the highest stimulation intensity before the interventions across all patients attained values of 517 ± 489 μV, 448 ± 247 μV, and 454 ± 320 μV for the BCInonassociative sessions. There was no significant interaction between days and stimulation intensities [F(8,56) = 0.77, P = 0.631]. After pooling the interaction terms, “day” was found to be not significant [F(2,14) = 0.48, P = 0.629], indicating that for all three BCInonassociative intervention days, the experiment started with similar baseline excitability across subjects (Table 3).
Figure 3, D–F, show the averaged TA MEP size before and after the intervention for all subjects plotted against RMT for each intervention session. Data are expressed as a fraction of the maximum TA MEP before the intervention.
In the full-model three-way ANOVA, none of the interaction terms (3-way and 2-way) were significant (all P > 0.22). After pooling all interaction terms, the three-way analysis on the main effects found that factors “day” and “time” were not significant (both P > 0.39), while the factor “stimulation intensity” [F(4,28) = 9.14, P < 0.001] was significant. For stimulation intensity, the post hoc comparisons found that the higher stimulation intensity resulted in larger MEP magnitudes than lower stimulation intensities, which was expected. These results indicate that the random stimulation did not have any effect on the TA MEP size following the intervention.
Clinical and behavioral measures.
Baseline clinical scores for the BCIassociative intervention group and for the BCInonassociative intervention group are shown in Table 2. There were no statistically significant differences between the BCIassociative and the BCInonassociative intervention groups for ASS (P = 0.47) and LE-FM (P = 0.86) upon enrollment. However, the patients in the BCIassociative intervention group were significantly slower in the 10-m walking test [t(19) = 2.339, P = 0.03].
The clinical assessments were repeated after the 3 days of both interventions. For the BCIassociative intervention group, significant differences were obtained in the 10-m walking test [t(12) = 3.279, P = 0.007] and the LE-FM scale before and after intervention [t(12) = −2.993, P = 0.011] as shown in Table 2. No significant changes were detected for mRS (P = 0.34) or ASS (P = 0.186). For the BCInonassociative patients, no significant changes were detected after the intervention for any of the clinical measures (10-m walking test, P = 0.688; LE-FM scale, P = 0.25; mRS, P = 0.35; ASS, P = 0.977).
Before the intervention, the BCIassociative patients performed 80 ± 16 taps with the index finger and 70 ± 16 foot taps of the affected side. After the intervention, a statistically significant difference [t(6) = −3.099, P = 0.02] was observed for the foot tapping task, where tapping frequency increased from 3.43 ± 0.85 Hz before to 4.05 ± 0.52 Hz after. No statistical significance was found (P = 0.48) for the index finger tapping task. For the BCInonassociative patients, neither foot tapping frequency (pre: 3.29 ± 1.23 Hz, post: 3.16 ± 1.28 Hz; P = 0.54) nor index finger tapping frequency (pre: 2.26 ± 1.48 Hz, post: 2.98 ± 2.48 Hz; P = 0.23) changed significantly.
Diffusion tensor imaging.
These data were only analyzed for the BCIassociative patient group. Four patients were not scanned because of personal reasons. There was a significant decrease of FA values for the CST originating in the lesioned (0.54 ± 0.48) vs. the nonlesioned (0.59 ± 0.03) side [t(8) = −3.863, P = 0.005]; FA asymmetry = 0.048 ± 0.038. MD values were also significantly different between the two sides [lesioned: 0.85 ± 0.08; nonlesioned: 0.76 ± 0.03; t(8) = 3.928, P = 0.004]; MD asymmetry = −0.05 ± 0.04. FA and MD values of the corpus callosum were 0.56 ± 0.02 and 0.96 ± 0.07, respectively. No significant correlation was found between FA values with any of the neurophysiological or functional changes induced by the intervention.
DISCUSSION
In this proof-of-concept study, the BCIassociative intervention led to significant neurophysiological and behavioral improvements in all patients investigated after only three sessions. No such alterations were observed for patients engaged in the BCInonassociative intervention. Importantly, the effective duration of each BCIassociative intervention session over which the patients were required to be engaged in the task itself was only ~15 min per session. The key feature of our paradigm is that the expected sensory information, triggered by user volition, coincides with the physiologically generated brain activation at the cortical level, satisfying the requirements of the Hebbian associative learning theory (Hebb 1949).
The importance of the precise temporal association between a peripherally generated afferent volley and the activation of the motor cortex was first reported by Stefan and colleagues (Stefan et al. 2002). Their intervention was comprised of a single electrical stimulus delivered to the median nerve that innervates the target muscle abductor pollicis brevis (APB) and a single stimulus applied directly to that area of the motor cortex with projections to the APB with noninvasive TMS. The TMS was triggered 25 ms after the peripheral stimulus, and this represents the delay for the afferent volley to reach the motor cortex. Only with this specific interval of 25 ms between the two stimuli was a significant change in the excitability of the cortical projections to the APB observed. A similar protocol has been applied to the TA, although the delay between the two stimuli was reported to be longer (50 ms) for any significant induction of plasticity (Mrachacz-Kersting et al. 2007). In the present study, the ES was timed to occur 50 ms before the PN of the MRCP, since the PN is associated to movement execution and may be seen as a more natural activation of the motor cortex compared with TMS. In our past study using this paradigm in healthy participants, we demonstrated that only if the ES was timed so that the generated afferent signal arrived at PN was a significant effect observed. In the present study we thus did not expose our patients to different time intervals between the ES and PN. Instead, the control condition involved the BCInonassociative patient group receiving ES timed to occur randomly throughout the MRCP (Fig. 1E, right). In this way, there was no association between the arrival of the afferent inflow generated by the ES and the PN of the MRCP. It should be noted that the BCInonassociative group was tested separately in a control experiment and thus it was not possible to run a statistical analysis with a group factor that might have provided a more robust analysis.
The signal modality (MRCP) used here is natural and physiologically associated with the movement (Kuhtz-Buschbeck et al. 2003; Leifert-Fiebach et al. 2013; Salinet et al. 2012), so no user training was necessary to produce this signal. This is in contrast to other BCIs for neuromodulation in stroke where patients were required to spend several sessions (15 or more, over the duration of months) for learning to produce controllable brain signals (Ang et al. 2009; Buch et al. 2008). Stroke patients have altered temporal as well as spatial MRCPs (Platz et al. 2000; Yilmaz et al. 2013), and it is known that a patient's ability to perform a motor task accurately is significantly enhanced when a cue is provided (Heremans et al. 2009). This was the case in our intervention and may have led to the enhanced effects in the BCIassociative group.
The BCIassociative intervention led to statistically significant improvements in the LE-FM scale and the 10-m walking test in all subjects (Table 2) and in foot tapping frequency in a subgroup of patients, which was not expected. Although from a clinical perspective the improvements are too small to be considered significant, it is important to mention that the patients only performed three intervention sessions and each session comprised only 30–50 pairings. It is likely that the intervention would lead to even greater improvements if applied over a longer duration as has been done for past BCIs in this context (Ramos-Murguialday et al. 2013). The control group taking part in the BCInonassociative intervention showed no such improvements, so that the changes in the patients of the BCIassociative group cannot be attributed to an experimental participation effect. The improvements occurred in tasks that were unrelated to the task executed in the BCI intervention. Similar reports of such transfer come from the area of spinal cord plasticity. Wolpaw and colleagues (Chen et al. 2014; Wolpaw 2007) have induced such plasticity by using an operant conditioning of a spinal reflex (the H reflex). Changes in this simple reflex arc are transferred to dynamic tasks in lesioned animals, and most recently similar results have been shown in spinal cord-injured humans (Thompson and Wolpaw 2015).
All patients in the BCIassociative group showed significant increases in the MEP recorded at the TA after the intervention. The presence of MEPs in the TA in the acute phase following a stroke has been associated to recovery of motor function (Hendricks et al. 2003b). In chronic patients, increases in MEP amplitude over time are an indicator for functional improvements (Piron et al. 2005). In the present study, the changes in MEP size through all three sessions were only weakly correlated with the improvements in function. In light of previous publications, this was unexpected. However, a few recent reports have documented that improvements in motor function are not necessarily mirrored by alterations in the excitability of the cortical projections to the target muscle (Avanzino et al. 2011; Hendricks et al. 2003a). However, it is also important to note that although within each session the sizes of the MEPs were significantly increased for the BCIassociative intervention, there was no significant difference between the changes induced across the 3 days and baseline MEP values did not differ between days. This implies that, although a significant within-session effect is present, MEPs do not change across sessions. However, in the present proof-of-concept study patients only partook in a total of three sessions. It is likely that if the intervention was of longer duration, significant changes in MEP size would occur also across sessions.
The MEP amplitudes were significantly larger 30 min after the cessation of the BCIassociative intervention compared with immediately before. A similar effect has been reported in previous studies using PAS as the intervention and targeting lower limb muscles (Kumpulainen et al. 2012). This effect is not easily explained on the basis of on current knowledge on the underlying mechanisms following such interventions. It is likely caused by complex interactions between different neuronal populations in the motor cortex of the lesioned hemisphere. However, in the absence of invasive neuronal investigation any hypothesis about the precise nature of this effect remains speculative.
One possible reason for the significant improvements after so few intervention sessions and task repetitions reported here as opposed to other available BCIs may be directly related to the temporal association. After stroke that damages part of the sensory cortex, the intact part significantly enhances its output to both motor cortex and other cortical regions (Brown et al. 2009; Murphy and Corbett 2009). In our BCI intervention, the afferent volley induced by the peripheral nerve stimulus was timed to arrive during the movement execution phase of the MRCP and can thus be viewed as a means to guide the afferent input appropriately to restore the connectivity between the sensory cortex and the motor cortex. In healthy subjects when the timing was altered to either before or after this phase, no plasticity was induced (Mrachacz-Kersting et al. 2012a). This was substantiated by the results from the control BCInonassociative intervention, where the timing was such that the afferent volley arrived randomized during either the motor preparation phase or the reafferent phase but never during the movement execution phase. Taken together, the results from the BCIassociative and the BCInonassociative interventions underline the importance of the correct sequence of signals for inducing long-lasting plastic changes. The critical importance of the delay between motor command and sensory input shown here for patients and in previous studies for healthy subjects (Mrachacz-Kersting et al. 2012a) may also explain the substantially less efficient outcome of BCI neuromodulation in stroke patients in previous studies (for review see Silvoni et al. 2011).
Repeated activation of somatosensory afferents projecting onto M1 has a pivotal role in motor skill learning in monkeys (Pavlides et al. 1993). In a recent BCI study of chronic stroke patients, somatosensory feedback triggered from detected brain signals led to functional improvements, while visual feedback induced no such changes (Ono et al. 2014), thus further highlighting its importance. In the present study, the CPN, which is a mixed nerve, was electrically stimulated to generate the afferent volley. Since the intensity of the stimulus was set to MT, we expect that mainly afferents arising from muscle receptors were stimulated and thus provided their input to the sensory cortex. Sensory information arising from muscles contributes substantially (up to 50%) to the activation of muscles by the nervous system (Grey et al. 2004; Mazzaro et al. 2006; Nielsen 2004; Nielsen and Sinkjaer 2002). Sensory information is also modulated by the brain, allowing either large or small influences during a particular movement (Nielsen 2003). For example, during walking, sensory feedback from calf muscles directly activates these muscles during the push-off phase, where a strong contraction is required. However, during the time when the foot has to be placed in the correct position for heel strike, the brain stops the sensory signals from the calf muscles by inhibition of the relevant spinal pathways (Sinkjaer et al. 2000). In this way, these signals will not interfere with the placement of the heel on the ground. The ES was applied at a time when the patient attempted to dorsiflex the foot and was therefore task related and delivered within a meaningful context. However, a dorsiflexion will also impose a lengthening of the antagonist muscles (soleus and the two heads of the gastrocnemius), which would generate afferent signals arising from those muscles that travel toward the brain. Indeed, the effects observed here might be more potent when applied during a walking task at the appropriate time of dorsiflexion (early swing phase) and with relevant additional input from afferents located in the antagonist and also the sole of the foot. In a recent experiment we have demonstrated the feasibility of detecting gait initiation from single-trial MRCPs (Jiang et al. 2014), setting the stage for such experiments.
The peripheral afferent volley induced comprised a single volley set to an intensity at the vicinity of MT. It is currently not known how signals arising from the periphery are attenuated as they are conveyed to the motor cortex. Indeed, functional electrical stimulation (FES) is another therapy that has been used specifically to target the TA muscle after stroke either alone (Burridge et al. 1997; Everaert et al. 2010; Knash et al. 2003; Lyons et al. 2002; Thompson and Stein 2004) or in combination with a BCI (Cincotti et al. 2012; Daly et al. 2009; Li et al. 2014). FES uses a higher-frequency and -intensity stimulation to alter the excitability of the cortical projections to the target muscle than that applied in the present study. The effects of FES are enhanced when the voluntary drive to the TA is augmented such as through an active contraction of the TA (Khaslavskaia and Sinkjaer 2005), suggesting that some form of coincident summation is also involved. It is possible that the effects of our intervention may be further enhanced when the peripheral stimulus is similar to FES in aspects of frequency and stimulus strength. Indeed, with only FES for a duration of 3–12 mo, walking speed was significantly increased by 24% in chronic stroke as well as multiple sclerosis patients when the stimulator was in the off position (Everaert et al. 2010).
Residual corticospinal tract integrity and improvement in function.
Past studies have suggested that residual corticospinal cord integrity is a predictor for recovery of upper limb function (Stinear et al. 2007; Tombari et al. 2004). In the present study, residual corticospinal integrity was not significantly correlated both to functional improvements and to changes in MEP size, which is in support of such studies targeting lower limb muscles (Dawes et al. 2008). Lower limb muscles have been suggested to be under less cortical control compared with upper limb muscles (Peters 1990). However, one exception is the TA muscle, which requires precise control during foot clearance in the late swing phase of human walking and has been shown to have a strong cortical contribution to its activation (Bawa et al. 2002; Brouwer and Qiao 1995; Petersen et al. 2003). Presence of MEPs in the TA but not in the larger quadriceps muscle group is a predictor of functional recovery (Hendricks et al. 2003b), so it was surprising that DTI data were not well correlated to functional improvements. Possible confounding factors include both the types of patients recruited as well as the low number of patients who took part in the scans.
Conclusions.
We have introduced an innovative BCI intervention for stroke rehabilitation, based on Hebbian principles of associativity (Hebb 1949). Different from other BCI protocols reported in the literature, it requires no subject training (all patients were BCI-naive prior to the study); the attempted movement does not need to have residual muscle control, and a small number of repetitions and sessions were sufficient to induce a significant effect. The results presented prove the possibility of using neurofeedback systems, based on BCI concepts, for efficient and targeted induction of plastic changes in the motor cortex in stroke therapy. Because of the efficacy of the intervention and the relatively simple equipment needed, the proposed approach opens a strong perspective for a clinically viable BCI therapy for patient populations to promote functional plasticity and to improve motor function after stroke.
GRANTS
This work was supported by the Danish Agency for Science and Technology and Innovation, by the European project BETTER (contract no. 247935), by the Bernstein Focus Neurotechnology Göttingen, and by Project No. 159070 of the Ministry of Education, Science and Technological Development (MESTD) of Serbia.
AUTHOR CONTRIBUTIONS
Author contributions: N.M.-K., N.J., V.K., A.P., S.R., K.D., and D.F. conception and design of research; N.M.-K., I.K.N., A.P., S.R., and M.D.-J. performed experiments; N.M.-K., N.J., A.J.T.S., I.K.N., M.D.-J., and F.A. analyzed data; N.M.-K., N.J., V.K., M.D.-J., F.A., and D.F. interpreted results of experiments; N.M.-K. prepared figures; N.M.-K., N.J., and D.F. drafted manuscript; N.M.-K., N.J., A.J.T.S., V.K., and D.F. edited and revised manuscript; N.M.-K., N.J., A.J.T.S., I.K.N., V.K., A.P., S.R., M.D.-J., F.A., K.D., and D.F. approved final version of manuscript.
ACKNOWLEDGMENTS
We thank all the patients for participating in this study as well as Mercy Lain, who helped with graphics (Fig. 1, A–C).
REFERENCES
- Ang KK, Guan C, Chua KS, Ang BT, Kuah C, Wang C, Phua KS, Chin ZY, Zhang H. A clinical study of motor imagery-based brain-computer interface for upper limb robotic rehabilitation. Conf Proc IEEE Eng Med Biol Soc 2009: 5981–5984, 2009. [Abstract] [Google Scholar]
- Ang KK, Guan C, Chua KS, Ang BT, Kuah C, Wang C, Phua KS, Chin ZY, Zhang H. Clinical study of neurorehabilitation in stroke using EEG-based motor imagery brain-computer interface with robotic feedback. Conf Proc IEEE Eng Med Biol Soc 2010: 5549–5552, 2010. [Abstract] [Google Scholar]
- Ang KK, Guan C, Phua KS, Wang C, Zhou L, Tang KY, Ephraim Joseph GJ, Kuah CW, Chua KS. Brain-computer interface-based robotic end effector system for wrist and hand rehabilitation: results of a three-armed randomized controlled trial for chronic stroke. Front Neuroeng 7: 30, 2014. [Europe PMC free article] [Abstract] [Google Scholar]
- Avanzino L, Tacchino A, Abbruzzese G, Quartarone A, Ghilardi MF, Bonzano L, Ruggeri P, Bove M. Recovery of motor performance deterioration induced by a demanding finger motor task does not follow cortical excitability dynamics. Neuroscience 174: 84–90, 2011. [Abstract] [Google Scholar]
- Bawa P, Chalmers GR, Stewart H, Eisen AA. Responses of ankle extensor and flexor motoneurons to transcranial magnetic stimulation. J Neurophysiol 88: 124–132, 2002. [Abstract] [Google Scholar]
- Bohannon RW, Smith MB. Interrater reliability of a modified Ashworth scale of muscle spasticity. Phys Ther 67: 2: 206–207, 1987. [Abstract] [Google Scholar]
- Bonita R, Beaglehole R. Recovery of motor function after stroke. Stroke 19: 1497–1500, 1988. [Abstract] [Google Scholar]
- Broetz D, Braun C, Weber C, Soekadar SR, Caria A, Birbaumer N. Combination of brain-computer interface training and goal-directed physical therapy in chronic stroke: a case report. Neurorehabil Neural Repair 24: 7: 674–679, 2010. [Abstract] [Google Scholar]
- Brouwer B, Qiao J. Characteristics and variability of lower limb motoneuron responses to transcranial magnetic stimulation. Electroencephalogr Clin Neurophysiol 97: 49–54, 1995. [Abstract] [Google Scholar]
- Brown CE, Aminoltejari K, Erb H, Winship IR, Murphy TH. In vivo voltage-sensitive dye imaging in adult mice reveals that somatosensory maps lost to stroke are replaced over weeks by new structural and functional circuits with prolonged modes of activation within both the peri-infarct zone and distant sites. J Neurosci 29: 1719–1734, 2009. [Abstract] [Google Scholar]
- Buch E, Weber C, Cohen LG, Braun C, Dimyan MA, Ard T, Mellinger J, Caria A, Soekadar S, Fourkas A, Birbaumer N. Think to move: a neuromagnetic brain-computer interface (BCI) system for chronic stroke. Stroke 39: 910–917, 2008. [Abstract] [Google Scholar]
- Burridge JH, Taylor PN, Hagan SA, Wood DE, Swain ID. The effects of common peroneal stimulation on the effort and speed of walking: a randomized controlled trial with chronic hemiplegic patients. Clin Rehabil 11: 201–210, 1997. [Abstract] [Google Scholar]
- Chen Y, Chen L, Liu R, Wang Y, Chen XY, Wolpaw JR. Locomotor impact of beneficial or nonbeneficial H-reflex conditioning after spinal cord injury. J Neurophysiol 111: 1249–1258, 2014. [Europe PMC free article] [Abstract] [Google Scholar]
- Cincotti F, Pichiorri F, Arico P, Aloise F, Leotta F, de Vico Fallani F, Millan Jdel R, Molinari M, Mattia D. EEG-based brain-computer interface to support post-stroke motor rehabilitation of the upper limb. Conf Proc IEEE Eng Med Biol Soc 2012: 4112–4115, 2012. [Abstract] [Google Scholar]
- Daly JJ, Cheng R, Rogers J, Litinas K, Hrovat K, Dohring M. Feasibility of a new application of noninvasive brain computer interface (BCI): a case study of training for recovery of volitional motor control after stroke. J Neurol Phys Ther 33: 203–211, 2009. [Abstract] [Google Scholar]
- Dawes H, Enzinger C, Johansen-Berg H, Bogdanovic M, Guy C, Collett J, Izadi H, Stagg C, Wade D, Matthews P. Walking performance and its recovery in chronic stroke in relation to extent of lesion overlap with the descending motor tract. Exp Brain Res 186: 325–333, 2008. [Abstract] [Google Scholar]
- Djuric-Jovicic MD, Jovicic NS, Popovic DB. Kinematics of gait: new method for angle estimation based on accelerometers. Sensors (Basel) 11: 10571–10585, 2011. [Europe PMC free article] [Abstract] [Google Scholar]
- Everaert DG, Thompson AK, Su LC, Stein RB. Does functional electrical stimulation for foot drop strengthen corticospinal connections? Neurorehabil Neural Repair 24: 168–177, 2010. [Abstract] [Google Scholar]
- Fugl-Meyer AR, Jaasko L, Leyman I, Olsson S, Steglind S. The post-stroke hemiplegic patient. 1. A method for evaluation of physical performance. Scand J Rehabil Med 7: 13–31, 1975. [Abstract] [Google Scholar]
- Grey MJ, Mazzaro N, Nielsen JB, Sinkjaer T. Ankle extensor proprioceptors contribute to the enhancement of the soleus EMG during the stance phase of human walking. Can J Physiol Pharmacol 82: 610–616, 2004. [Abstract] [Google Scholar]
- Hamilton M. Development of a rating scale for primary depressive illness. Br J Soc Clin Psychol 6: 278–296, 1967. [Abstract] [Google Scholar]
- Hebb DO. The Organization of Behavior: a Neuropsychological Theory. Mahwah, NJ: Erlbaum, 1949. [Google Scholar]
- Hendricks HT, Pasman JW, Merx JL, van Limbeek J, Zwarts MJ. Analysis of recovery processes after stroke by means of transcranial magnetic stimulation. J Clin Neurophysiol 20: 188–195, 2003a. [Abstract] [Google Scholar]
- Hendricks HT, Pasman JW, van Limbeek J, Zwarts MJ. Motor evoked potentials of the lower extremity in predicting motor recovery and ambulation after stroke: a cohort study. Arch Phys Med Rehabil 84: 1373–1379, 2003b. [Abstract] [Google Scholar]
- Heremans E, Helsen WF, De Poel HJ, Alaerts K, Meyns P, Feys P. Facilitation of motor imagery through movement-related cueing. Brain Res 1278: 50–58, 2009. [Abstract] [Google Scholar]
- Jiang N, Gizzi L, Mrachacz-Kersting N, Dremstrup K, Farina D. A brain-computer interface for single-trial detection of gait initiation from movement related cortical potentials. Clin Neurophysiol 126: 154–159, 2014. [Abstract] [Google Scholar]
- Kasashima-Shindo Y, Fujiwara T, Ushiba J, Matsushika Y, Kamatani D, Oto M, Ono T, Nishimoto A, Shindo K, Kawakami M, Tsuji T, Liu M. Brain-computer interface training combined with transcranial direct current stimulation in patients with chronic severe hemiparesis: proof of concept study. J Rehabil Med 47: 318–324, 2015. [Abstract] [Google Scholar]
- Khaslavskaia S, Sinkjaer T. Motor cortex excitability following repetitive electrical stimulation of the common peroneal nerve depends on the voluntary drive. Exp Brain Res 162: 497–502, 2005. [Abstract] [Google Scholar]
- Knash M, Kido A, Gorassini M, Chan KM, Stein R. Electrical stimulation of the human common peroneal nerve elicits lasting facilitation of cortical motor-evoked potentials. Exp Brain Res 153: 366–377, 2003. [Abstract] [Google Scholar]
- Kornhuber HH, Deecke L. Changes in the brain potential in voluntary movements and passive movements in man: readiness potential and reafferent potential. Pflügers Arch 284: 1–17, 1965. [Abstract] [Google Scholar]
- Kuhtz-Buschbeck JP, Mahnkopf C, Holzknecht C, Siebner H, Ulmer S, Jansen O. Effector-independent representations of simple and complex imagined finger movements: a combined fMRI and TMS study. Eur J Neurosci 18: 3375–3387, 2003. [Abstract] [Google Scholar]
- Kumpulainen S, Mrachacz-Kersting N, Peltonen J, Voigt M, Avela J. The optimal interstimulus interval and repeatability of paired associative stimulation when the soleus muscle is targeted. Exp Brain Res 221: 241–249, 2012. [Abstract] [Google Scholar]
- Leifert-Fiebach G, Welfringer A, Babinsky R, Brandt T. Motor imagery training in patients with chronic neglect: A pilot study. NeuroRehabilitation 32: 43–58, 2013. [Abstract] [Google Scholar]
- Li M, Liu Y, Wu Y, Liu S, Jia J, Zhang L. Neurophysiological substrates of stroke patients with motor imagery-based brain-computer interface training. Int J Neurosci 124: 403–415, 2014. [Abstract] [Google Scholar]
- Lyden PD, Lu M, Levine SR, Brott TG, Broderick J., NINDS rtPA Stroke Study Group. A modified National Institutes of Health Stroke Scale for use in stroke clinical trials: preliminary reliability and validity. Stroke 32: 1310–1317, 2001. [Abstract] [Google Scholar]
- Lyons GM, Sinkjaer T, Burridge JH, Wilcox DJ. A review of portable FES-based neural orthoses for the correction of drop foot. IEEE Trans Neural Syst Rehabil Eng 10: 260–279, 2002. [Abstract] [Google Scholar]
- Mazzaro N, Grey M, do Nascimento O, Sinkjaer T. Afferent-mediated modulation of the soleus muscle activity during the stance phase of human walking. Exp Brain Res 173: 713–723, 2006. [Abstract] [Google Scholar]
- McFarland DJ, McCane LM, David SV, Wolpaw JR. Spatial filter selection for EEG-based communication. Electroencephalogr Clin Neurophysiol 103: 386–394, 1997. [Abstract] [Google Scholar]
- Mrachacz-Kersting N, Fong M, Murphy BA, Sinkjaer T. Changes in excitability of the cortical projections to the human tibialis anterior after paired associative stimulation. J Neurophysiol 97: 1951–1958, 2007. [Abstract] [Google Scholar]
- Mrachacz-Kersting N, Kristensen SR, Niazi IK, Farina D. Precise temporal association between cortical potentials evoked by motor imagination and afference induces cortical plasticity. J Physiol 590: 1669–1682, 2012a. [Abstract] [Google Scholar]
- Mrachacz-Kersting N, Niazi IK, Jiang N, Pavlovic AM, Radovanovic S, Kostiç VS, Popovic DB, Dremstrup K, Farina D. A novel brain-computer interface for chronic stroke patients. In: Biosystems and Biorobotics: International Conference on Neurorehabilitation, Berlin: Springer, 2012b, p. 837–841. [Google Scholar]
- Mukaino M, Ono T, Shindo K, Fujiwara T, Ota T, Kimura A, Liu M, Ushiba J. Efficacy of brain-computer interface-driven neuromuscular electrical stimulation for chronic paresis after stroke. J Rehabil Med 46: 378–382, 2014. [Abstract] [Google Scholar]
- Murphy TH, Corbett D. Plasticity during stroke recovery: from synapse to behaviour. Nat Rev Neurosci 10: 861–872, 2009. [Abstract] [Google Scholar]
- Niazi IK, Jiang N, Tiberghien O, Feldbæk-Nielsen J, Dremstrup K, Farina D. Detection of movement intention from single-trial movement-related cortical potentials. J Neural Eng 8: 066009, 2011. [Abstract] [Google Scholar]
- Niazi IK, Mrachacz-Kersting N, Jiang N, Dremstrup K, Farina D. Peripheral electrical stimulation triggered by self-paced detection of motor intention enhances motor evoked potentials. IEEE Trans Neural Syst Rehabil Eng 20: 595–604, 2012. [Abstract] [Google Scholar]
- Nielsen JB. How we walk: central control of muscle activity during human walking. Neuroscientist 9: 195–204, 2003. [Abstract] [Google Scholar]
- Nielsen JB. Sensorimotor integration at spinal level as a basis for muscle coordination during voluntary movement in humans. J Appl Physiol 96: 1961–1967, 2004. [Abstract] [Google Scholar]
- Nielsen JB, Sinkjaer T. Afferent feedback in the control of human gait. J Electromyogr Kinesiol 12: 213–217, 2002. [Abstract] [Google Scholar]
- Ono T, Shindo K, Kawashima K, Ota N, Ito M, Ota T, Mukaino M, Fujiwara T, Kimura A, Liu M, Ushiba J. Brain-computer interface with somatosensory feedback improves functional recovery from severe hemiplegia due to chronic stroke. Front Neuroeng 7: 19, 2014. [Europe PMC free article] [Abstract] [Google Scholar]
- Pascual-Leone A, Nguyet D, Cohen LG, Brasil-Neto JP, Cammarota A, Hallett M. Modulation of muscle responses evoked by transcranial magnetic stimulation during the acquisition of new fine motor skills. J Neurophysiol 74: 1037–1045, 1995. [Abstract] [Google Scholar]
- Pavlides C, Miyashita E, Asanuma H. Projection from the sensory to the motor cortex is important in learning motor skills in the monkey. J Neurophysiol 70: 733–741, 1993. [Abstract] [Google Scholar]
- Perez MA, Lungholt BK, Nyborg K, Nielsen JB. Motor skill training induces changes in the excitability of the leg cortical area in healthy humans. Exp Brain Res 159: 197–205, 2004. [Abstract] [Google Scholar]
- Peters M. Neuropsychological identification of motor problems: can we learn something from the feet and legs that hands and arms will not tell us? Neuropsychol Rev 1: 165–183, 1990. [Abstract] [Google Scholar]
- Petersen NT, Pyndt HS, Nielsen JB. Investigating human motor control by transcranial magnetic stimulation. Exp Brain Res 152: 1–16, 2003. [Abstract] [Google Scholar]
- Pichiorri F, Morone G, Petti M, Toppi J, Pisotta I, Molinari M, Paolucci S, Inghilleri M, Astolfi L, Cincotti F, Mattia D. Brain-computer interface boosts motor imagery practice during stroke recovery. Ann Neurol 77: 851–865, 2015. [Abstract] [Google Scholar]
- Piron L, Piccione F, Tonin P, Dam M. Clinical correlation between motor evoked potentials and gait recovery in poststroke patients. Arch Phys Med Rehabil 86: 1874–1878, 2005. [Abstract] [Google Scholar]
- Platz T, Kim IH, Pintschovius H, Winter T, Kieselbach A, Villringer K, Kurth R, Mauritz KH. Multimodal EEG analysis in man suggests impairment-specific changes in movement-related electric brain activity after stroke. Brain 123: 2475–2490, 2000. [Abstract] [Google Scholar]
- Ramos-Murguialday A, Broetz D, Rea M, Laer L, Yilmaz O, Brasil FL, Liberati G, Curado MR, Garcia-Cossio E, Vyziotis A, Cho W, Agostini M, Soares E, Soekadar S, Caria A, Cohen LG, Birbaumer N. Brain-machine interface in chronic stroke rehabilitation: a controlled study. Ann Neurol 74: 100–108, 2013. [Europe PMC free article] [Abstract] [Google Scholar]
- Salinet AS, Robinson TG, Panerai RB. Reproducibility of cerebral and peripheral haemodynamic responses to active, passive and motor imagery paradigms in older healthy volunteers: a fTCD study. J Neurosci Methods 206: 143–150, 2012. [Abstract] [Google Scholar]
- Silvoni S, Ramos-Murguialday A, Cavinato M, Volpato C, Cisotto G, Turolla A, Piccione F, Birbaumer N. Brain-computer interface in stroke: a review of progress. Clin EEG Neurosci 42: 245–252, 2011. [Abstract] [Google Scholar]
- Sinkjaer T, Andersen JB, Ladouceur M, Christensen LO, Nielsen JB. Major role for sensory feedback in soleus EMG activity in the stance phase of walking in man. J Physiol 523: 817–827, 2000. [Abstract] [Google Scholar]
- Song J, Young BM, Nigogosyan Z, Walton LM, Nair VA, Grogan SW, Tyler ME, Farrar-Edwards D, Caldera KE, Sattin JA, Williams JC, Prabhakaran V. Characterizing relationships of DTI, fMRI, and motor recovery in stroke rehabilitation utilizing brain-computer interface technology. Front Neuroeng 7: 31, 2014. [Europe PMC free article] [Abstract] [Google Scholar]
- Stefan K, Kunesch E, Benecke R, Cohen LG, Classen J. Mechanisms of enhancement of human motor cortex excitability induced by interventional paired associative stimulation. J Physiol 543: 699–708, 2002. [Abstract] [Google Scholar]
- Stinear CM, Barber PA, Smale PR, Coxon JP, Fleming MK, Byblow WD. Functional potential in chronic stroke patients depends on corticospinal tract integrity. Brain 130: 170–180, 2007. [Abstract] [Google Scholar]
- Teo WP, Chew E. Is motor-imagery brain-computer interface feasible in stroke rehabilitation? PM R 6: 723–728, 2014. [Abstract] [Google Scholar]
- Thompson AK, Stein RB. Short-term effects of functional electrical stimulation on motor-evoked potentials in ankle flexor and extensor muscles. Exp Brain Res 159: 491–500, 2004. [Abstract] [Google Scholar]
- Thompson AK, Wolpaw JR. Restoring walking after spinal cord injury: operant conditioning of spinal reflexes can help. Neuroscientist 21: 203–215, 2015. [Europe PMC free article] [Abstract] [Google Scholar]
- Tombari D, Loubinoux I, Pariente J, Gerdelat A, Albucher JF, Tardy J, Cassol E, Chollet F. A longitudinal fMRI study: in recovering and then in clinically stable sub-cortical stroke patients. Neuroimage 23: 827–839, 2004. [Abstract] [Google Scholar]
- Walter WG, Cooper R, Aldridge VJ, McCallum WC, Winter AL. Contingent negative variation: an electric sign of sensorimotor association and expectancy in the human brain. Nature 203: 380–384, 1964. [Abstract] [Google Scholar]
- Wolpaw JR. Spinal cord plasticity in acquisition and maintenance of motor skills. Acta Physiol 189: 155–169, 2007. [Abstract] [Google Scholar]
- Xu R, Jiang N, Mrachacz-Kersting N, Lin C, Asin Prieto G, Moreno JC, Pons JL, Dremstrup K, Farina D. A closed-loop brain-computer interface triggering an active ankle-foot orthosis for inducing cortical neural plasticity. IEEE Trans Biomed Eng 61: 2092–2101, 2014. [Abstract] [Google Scholar]
- Yilmaz O, Cho W, Braun C, Birbaumer N, Ramos-Murguialday A. Movement related cortical potentials in severe chronic stroke. Conf Proc IEEE Eng Med Biol Soc 2013: 2216–2219, 2013. [Abstract] [Google Scholar]
- Young BM, Nigogosyan Z, Walton LM, Song J, Nair VA, Grogan SW, Tyler ME, Edwards DF, Caldera K, Sattin JA, Williams JC, Prabhakaran V. Changes in functional brain organization and behavioral correlations after rehabilitative therapy using a brain-computer interface. Front Neuroeng 7: 26, 2014. [Europe PMC free article] [Abstract] [Google Scholar]
Articles from Journal of Neurophysiology are provided here courtesy of American Physiological Society
Full text links
Read article at publisher's site: https://doi.org/10.1152/jn.00918.2015
Read article for free, from open access legal sources, via Unpaywall:
https://europepmc.org/articles/pmc4808132
Citations & impact
Impact metrics
Article citations
Brain-spine interface for movement restoration after spinal cord injury.
Brain Spine, 4:102926, 10 Aug 2024
Cited by: 0 articles | PMID: 39247724 | PMCID: PMC11379558
Sensing and Control Strategies Used in FES Systems Aimed at Assistance and Rehabilitation of Foot Drop: A Systematic Literature Review.
J Pers Med, 14(8):874, 17 Aug 2024
Cited by: 0 articles | PMID: 39202064 | PMCID: PMC11355777
Review Free full text in Europe PMC
A single-center, assessor-blinded, randomized controlled clinical trial to test the safety and efficacy of a novel brain-computer interface controlled functional electrical stimulation (BCI-FES) intervention for gait rehabilitation in the chronic stroke population.
BMC Neurol, 24(1):200, 13 Jun 2024
Cited by: 0 articles | PMID: 38872109 | PMCID: PMC11170800
Characterization of Event Related Desynchronization in Chronic Stroke Using Motor Imagery Based Brain Computer Interface for Upper Limb Rehabilitation.
Ann Indian Acad Neurol, 27(3):297-306, 01 May 2024
Cited by: 0 articles | PMID: 38835164 | PMCID: PMC11232817
Attentional state-synchronous peripheral electrical stimulation during action observation induced distinct modulation of corticospinal plasticity after stroke.
Front Neurosci, 18:1373589, 28 Mar 2024
Cited by: 0 articles | PMID: 38606309 | PMCID: PMC11007104
Go to all (88) article citations
Similar Articles
To arrive at the top five similar articles we use a word-weighted algorithm to compare words from the Title and Abstract of each citation.
The effect of type of afferent feedback timed with motor imagery on the induction of cortical plasticity.
Brain Res, 1674:91-100, 30 Aug 2017
Cited by: 15 articles | PMID: 28859916
A closed-loop brain-computer interface triggering an active ankle-foot orthosis for inducing cortical neural plasticity.
IEEE Trans Biomed Eng, 61(7):2092-2101, 26 Mar 2014
Cited by: 63 articles | PMID: 24686231
Peripheral electrical stimulation triggered by self-paced detection of motor intention enhances motor evoked potentials.
IEEE Trans Neural Syst Rehabil Eng, 20(4):595-604, 25 Apr 2012
Cited by: 58 articles | PMID: 22547461
A review of the progression and future implications of brain-computer interface therapies for restoration of distal upper extremity motor function after stroke.
Expert Rev Med Devices, 13(5):445-454, 01 May 2016
Cited by: 44 articles | PMID: 27112213 | PMCID: PMC5131699
Review Free full text in Europe PMC
Funding
Funders who supported this work.
Danish Agency for Science and Technology (1)
Grant ID: na
EU project BETTER (1)
Grant ID: 247935
MESTD of Serbia (1)
Grant ID: 159070