Abstract
Free full text

AMPK/α-ketoglutarate axis dynamically mediates DNA demethylation in the Prdm16 promoter and brown adipogenesis
SUMMARY
Promoting brown adipose tissue (BAT) development is an attractive strategy for the treatment of obesity, as activated BAT dissipates energy through thermogenesis; however, the mechanisms controlling BAT formation are not fully understood. We hypothesized that as a master regulator of energy metabolism, AMP-activated protein kinase (AMPK) may play a direct role in the process and found that AMPKα1 (PRKAA1) ablation reduced Prdm16 expression and impaired BAT development. During early brown adipogenesis, the cellular levels of α-ketoglutarate (α-KG), a key metabolite required for TET-mediated DNA demethylation, were profoundly increased and required for active DNA demethylation of the Prdm16 promoter. AMPKα1 ablation reduced isocitrate dehydrogenase 2 activity and cellular α-KG levels. Remarkably, postnatal AMPK activation with AICAR or metformin rescued obesity-induced suppression of brown adipogenesis and thermogenesis. In summary, AMPK is essential for the epigenetic control of BAT development through α-ketoglutarate, thus linking a metabolite to progenitor cell differentiation and thermogenesis.
INTRODUCTION
Brown adipose tissue (BAT) burns fatty acids and glucose to generate heat (Virtanen et al., 2009), providing a promising therapeutic target against obesity and type 2 diabetes (T2D). During brown adipogenesis, PR Domain Containing 16 protein (PRDM16) not only commits progenitors to the brown adipogenic lineage, but is also required for maintaining brown adipocyte identity (Seale et al., 2008). However, the regulation of Prdm16 at the transcriptional level remains largely unexplored. Prdm16 is enriched with CpG sites surrounding its transcription start site (TSS), a characteristic of ‘key developmental genes’ (Meissner et al., 2008). Promoters of key developmental genes are enriched with both H3K4me3 and H3K27me3, forming a ‘bivalent state’ (Mikkelsen et al., 2007b). During early differentiation, non-induced bivalent genes in progenitors preserve repressive H3K27me3 mark (Schuettengruber and Cavalli, 2009), converting to relatively stable inhibition by inducing DNA methylation (Mohn et al., 2008). Thus, active DNA demethylation is likely needed for activating their expression during subsequent differentiation. Active DNA demethylation is mediated by the ten-eleven translocation hydroxylases (TETs), including TET1, 2 and 3 (Ficz et al., 2011; Ito et al., 2010). Importantly, TET catalytic reaction requires α-ketoglutarate (αKG), a key metabolite of the Krebs cycle, linking metabolism to epigenetic modifications (Wu and Zhang, 2014) and stem cell differentiation (Wu et al., 2011b).
As a master regulator of cell metabolism, AMP-activated protein kinase (AMPK) positions as a key mediator of cell differentiation (Hardie, 2011). Recently, AMPK was proposed to regulate brown fat (BAT) thermogenic function indirectly via altering hypothalamus and adrenergic nervous system in adults (Martinez de Morentin et al., 2014; Whittle et al., 2012). Here, we hypothesized that AMPK directly regulates brown adipogenesis through elevating αKG production and DNA demethylation in the Prdm16 promoter of progenitor cells, and AMPK is required for effective brown adipogenesis.
Obesity is an epidemic problem and well known to suppress AMPK activity (Steinberg et al., 2006). We found that obesity inhibits AMPK activity, which reduces αKG concentration and impedes Prdm16 expression, resulted in impaired BAT development and thermogenic function. Moreover, AMPK activation in obese mice, using the common anti-diabetic drug metformin, rescued BAT development and its thermogenic capacity. These studies link AMPK and BAT development to αKG and to the subsequent TET-mediated DNA demethylation of the Prdm16 promoter. Furthermore, they suggest that AMPK may be an excellent drug target for promoting BAT development and for treating obesity.
RESULTS
Brown adipogenesis is impeded due to Prkaa1 ablation
The catalytic unit of AMPK has two isoforms, α1 (PRKAA1) and α2. We found that Prkaa1 is prominently expressed in brown progenitor cells (Figure S1A, S1B) in a manner resembling its expression pattern during white adipogenesis (Daval et al., 2005). In Prkaa1−/− mice, the BAT mass was smaller than in wild-type (WT) mice (Figures S1D, S1E), which was associated with a lower density of Lin-/CD45-/Sca-1+ progenitor cells and reduced Ki67+ cells (Figures S1F–S1I). The dorsal interscapular temperature (DIT) of Prkaa1−/− mice was convincingly lower than that of neonatal WT mice (Figure 1A), concomitant with reduced UCP1 content (Figure 1B). Consistently, the Prdm16 expression was attenuated in Prkaa1−/− E15.5 BAT (Figure S1K, S1L), a critical stage for early brown adipocyte commitment (Schulz et al., 2013). We then examined BAT cellular structure using electron microscopy and determined that although the number of intracellular lipid droplets was lower in Prkaa1−/− mice, cell size was larger and lipid droplets were less uniformly distributed compared to those in Prkaa1+/+ cells (Figure 1C, S1M). Mitochondrial density was also lower in Prkaa1−/− mice and there was evidence of poorly organized cristae (Figure 1D, S1J). Furthermore, the mRNA expression of markers for brown adipogenesis was reduced (Figure 1E). When subjected to the cold environment for short durations, the body temperature of Prkaa1−/− adult mice was notably lower than that of WT mice that were similarly exposed (Figure S2A). The long-term cold stimulus (11 days), however, did not change body temperature, consistent with a previous report (Bauwens et al., 2011). Long-term cold exposure induces a compensatory thermal adaptation that might mask the effect of BAT deficiency on thermostasis (Schulz et al., 2013). Stimulating cells in vitro with the b-adrenergic agonist isoproterenol up-regulates Ucp1 expression in both WT and Prkaa1−/− BAT, with responsiveness to be much weaker in the latter (Figure S2B). Cold challenge substantially reduced lipid droplet size in BAT of WT mice, but not in Prkaa1−/− BAT (Figure S2C). Consistently, in vivo, the Prkaa1 deleted BAT was less responsive to the challenge of high fat diet (HFD) or cold stimulus (6 h at 5 °C) (Figure S2D). Together, lipid utilization and thermogenesis in BAT of Prkaa1−/− mice was impaired. We therefore analyzed the AMP and ATP contents in both progenitor cells and BAT. In progenitor cells, acute Prkaa1 KO increased the AMP/ATP ratio, indicative of impaired energy status. Surprisingly, the AMP level was very high in BAT of young mice, which was likely due to highly active uncoupling, which is consistent with previous reports (Drahota et al., 1970; Pedersen and Grav, 1972). The AMP/ATP ratio was also lower in Prkaa1−/− BAT, showing attenuated uncoupling and BAT function (Figure S2E, S2F). These data clearly demonstrate the impairment of BAT function in the absence of Prkaa1.

(A) Body temperature of WT, heterozygous and Prkaa1 KO mice at P1. (B) Ucp1 expression at P1 (n = 4). (C) Scanning electron microscopic images of BAT at P21 (Scale bar, 50µm). (D) Transmission electron microscopic images of BAT from P21 mice (Scale bar, 5µm and 500 nm). (E) Expression of BAT markers and selected markers of BAT separated from WT and Prkaa1 KO mice at P1 (n = 5). (F–H) Neonatal BAT was isolated from Prkaa1 floxed mice with/without Cre and transplanted into a recipient mouse, and Prkaa1 KO was induced by tamoxifen injection (Figure S3A for the detailed protocol). At 21 days after transplantation, the thermogenesis of transplanted BAT from neonatal Prkaa1 KO mice (right side) was severely impaired compared to WT BAT (left side) (F); the difference in the structure of transplanted BAT (G, Scale bar, 100µm); mRNA expression of brown adipocyte markers was profoundly reduced in transplanted BAT due to Prkaa1 deficiency (H, n = 3). Gene mRNA expression was normalization to the 18S rRNA. (*P < 0.05; **P < 0.01; ***P < 0.001; means ± s.e.m.; Student’s t test with a two-tailed distribution; one-way ANOVA with Tukey’s post hoc test was used in B.)
Because AMPK is capable of regulating BAT function indirectly through the sympathetic nervous system (Martinez de Morentin et al., 2014; Whittle et al., 2012), we performed BAT transplantation and acute deletion of Prkaa1 in progenitor cells to demonstrate that endogenous PRKAA1 is critically required for brown adipogenesis independent of sympathetic control. First, we demonstrated that Cre recombinase expression or 4-hydroxytamoxifen (0.25 µM) treatment did not affect brown adipogenesis (Figure S1C), as previously reported (Cohen et al., 2014). Then, BAT was isolated from neonatal Prkaa1flox/flox/ER-Cre−/− and Prkaa1flox/flox/ER-Cre+/− mice of the same litter and transplanted into WT recipient mice; after 21 days, the transplanted BAT grew and integrated into the BAT niche (Figure S3A–S3C). Compared to WT BAT, the development of transplanted Prkaa1−/− BAT and its thermogenic capacity were severely hampered (Figure 1F, S3D, S3E); as a negative control, transplanted visceral fat did not generate heat (Figure S3F). Histologically, Prkaa1 KO rendered transplanted BAT structurally similar to that of white adipose tissue (WAT) (Figure 1G) with reduced expression of brown adipocyte markers (Figure 1H), underscoring the importance of Prkaa1 in brown adipogenesis which is independent of other physiological influences.
During early development, brown adipocytes are originated from progenitor cells of several different cell lineages (Peirce et al., 2014; Tran et al., 2012) in addition to the well-known Myf5+ lineage (Seale et al., 2008). We therefore used mouse embryonic fibroblasts (MEFs) and brown stromal vascular cells (BSVs) so that likely sources of progenitors were included. We isolated BSVs from neonatal Prkaa1flox/flox/ER-Cre+/− mice, treated with/without 4-hydroxytamoxifen, and induced brown adipogenesis. Prkaa1 KO profoundly reduced the formation of brown adipocytes and mitochondrial abundance (Figure 2A–D) as well as oxygen consumption and expression of brown adipogenic markers (Figure 2E, 2F). We further prepared E13.5 MEFs. In inducible Prkaa1 KO MEFs, fewer brown adipocytes were detected by Oil-Red-O and UCP1 staining while glucose uptake was compromised (Figure S4A–S4D). Transfecting C3H10T1/2 cells with Prkaa1/Cas9 plasmid prevented differentiation into brown adipocytes as cells with green fluorescence (Prkaa1 KO) remained spindle shape, while control cells (Scrambled/Cas9) became round shape, indicative of brown adipogenesis (Figure 2G). These results unambiguously demonstrate the necessity of PRKAA1 in brown adipogenesis. The differential brown adipogenesis was further confirmed by Oil-Red-O staining, and attenuated PRDM16 and UCP1 expression (Figure 2H, 2I). However, Prkaa1 KO after the commitment stage had no effect on the number of brown adipocytes but changed the morphology of mature adipocytes (Figure S4E–S4G).
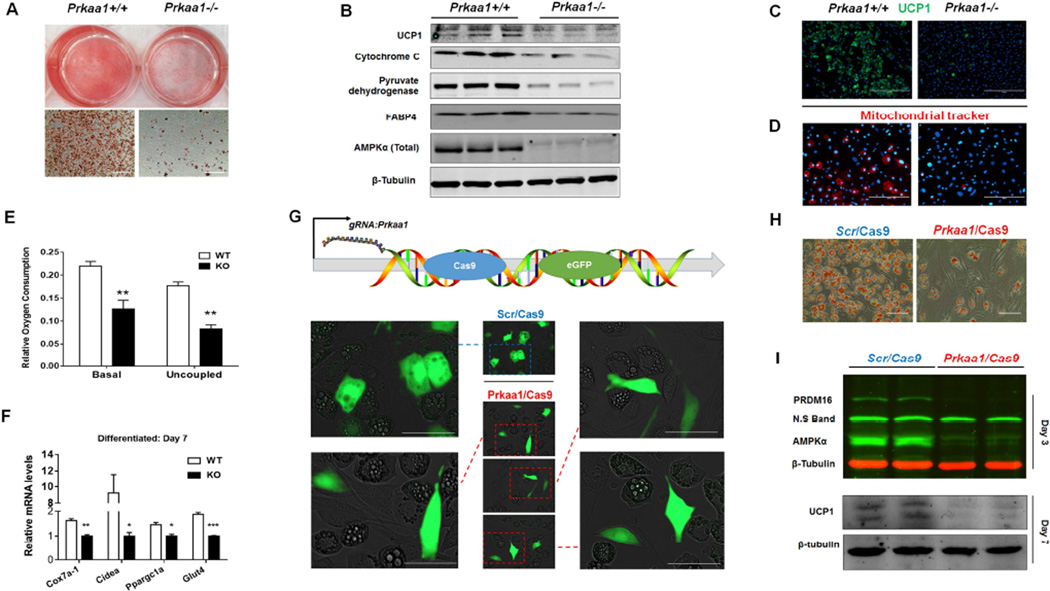
(A–F) Brown stromal vascular cells (BSVs) were prepared from the brown adipose tissue of Prkaa1flox/flox /ER-Cre neonatal mice, and Prkaa1 KO was induced by 4-hydroxytamoxifen (4-HTO) after reaching confluence. Oil-red-O staining of BSVs at 7 days after inducing brown adipogenesis (A, Scale bar, 400µm); immunoblotting of markers of BAT and mitochondria (B); immunofluorescence staining of UCP1 after inducing brown adipogenesis (Scale bar, 400µm) (C); mitochondrial Tracker (Scale bar, 200µm) (D); oxygen consumption of differentiated brown adipocytes from WT and KO BSVs (E); mRNA expression of related markers of brown adipocytes (F). (G–I) Deleting Prkaa1 by CRISPR/Cas9 genome editing blocked brown adipogenesis. C3H10T1/2 cells were transfected with scrambled (Scr/Cas9) and Prkaa1 CRISPR/Cas9 plasmids respectively, and then induced brown adipogenesis. Transfected cells (Green fluorescence) with Prkaa1 ablation could not convert to brown adipocytes (remained spindle shape), while control cells (Scrambled/Cas9) became brown adipocytes (round shape) (G, Scale bar, 100µm); Less brown adipocytes were detected by Oil-Red-O staining at day 7 (H, Scale bar, 200µm); Prkaa1 ablation reduced PRDM16 and UCP1 protein contents (I). Gene mRNA expression was normalization to the 18S rRNA. (**P < 0.01; ***P < 0.001; mean ± s.e.m.; n = 3; Student’s t test with a two-tailed distribution.)
Because a portion of brown progenitor cells is myogenic (Seale et al., 2008), we induced myogenesis of BSVs isolated from E18.5 fetuses, when BAT is actively developing. Myogenin, a marker of myogenic differentiation, was much more abundant in Prkaa1−/− cells (Figure S4H). The shape of cells was primarily round in WT cells, while Prkaa1−/− cells formed long tubes (Figure S4I) and stained positive for myosin heavy chain (MHC) (Figure S4J). Consistently, MHC content was higher in the BAT of Prkaa1−/− E18.5 fetuses (Figure S4K). We also analyzed the expression of white adipogenic markers during early brown adipogenesis, which was not affected by Prkaa1 loss (Figure S4L, S4M). Thus, BSVs from Prkaa1−/− mice possess a higher capacity to undergo myogenesis.
DNA demethylation in the Prdm16 promoter is impaired due to Prkaa1 ablation
Based on recent ChIP-sequencing data (Mikkelsen et al., 2007a), the region surrounding Prdm16 promoter has a great abundance of H3K27me3 and H3K4me3, which colocalize with the CpG island, eliciting the bivalent status of Prdm16 promoter (Ku et al., 2008). Moreover, the core subunits of PRC2, EZH2 and SUZ12, colocalize with TET1 in the CpG island (Xu et al., 2011b) (Figure S5A). During brown adipogenic differentiation of BSVs, Prdm16 expression peaked at day 3 (Figure 3A), which was blunted due to Prkaa1 KO (Figure 3A, 3B). Moreover, Prdm16 over-expression in Prkaa1 KO cells restored brown adipogenesis (Figure 3C, 3D), showing the mediatory role of PRDM16.
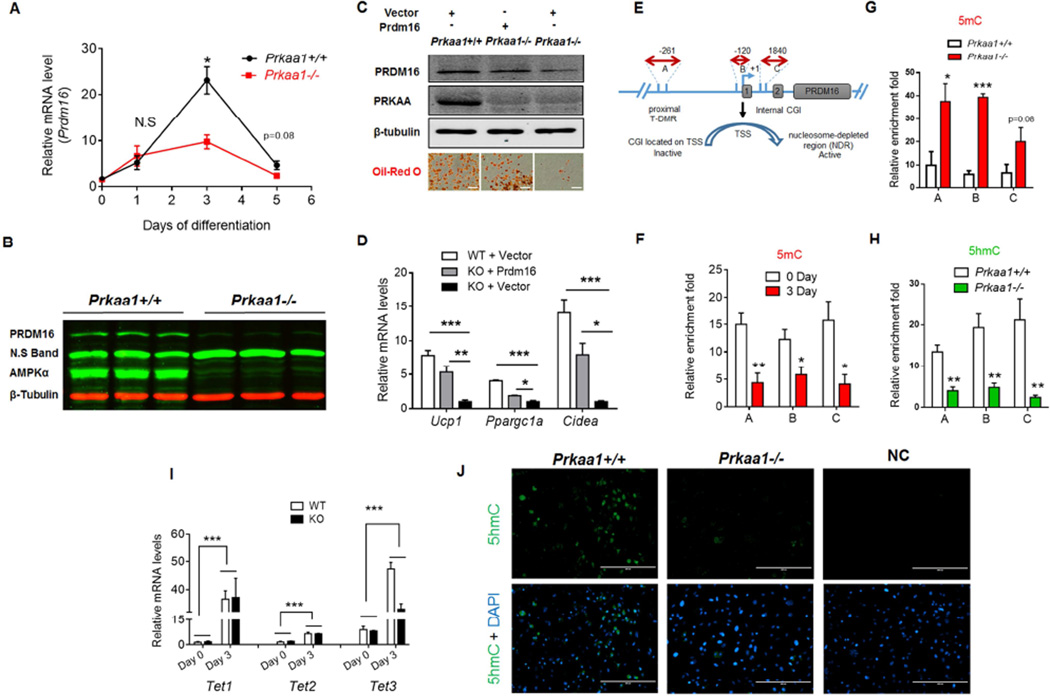
(A, B) The mRNA expression (A) and protein content (Day 3) (B) of Prdm16 in WT and Prkaa1 KO cells during brown adipogenic differentiation of brown stromal vascular cells (BSVs) (n = 3). (C, D) Prdm16 ectopic expression rescues brown adipogenesis impaired due to Prkaa1 ablation. BSVs were separated from Prkaa1 floxed mice (n = 4). Then, WT cells were transfected with eGFP plasmid, and Prkaa1 KO cells were transfected with either eGFP or Prdm16 over-expressing plasmid. Prdm16 over-expression increased PRDM16 content at day 3 of differentiation and brown adipogenesis stained by Oil-Red-O at day 7 (C, Scale bar, 200µm), and rescued expression of brown adipocyte markers in cells with PRKAA1 deficiency (D). (E) Diagram showing three amplicon regions in the Prdm16 gene, including proximal tissue specific-DNA methylation (T-DMR, A), a nucleosome-depleted region (NDR, B) and a GC island covered by rich epigenetic modifications (intergenic regulated region, C). (F) Reduction of DNA methylation in Prdm16 promoter of BSVs before and 3 days of differentiation (n = 5 for 0 d, n = 4 for 3 d). (G, H) Prkaa1 KO led to an increase of 5mC (G) and a reduction of 5hmC (H) in the Prdm16 promoter at day 3. (I) Tet1, 2 and 3 expression before and 3 days after brown adipogenic differentiation (n = 3). (J) Immunohistochemical staining of 5hmC at day 3 of differentiation (Scale bar, 200µm). Gene mRNA expression was normalization to the 18S rRNA; Immunoprecipitated target sequences were analyzed by qPCR and normalized to inputs. (*P < 0.05; **P < 0.01; ***P < 0.001; mean ± s.e.m.; n = 5 unless otherwise indicated; Student’s t test with a two-tailed distribution.)
To explore responsible epigenetic changes reducing Prdm16 expression, we focused on three specific regions in the Prdm16 promoter, including tissue specific DNA methylation region (T-DMR) (Kron et al., 2013; Tan et al., 2014), the transcription start site (TSS) which is located in the nucleosome-depleted region (NDR) (Jones, 2012), and a GC-rich area in the gene body covered by rich epigenetic modifications (Figure 3E). Consistent with increased Prdm16 expression, the DNA methylation in these three regions was reduced during differentiation (Figure 3F), showing the occurrence of DNA demethylation. A recent global genomic mapping study found that key developmental genes are regularly enriched with 5hmC during differentiation (Pastor et al., 2011; Wu et al., 2011a), and 5hmC presence repels PRC2 binding (Wu et al., 2011b). Aligned with lower Prdm16 expression in Prkaa1−/− cells, the PRC2 mediated inhibitory histone modification, H2K27me3, was higher in the Prdm16 promoter (Figure S5B), and was accompanied by higher 5mC and lower 5hmC concentrations due to Prkaa1 KO (Figure 3G, 3H and 3J). Meanwhile, 5hmC enrichment was not detected in the Pparg2 promoter (Figure S5D), demonstrating that demethylation was locus-specific. The expression of all Tets, which catalyze 5hmC formation, was pronouncedly enhanced during brown adipogenic differentiation (Figure 3I), suggesting the importance of DNA demethylation during brown adipogenesis. On the other hand, the expression of DNA methyltransferases (DNMTs) was unaltered (Figure S5C).
Prkaa1 ablation impairs DNA demethylation in the Prdm16 promoter via an α-ketoglutarate-dependent mechanism
We hypothesized that the availability of substrate, αKG, has a critical role in mediating DNA demethylation of the Prdm16 promoter. We employed metabolomic analyses to identify metabolites in WT and Prkaa1−/− BSVs. Surprisingly, the concentration of αKG was extremely low in both WT BSVs and E13.5 MEFs (Figure 4A, 4B). Compared to progenitors, αKG concentration was vastly higher in BAT or differentiated brown adipocytes at day 3, while citrate content only slightly differed between progenitor cells and BAT (Figure 4A, 4B). Thus, αKG, due to its very low concentration (WT: 14.15 ± 2.2 µM at day 0; 33.49 ± 6.1 µM at day 3), likely is the rate limiting factor hindering TET-mediated DNA demethylation in progenitor cells considering αKG Km for TETs is around 50–60 µM (Laukka et al., 2016) (Figure 4A). We further compared metabolite contents between WT and Prkaa1−/− cells and found that αKG was reduced due to Prkaa1 KO (Figure 4C), which was confirmed by chemical analysis (Figure 4D). We then analyzed the expression of rate-limiting enzymes catalyzing αKG generation. During differentiation, the expression of isocitrate dehydrogenases (IDH) were dramatically increased, which might provide an explanation for the low αKG content in progenitor cells (Figure 4E). Furthermore, the expression of IDH2 was suppressed due to the absence of Prkaa1 (Figure 4E, 4F). We then analyzed the IDH2 acetylation that is known to reduce its activity (Lombard et al., 2007; Someya et al., 2010; Yu et al., 2012), and discovered that it was indeed increased with Prkaa1 KO (Figure 4F and Figure S6A). Thus, Prkaa1 deficiency inhibits IDH2 activity. We then deleted Idh2 gene in C3H10T1/2 cells, induced brown adipogenesis and discovered that Idh2 KO decreased αKG level (Figure S6B and S6C) and suppressed PRDM16 content (Figure S6D), which was accompanied by impaired brown adipogenesis (Figure S6E). In short, Prkaa1 regulates cellular αKG level at least partially through altering IDH2 activity.
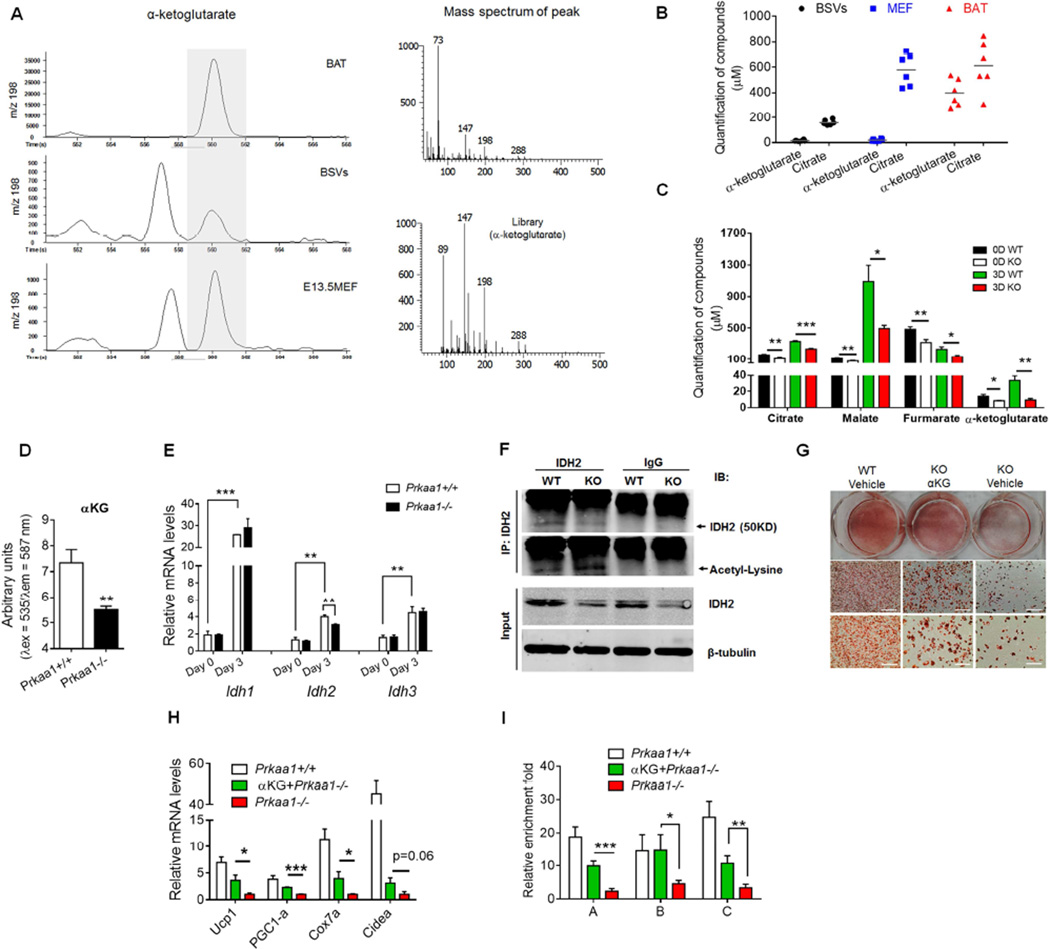
(A, B) Metabolomic analyses of metabolites in E13.5 mouse embryonic fibroblasts (MEF), brown stromal vascular cells (BSV) and neonatal brown adipose tissue (n = 6). Mass spectrum of αa-ketoglutarate (A) and quantification of α-ketoglutarate content (B). (C) Concentration of key metabolites of the TCA cycle of WT and Prkaa1 ablated cells (n = 6). (D) Higher α-ketoglutarate concentration in WT compared to Prkaa1 ablated BSVs (n = 5). (E) Isocitrate dehydrogenases (IDH) 1, 2 and 3 expression before and 3 days after brown adipogenic differentiation (n = 3). (F) BSVs were separated from brown fat of WT and Prkaa1 conditional KO mice (AMPKα1 KO was induced by tamoxifen) and, then, induced brown adipogenesis for 3 days. The IDH2 content was lower in Prkaa1 ablated cells, while IDH2 acetylation analyzed by immunoprecipitation was higher. (G–I) α-Ketoglutarate supplementation rescues brown adipogenesis in Prkaa1 ablated cells (n = 3). α-Ketoglutarate (4 mM) was added during the commitment stage (initial 3 days of brown adipogenic differentiation). Oil-red-O staining of BSVs at day 7 after inducing brown adipogenesis (G, Scale bar, 400µm and 200 µm); the mRNA expression of brown adipocyte markers at day 7 post-differentiation (H); 5hmC enrichment in the Prdm16 promoter at day 3 of differentiation (I). Gene mRNA expression was normalization to the 18S rRNA; Immunoprecipitated target sequences were analyzed by qPCR and normalized to inputs. (*P< 0.05; **P < 0.01; ***P < 0.001; mean ± s.e.m.; n = 5 unless otherwise indicated; Student’s t test with a two-tailed distribution.)
We then questioned whether αKG can recover brown adipogenesis. In fact, supplementing dimethyl-α-ketoglutarate, a membrane permeable αKG, in BSVs and C3H10T1/2 cells enhanced brown adipogenesis (Figure 4G, 4H and Figure 5A–D), which was correlated with enrichment of 5hmC in the Prdm16 promoter (Figure 4I), showing enhanced DNA demethylation. We further found that, at the same concentration, αKG enhanced TET activity in the nuclei, while 2-hydroxyglutarate, an αKG competitive inhibitor (Xu et al., 2011a), blocked TET activity without changing Tet expression, accompanied by altered brown adipogenesis (Figure 5E–5I). However, during white adipogenesis, αKG had no effect (Figure S6F–H), showing that αKG specifically promotes brown adipogenesis. Furthermore, Prkaa1 loss during white adipogenesis in inguinal SVF had no effect on white adipocyte commitment but enhanced expression of mature adipogenic markers (Figure S6I–K).

(A–D) αKG was added during the initial 3 days of brown adipogenesis in C3H10T1/2 cells. PRDM16 (at day 3) and UCP1 (at day 7) contents were enhanced due to aKG supplementation (A); Oil-Red-O staining of brown adipocytes (B, Scale bar, 200µm) and UCP-1 content (C, Scale bar, 100µm) after 7 days of brown adipogenic differentiation; aKG dose dependently enhanced brown adipogenesis based on qualification of Oil-Red-O staining by light absorbance at 530 nm (D). (E–I) At the same concentration (1mM), aKG enhanced while 2-hydroxyglutarate inhibited TET activity. Diethyl-α-ketoglutarate, diethyl-2-hydroxyglutarate and vehicle only were separately added to C3H10T1/2 cells during the initial three days of brown adipogenesis. aKG and 2-hydroxyglutarate did not affect Tet1,2,3 expression (E); nuclear fraction was isolated at day 3 of differentiation and extracts were used for analyzing TET activity (F); mRNA expression of brown adipocyte markers at day 7 of differentiation (G); qualification of Oil-Red-O staining by light absorbance at 530 nm (H); images of Oil-Red-O staining of brown adipocytes at day 7 of differentiation (I, Scale bar, 200µm). Gene mRNA expression was normalization to the 18S rRNA; Immunoprecipitated target sequences were analyzed by qPCR and normalized to inputs. (*P < 0.05; **P < 0.01; ***P < 0.001; mean ± s.e.m.; n = 5 unless otherwise indicated; Student’s t test with a two-tailed distribution. (*P<0.05; ***P < 0.001; P**** < 0.0001; n = 3; means ± s.e.m.; one-way ANOVA with Tukey’s post hoc test was used in D, F and H.)
Absence of Prkaa1 mimics the impact of obesity on BAT properties
We found that the body temperature of neonates from obese mothers (OB) was much lower than that of non-obese controls (Con) (Figure 6A). While there was no difference in the litter sizes, the death rate of OB neonates was much higher than that of Con and correlated with the low body temperature (Figure 6B, 6C), suggesting hypothermia might be a key mechanism leading to neonatal death. This observation is consistent with relatively high death rates of neonates of obese women (Chen et al., 2009; Hoppenbrouwers, 2014). Sudden Infant Death Syndrome (SIDS) is relatively common and devastating, with poorly defined etiology (Kinney and Thach, 2009). It suggests that the impaired BAT function may be one of the key etiological factors, consistent with earlier observation of BAT deficiency in SIDS infants during autopsy (Vijgen and van Marken Lichtenbelt, 2013). In agreement, offspring of obese mice had a deficient BAT similar to Prkaa1 KO mice (Figure 6D–6F, 6H–6K, S7A and S7B). Interestingly, the AMPK phosphorylation which correlates with AMPK activity, was much lower in OB compared to Con BAT at E15.5, a critical stage for brown adipogenesis (Figure 6G). Furthermore, Prdm16 expression was diminished in the BAT of obese neonates and weaning mice (Figure 6I, ,7F),7F), which was correlated with lower αKG content (Figure 6H), reminiscent of what observed in Prkaa1−/− mice. These data are supported by a recent clinical study showing that MO increased DNA methylation in the Prdm16 promoter in placenta at birth (Côté et al., 2013). Consistently, the UCP1 expression was reduced in E18.5 fetus of obese mothers (Figure S7C, S7D). Therefore, we also analyzed the impact of cold stimulus on the body temperature of weaning mice, and OB offspring demonstrated impaired ability to maintain body temperature in a cold environment (Figure 6L), consistent with lower Ucp1 expression (Figure 6M). Up to 3 months of HFD exposure, the BAT of OB mice demonstrated a phenotype that resembled white fat (Figure 6N). We demonstrated that under HFD exposure, the BAT identity of AMPK KO and OB mice was weakened, with impaired ability of thermogenesis. These data clearly show the impairment of BAT function in both Prkaa1−/− mice and OB offspring.

(A) Infrared (up) and photographic (down) images of newborn mice. Left panel shows offspring of maternal control mice (Con) and right panel shows offspring of maternal obese mice (OB) at the same scale. (B) Litter sizes of neonates of Con and OB mothers. (C) Tracing death rates during 0–96 h after birth. (D, E) OB impaired the development of BAT in offspring. OB reduced absolute weight of BAT of offspring mice at P0 (D) and P21 (E) (n = 10). (F) Hematoxylin and eosin (H&E) staining of sections from the interscapular regions of neonatal Con and OB mice (Scale bar, 200µm). (G) During the early fetal development, MO suppressed p-AMPK (T172) level at E15.5. (H) MO reduced α-ketoglutarate content in E15.5 fetal tissue (n = 5). (I) mRNA expression of Prdm16 (Con = 4, OB = 5) at P0. (J, K) Brown adipogenesis was impaired at P0. UCP1 contents (n = 3) (J) and immunofluorescence staining of UCP1 at P0 (Scale bar, 200µm) (K). (L–N) BAT functionality in offspring of obese mothers was compromised. MO impaired BAT thermogenesis of P21 mice under cold exposure (6 h, 5 °C). Rectal temperature (L), Ucp1 expression (M), and H&E staining (N, Scale bar, 200µm) of sections from the BAT of 3 month old offspring fed with HFD (n = 4). Gene mRNA expression was normalization to the 18S rRNA. (*P< 0.05; ***P < 0.001; means ± s.e.m.; Student’s t test with a two-tailed distribution.).
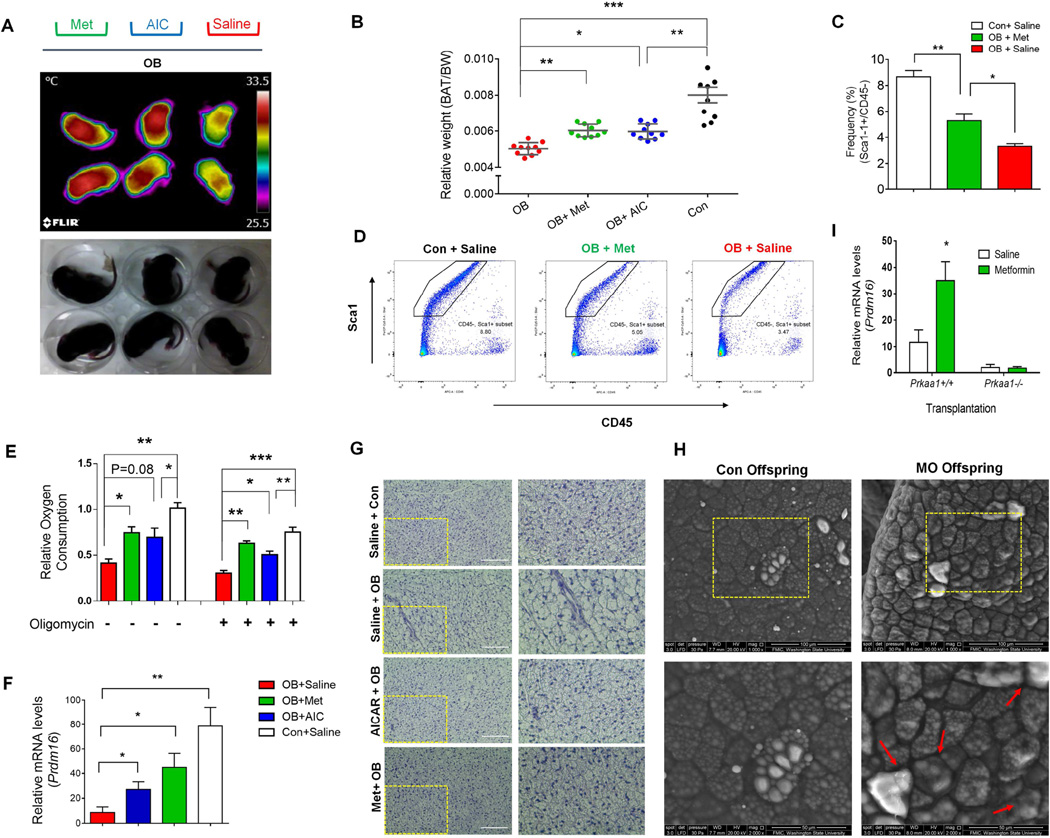
(A) Infrared (up) and photographic (down) images of OB offspring (10 days old) which had been administered with metformin, AICAR or vehicle daily withdrawn 24 h before measurement. (B–E) AMPKα agonists partially recover BAT function in the offspring of obese mice (metformin, AICAR and vehicle administration daily up to P15, and samples were collected at P21). The BAT weight (B) (n = 10), density of progenitor cells (C, D), oxygen consumption of both coupled and uncoupled (E), Prdm16 mRNA expression (F), and Hematoxylin and eosin (H&E) staining of sections from the BAT (G, Scale bar, 200 µm). (H) Scanning electron microscopic images of BAT at OB and Con offspring of 3 month old (Scale bar, 100 and 50µm). (I) BATs were separated from WT and AMPK floxed mice and, then, transplanted into the same recipient mice (Three days after transplantation, recipient mice were injected with tamoxifen daily for 4 days to induce Prkaa1 KO in transplanted Prkaa1flox/floxCre+/− BAT. Also see Figure S3 and Supplemental Experimental Procedures for the detailed protocol). Gene mRNA expression was normalization to the 18S rRNA. (*P < 0.05; **P < 0.01; ***P < 0.001; mean ± s.e.m.; n = 4 unless otherwise indicated; one-way ANOVA with Tukey’s post hoc test was used in B, C, E).
AMPK agonists administrated during the early stage ameliorate impaired BAT development in weaning obese mice
The similarity of BAT phenotype between Prkaa1−/− and obese neonates suggested that suppression of Prkaa1 activity might be a key mechanism impairing BAT development. Thus, activating AMPK in OB offspring should recover BAT development. Indeed, the neonatal temperature was reduced due to maternal obesity and largely recovered through the administration of both metformin and AICAR, two activators of AMPK, after birth (Figure 7A). At weaning (P21), BAT weights were partially recovered due to AMPK activation (Figure 7B), which was associated with increased density of Lin-/CD45-/Sca-1+ progenitor cells (Figure 7C, 7D). Such recovery is striking considering that the drug administration was initiated after birth and could only influence postnatal BAT development. In agreement, the oxygen consumption of isolated BAT and browning identity marker Prdm16 were increased due to metformin and AICAR treatments (Figure 7E, 7F and Figure S7E, S7F). Consequently, visceral fat weight was reduced in treated mice (Figure S7G), consistent with a recent clinical report showing that BAT development and visceral fat accumulation are mutually antagonistic (Wang et al., 2015). In addition, maternal obesity impaired glucose tolerance of offspring (Figure S7H, S7I) at weaning, which was ameliorated by metformin. Histologically, BAT of OB mice developed into WAT-like adipocytes compared to those of Con mice and OB with AMPK agonists (Figure 7G, 7H). Because metformin affects whole body metabolism in addition to BAT, we conducted a transplantation study. BATs were separated from AMPK floxed mice with/without Cre and then transplanted into the same recipient mice (Figure S3). After inducing Prkaa1 KO and treating with metformin, Prdm16 expression was elevated only in WT transplanted BAT, not in Prkaa1−/−BAT, showing that metformin promoted brown adipogenesis through AMPKα1 in vivo, independent of other physiological changes (Figure 7I). Overall, these results show that drugs activating AMPK rescued BAT development and its functionality in obese mice.
DISCUSSION
Our data delineate a key mechanism linking AMPK and BAT development via changing an important metabolite, αKG, and subsequent TET-mediated DNA demethylation in the Prdm16 promoter. AMPK is an excellent drug target to promote BAT development under obese condition where AMPK activity is inhibited (Steinberg et al., 2006). The importance of this mechanism is further highlighted by the extremely low concentrations of αKG and low Tet expression in progenitor cells and their surge during early brown adipogenesis, suggesting the central role of DNA demethylation in initiating brown adipocyte differentiation. Our findings further reveals an intrinsic link between metabolism and brown adipogenesis that is mediated by AMPK. Because drugs activating AMPK are commonly used, such as metformin, this mechanism provides a promising therapeutic strategy to promote BAT development and reduce metabolic diseases.
Strikingly, we observed that there is a profound increase in αKG concentration during the brown adipogenesis of progenitor cells, which suggests a possible generic mechanism regulating stem cell and progenitor differentiation, via vast up-regulation of IDH expression and αKG concentration, serves to facilitate DNA demethylation of key developmental genes. This notion is supported by the inhibitory roles of αKG and IDH in carcinogenesis (Possemato et al., 2011; Prensner and Chinnaiyan, 2011), where cancer stem cells fail to undergo differentiation; on the other hand, antagonization of αKG by its reduced form, an oncometabolite 2-hydroxylglutarate, promotes oncogenesis (Ye et al., 2012). Besides, αKG extends lifespan in C. elegans (Chin et al., 2014). Intriguingly, AMPK activation also inhibits tumor growth (Faubert et al., 2013; Shackelford and Shaw, 2009) and extends lifespan in C. elegans (Shackelford and Shaw, 2009). These data hint a fascinating possibility of priming the expression of lineage specific genes via boosting AMPK/αKG axis in order to treat cancers and other conditions.
Similar to the obesity epidemic in general population, more than 30% of pregnant women are obese and another one third overweight in the US (Flegal et al., 2012), which predisposes children to obesity and metabolic diseases (Lawlor et al., 2012). Our discovery of impairment in BAT development via AMPK/αKG pathway due to obesity provides a drug target for clinical interventions of obese pregnant women and their children thus curbing the physiological vicious cycle between maternal obesity and the obesity and T2D in children (Rando and Simmons, 2015). In fact, two multi-centric trials using metformin on obese pregnant women and their children are ongoing (MOP: NCT01273584, and EMPOWaR: ISRCTN51279843); it will be of great interest to see whether BAT development is improved with metformin treatment in these children. Besides, our finding has potential clinical link to SIDS, a devastating disease (Kinney and Thach, 2009), based on recent observation and the death rates of neonates higher in obese women (Chen et al., 2009; Leddy et al., 2008). Similarly, our discovery provides a potential drug target to enhance BAT thermogenic function and thus curbs obesity in general population.
The clinical application of our discovery is further heightened by those available dietary αKG supplements, such as ornithine α-ketoglutarate, which are known for their anabolic effect (Donati et al., 1999) and for potentially preventing sarcopenia (Walrand, 2010) and improving wound healing in burn patients (Donati et al., 1999). Clinically, αKG administration in vivo protects from ischemia-reperfusion injury (Olenchock et al., 2016). Despite unestablished mechanisms for the anabolic effects of αKG, these studies suggest the likely wide existence of AMPK/αKG mediated DNA demethylation in the processes of tissue development and regeneration that are only limited to BAT.
EXPERIMENTAL PROCEDURES
Animals
Animal studies were conducted according to the protocol approved by the Institute of Animal Use and Care Committees (IAUCC) at Washington State University. Additional information about the animal studies is described in Extended Experimental Procedures.
Brown fat transplantation
Brown fat transplantation was conducted as previously described with slight modifications (Stanford et al., 2013). Experimental procedures are provided in Extended Experimental Procedure.
Cell line, plasmid transfection and assays
The C3H10T1/2 cell line was purchased from ATCC (Manassas, VA) and cultured in DMEM containing 15% FBS at 37°C with 5% CO2. All details of cell operations were described in Extended Experimental Procedure.
2-Deoxy-D-glucose uptake assay
Glucose uptake by differentiated brown adipocytes was analyzed using a 2-Deoxy-D-Glucose Uptake Kit (Cayman chemical, Ann Arbor, MI).
Oil-Red-O staining of lipid droplets in adipocytes
Oil-Red-O staining as previously described (Jimenez et al., 2007).
Oxygen consumption assays
Isolated BAT was weighed and 20 mg of tissue slice was placed in a respiration buffer (2% BSA, 1.1 mM Sodium pyruvate and 25 mM glucose in PBS). Similarly, cultured cells were replaced with the respiration buffer. Oxygen consumption was measured using an Orion Dissolved Oxygen platform (Thermo Scientific, Waltham, MA) for approximately 25 min. Each sample was measured in triplicate (Harms et al., 2014b).
α-Ketoglutarate Assay
The αKG contents in BAT and differentiated brown adipocytes were analyzed using an α-Ketoglutarate Assay Kit (Sigma, St. Louis, MO).
Thermal imagining
Thermo-images were taken using an E6 Thermal Imaging Infrared Camera (FLIR Systems, Boston, MA).
Metabolomic analyses of BAT and BAT derived stem cells
Detailed protocols and analysis of data are described in the Extended Experimental Procedures.
IDH2 acetylation assay
Cell lysates were centrifuged at 13,000 g for 10 min at 4°C and the supernatant was pre-cleared with protein A/G magnetic beads (Thermo Fisher Scientific). Subsequently, the lysates were incubated overnight at 4°C with anti-IDH2 antibody (12652, Cell Signaling Technology) and then incubated with protein A/G magnetic beads. Equivalent amounts of protein were analyzed for each sample. The normal rabbit IgG (2729, Cell Signaling Technology) was used as the negative control. The beads-protein complexes were boiled in SDS sample buffer and subjected to western blotting.
H&E staining and Immunofluorescence staining
Adipose tissues were fixed in 4% PFA for 24 h at 4°C. After dehydration, the tissues were embedded into paraffin and cut into 5 µm thick slices, deparaffinized and rehydrated using standard methods. Sections were stained with hematoxylin & eosin. For immunohistochemical staining, antigen retrieval was performed by submerging in 0.01 M sodium citrate (pH 6.0) and heated to boil for 20 min in a microwave oven. BAT was subjected to immunofluorescence staining and antibodies against UCP1 was purchased from Santa Cruz Biotech (sc-28766), and Ki67 (12202) was purchased from Cell signaling technology.
Mitochondrial measurement
A Mitochondria Tracker Kit purchased was used to visualize mitochondria (Cell Signaling Technology).
Transmission electron microscope (TEM) and Scanned electron microscope (SEM)
The protocols were based on previous reports (Bartelt et al., 2011; Harms et al., 2014a).
Glucose tolerance test
The assay was conducted as previously described (Stanford et al., 2013).
Statistical analyses
Previous experiments done were used to determine sample size with adequate statistical power. Results were analyzed using Student’s t-test or one-way ANOVA (for multiple comparison) where appropriate, using GraphPad Prism software. All analyses were conducted with Student’s t test with a two-tailed distribution, except recovery experiments (Figure 4H–I & Figure 7) where a one-tailed distribution was used. All data were found to be normally distributed. Significance was accepted at P < 0.05. The researchers involved in experiments were not completely blinded during sample obtainment or data analysis. All data are reported as mean ± s.e.m.
Acknowledgments
We thank Valerie J. Lynch-Holm for providing technical support of the TEM and SEM imaging system (Franceschi Microscopy and Imaging Center, Washington State University); Liang Wang (Department of Chemistry, Washington State University) for technical support with the GS-MS. This work was supported by a grant from the US National Institutes of Health (R01-HD067449).
Footnotes
Publisher's Disclaimer: This is a PDF file of an unedited manuscript that has been accepted for publication. As a service to our customers we are providing this early version of the manuscript. The manuscript will undergo copyediting, typesetting, and review of the resulting proof before it is published in its final citable form. Please note that during the production process errors may be discovered which could affect the content, and all legal disclaimers that apply to the journal pertain.
AUTHOR CONTRIBUTIONS
Q.-Y.Y. and M.D. conceived the project, designed the experiments, and wrote the manuscript. Q. -Y.Y. and X. -W.L. researched data. Q.-Y.Y., X.-W.L., X.-F.S. and L.-P.Z. performed the experiments. X.F., C.J.R., A.B., S.-M.Z., S.-B.W. and B.W. provided technical expertise. M.F. and B.V. contributed the transgenic mouse. D.R.G., B.D.R. and M.-J.Z. contributed to discussion and reviewed and edited the manuscript.No conflicts of interest, financial or otherwise, are declared by the authors.
REFERENCES
- Bartelt A, Bruns OT, Reimer R, Hohenberg H, Ittrich H, Peldschus K, Kaul MG, Tromsdorf UI, Weller H, Waurisch C, et al. Brown adipose tissue activity controls triglyceride clearance. Nature medicine. 2011;17:200–205. [Abstract] [Google Scholar]
- Bauwens JD, Schmuck EG, Lindholm CR, Ertel RL, Mulligan JD, Hovis I, Viollet B, Saupe KW. Cold tolerance, cold-induced hyperphagia, and nonshivering thermogenesis are normal in alpha(1)-AMPK−/− mice. American journal of physiology. Regulatory, integrative and comparative physiology. 2011;301:R473–R483. [Europe PMC free article] [Abstract] [Google Scholar]
- Chen A, Feresu SA, Fernandez C, Rogan WJ. Maternal obesity and the risk of infant death in the United States. Epidemiology. 2009;20:74–81. [Europe PMC free article] [Abstract] [Google Scholar]
- Chin RM, Fu X, Pai MY, Vergnes L, Hwang H, Deng G, Diep S, Lomenick B, Meli VS, Monsalve GC, et al. The metabolite alpha-ketoglutarate extends lifespan by inhibiting ATP synthase and TOR. Nature. 2014;510:397–401. [Europe PMC free article] [Abstract] [Google Scholar]
- Cohen P, Levy Julia D, Zhang Y, Frontini A, Kolodin Dmitriy P, Svensson Katrin J, Lo James C, Zeng X, Ye L, Khandekar Melin J, et al. Ablation of PRDM16 and Beige Adipose Causes Metabolic Dysfunction and a Subcutaneous to Visceral Fat Switch. Cell. 2014;156:304–316. [Europe PMC free article] [Abstract] [Google Scholar]
- Côté S, Brisson D, Guérin R, Perron P, St-Pierre J, Gaudet D, Hivert MF, Bouchard L. PRDM16 Gene DNA Methylation Levels in the Placenta Are Associated With Maternal Overweight and Obesity at First Trimester of Pregnancy. Canadian Journal of Diabetes. 2013;37:59. [Google Scholar]
- Daval M, Diot-Dupuy F, Bazin R, Hainault I, Viollet B, Vaulont S, Hajduch E, Ferre P, Foufelle F. Anti-lipolytic action of AMP-activated protein kinase in rodent adipocytes. The Journal of biological chemistry. 2005;280:25250–25257. [Abstract] [Google Scholar]
- Donati L, Ziegler F, Pongelli G, Signorini M. Nutritional and clinical efficacy of ornithine alphaketog-ketoglutaratein severe burn patients. Clinical Nutrition. 1999;18:307–311. [Abstract] [Google Scholar]
- Drahota Z, Alexander A, Rossi C, Siliprandi N. Organization and regulation of fatty acid oxidation in mitochondria of brown adipose tissue. Biochimica et Biophysica Acta (BBA)-Bioenergetics. 1970;205:491–498. [Abstract] [Google Scholar]
- Faubert B, Boily G, Izreig S, Griss T, Samborska B, Dong Z, Dupuy F, Chambers C, Fuerth BJ, Viollet B, et al. AMPK is a negative regulator of the Warburg effect and suppresses tumor growth in vivo. Cell metabolism. 2013;17:113–124. [Europe PMC free article] [Abstract] [Google Scholar]
- Ficz G, Branco MR, Seisenberger S, Santos F, Krueger F, Hore TA, Marques CJ, Andrews S, Reik W. Dynamic regulation of 5-hydroxymethylcytosine in mouse ES cells and during differentiation. Nature. 2011;473:398–402. [Abstract] [Google Scholar]
- Flegal KM, Carroll MD, Kit BK, Ogden CL. Prevalence of obesity and trends in the distribution of body mass index among US adults, 1999–2010. JAMA : the journal of the American Medical Association. 2012;307:491–497. [Abstract] [Google Scholar]
- Hardie DG. Sensing of energy and nutrients by AMP-activated protein kinase. The American journal of clinical nutrition. 2011;93:891S–896. [Abstract] [Google Scholar]
- Harms Matthew J, Ishibashi J, Wang W, Lim H-W, Goyama S, Sato T, Kurokawa M, Won K-J, Seale P. Prdm16 Is Required for the Maintenance of Brown Adipocyte Identity and Function in Adult Mice. Cell metabolism. 2014a;19:593–604. [Europe PMC free article] [Abstract] [Google Scholar]
- Harms MJ, Ishibashi J, Wang W, Lim HW, Goyama S, Sato T, Kurokawa M, Won KJ, Seale P. Prdm16 is required for the maintenance of brown adipocyte identity and function in adult mice. Cell metabolism. 2014b;19:593–604. [Europe PMC free article] [Abstract] [Google Scholar]
- Hoppenbrouwers T. Sudden Infant Death Syndrome, Sleep, and Seizures. Journal of child neurology. 2014 [Abstract] [Google Scholar]
- Ito S, D’Alessio AC, Taranova OV, Hong K, Sowers LC, Zhang Y. Role of Tet proteins in 5mC to 5hmC conversion, ES-cell self-renewal and inner cell mass specification. Nature. 2010;466:1129–1133. [Europe PMC free article] [Abstract] [Google Scholar]
- Jimenez MA, Akerblad P, Sigvardsson M, Rosen ED. Critical role for Ebf1 and Ebf2 in the adipogenic transcriptional cascade. Molecular and cellular biology. 2007;27:743–757. [Europe PMC free article] [Abstract] [Google Scholar]
- Jones PA. Functions of DNA methylation: islands, start sites, gene bodies and beyond. Nature reviews. Genetics. 2012;13:484–492. [Abstract] [Google Scholar]
- Kinney HC, Thach BT. The sudden infant death syndrome. New England Journal of Medicine. 2009;361:795–805. [Europe PMC free article] [Abstract] [Google Scholar]
- Kron K, Trudel D, Pethe V, Briollais L, Fleshner N, van der Kwast T, Bapat B. Altered DNA methylation landscapes of polycomb-repressed loci are associated with prostate cancer progression and ERG oncogene expression in prostate cancer. Clinical cancer research : an official journal of the American Association for Cancer Research. 2013;19:3450–3461. [Abstract] [Google Scholar]
- Ku M, Koche RP, Rheinbay E, Mendenhall EM, Endoh M, Mikkelsen TS, Presser A, Nusbaum C, Xie X, Chi AS, et al. Genomewide analysis of PRC1 and PRC2 occupancy identifies two classes of bivalent domains. PLoS genetics. 2008;4:e1000242. [Europe PMC free article] [Abstract] [Google Scholar]
- Laukka T, Mariani CJ, Ihantola T, Cao JZ, Hokkanen J, Kaelin WG, Jr, Godley LA, Koivunen P. Fumarate and Succinate Regulate Expression of Hypoxia-inducible Genes via TET Enzymes. The Journal of biological chemistry. 2016;291:4256–4265. [Europe PMC free article] [Abstract] [Google Scholar]
- Lawlor DA, Relton C, Sattar N, Nelson SM. Maternal adiposity--a determinant of perinatal and offspring outcomes? Nature reviews. Endocrinology. 2012;8:679–688. [Abstract] [Google Scholar]
- Leddy MA, Power ML, Schulkin J. The impact of maternal obesity on maternal and fetal health. Reviews in obstetrics and gynecology. 2008;1:170. [Abstract] [Google Scholar]
- Lombard DB, Alt FW, Cheng HL, Bunkenborg J, Streeper RS, Mostoslavsky R, Kim J, Yancopoulos G, Valenzuela D, Murphy A, et al. Mammalian Sir2 homolog SIRT3 regulates global mitochondrial lysine acetylation. Molecular and cellular biology. 2007;27:8807–8814. [Europe PMC free article] [Abstract] [Google Scholar]
- Martinez de Morentin PB, Gonzalez-Garcia I, Martins L, Lage R, Fernandez-Mallo D, Martinez-Sanchez N, Ruiz-Pino F, Liu J, Morgan DA, Pinilla L, et al. Estradiol Regulates Brown Adipose Tissue Thermogenesis via Hypothalamic AMPK. Cell metabolism. 2014 [Europe PMC free article] [Abstract] [Google Scholar]
- Meissner A, Mikkelsen TS, Gu H, Wernig M, Hanna J, Sivachenko A, Zhang X, Bernstein BE, Nusbaum C, Jaffe DB. Genome-scale DNA methylation maps of pluripotent and differentiated cells. Nature. 2008;454:766–770. [Europe PMC free article] [Abstract] [Google Scholar]
- Mikkelsen TS, Ku M, Jaffe DB, Issac B, Lieberman E, Giannoukos G, Alvarez P, Brockman W, Kim T-K, Koche RP. Genome-wide maps of chromatin state in pluripotent and lineage-committed cells. Nature. 2007a;448:553–560. [Europe PMC free article] [Abstract] [Google Scholar]
- Mikkelsen TS, Ku M, Jaffe DB, Issac B, Lieberman E, Giannoukos G, Alvarez P, Brockman W, Kim TK, Koche RP, et al. Genome-wide maps of chromatin state in pluripotent and lineage-committed cells. Nature. 2007b;448:553–560. [Europe PMC free article] [Abstract] [Google Scholar]
- Mohn F, Weber M, Rebhan M, Roloff TC, Richter J, Stadler MB, Bibel M, Schubeler D. Lineage-specific polycomb targets and de novo DNA methylation define restriction and potential of neuronal progenitors. Molecular cell. 2008;30:755–766. [Abstract] [Google Scholar]
- Olenchock BA, Moslehi J, Baik AH, Davidson SM, Williams J, Gibson WJ, Pierce KA, Miller CM, Hanse EA, Kelekar A, et al. EGLN1 Inhibition and Rerouting of alpha-Ketoglutarate Suffice for Remote Ischemic Protection. Cell. 2016;164:884–895. [Europe PMC free article] [Abstract] [Google Scholar]
- Pastor WA, Pape UJ, Huang Y, Henderson HR, Lister R, Ko M, McLoughlin EM, Brudno Y, Mahapatra S, Kapranov P, et al. Genome-wide mapping of 5-hydroxymethylcytosine in embryonic stem cells. Nature. 2011;473:394–397. [Europe PMC free article] [Abstract] [Google Scholar]
- Pedersen JI, Grav HJ. Physiologically-Induced Loose Coupling of Brown-Adipose-Tissue Mitochondria Correlated to Endogenous Fatty Acids and Adenosine Phosphates. European Journal of Biochemistry. 1972;25:75–83. [Abstract] [Google Scholar]
- Peirce V, Carobbio S, Vidal-Puig A. The different shades of fat. Nature. 2014;510:76–83. [Abstract] [Google Scholar]
- Possemato R, Marks KM, Shaul YD, Pacold ME, Kim D, Birsoy K, Sethumadhavan S, Woo HK, Jang HG, Jha AK, et al. Functional genomics reveal that the serine synthesis pathway is essential in breast cancer. Nature. 2011;476:346–350. [Europe PMC free article] [Abstract] [Google Scholar]
- Prensner JR, Chinnaiyan AM. Metabolism unhinged: IDH mutations in cancer. Nature medicine. 2011;17:291–293. [Abstract] [Google Scholar]
- Rando OJ, Simmons RA. I’m eating for two: parental dietary effects on offspring metabolism. Cell. 2015;161:93–105. [Europe PMC free article] [Abstract] [Google Scholar]
- Schuettengruber B, Cavalli G. Recruitment of polycomb group complexes and their role in the dynamic regulation of cell fate choice. Development. 2009;136:3531–3542. [Abstract] [Google Scholar]
- Schulz TJ, Huang P, Huang TL, Xue R, McDougall LE, Townsend KL, Cypess AM, Mishina Y, Gussoni E, Tseng YH. Brown-fat paucity due to impaired BMP signalling induces compensatory browning of white fat. Nature. 2013;495:379–383. [Europe PMC free article] [Abstract] [Google Scholar]
- Seale P, Bjork B, Yang W, Kajimura S, Chin S, Kuang S, Scime A, Devarakonda S, Conroe HM, Erdjument-Bromage H, et al. PRDM16 controls a brown fat/skeletal muscle switch. Nature. 2008;454:961–967. [Europe PMC free article] [Abstract] [Google Scholar]
- Shackelford DB, Shaw RJ. The LKB1-AMPK pathway: metabolism and growth control in tumour suppression. Nature reviews. Cancer. 2009;9:563–575. [Europe PMC free article] [Abstract] [Google Scholar]
- Someya S, Yu W, Hallows WC, Xu J, Vann JM, Leeuwenburgh C, Tanokura M, Denu JM, Prolla TA. Sirt3 mediates reduction of oxidative damage and prevention of age-related hearing loss under caloric restriction. Cell. 2010;143:802–812. [Europe PMC free article] [Abstract] [Google Scholar]
- Stanford KI, Middelbeek RJ, Townsend KL, An D, Nygaard EB, Hitchcox KM, Markan KR, Nakano K, Hirshman MF, Tseng YH, et al. Brown adipose tissue regulates glucose homeostasis and insulin sensitivity. The Journal of clinical investigation. 2013;123:215–223. [Europe PMC free article] [Abstract] [Google Scholar]
- Steinberg GR, Michell BJ, van Denderen BJ, Watt MJ, Carey AL, Fam BC, Andrikopoulos S, Proietto J, Gorgun CZ, Carling D, et al. Tumor necrosis factor alpha-induced skeletal muscle insulin resistance involves suppression of AMP-kinase signaling. Cell metabolism. 2006;4:465–474. [Abstract] [Google Scholar]
- Tan SX, Hu RC, Liu JJ, Tan YL, Liu WE. Methylation of PRDM2, PRDM5 and PRDM16 genes in lung cancer cells. International journal of clinical and experimental pathology. 2014;7:2305–2311. [Europe PMC free article] [Abstract] [Google Scholar]
- Tran KV, Gealekman O, Frontini A, Zingaretti MC, Morroni M, Giordano A, Smorlesi A, Perugini J, De Matteis R, Sbarbati A, et al. The vascular endothelium of the adipose tissue gives rise to both white and brown fat cells. Cell metabolism. 2012;15:222–229. [Europe PMC free article] [Abstract] [Google Scholar]
- Vijgen G, van Marken Lichtenbelt W. Brown adipose tissue: clinical impact of a re-discovered thermogenic organ. Front Biosci (Elite Ed) 2013;5:823–833. [Abstract] [Google Scholar]
- Virtanen KA, Lidell ME, Orava J, Heglind M, Westergren R, Niemi T, Taittonen M, Laine J, Savisto N-J, Enerbäck S. Functional brown adipose tissue in healthy adults. New England Journal of Medicine. 2009;360:1518–1525. [Abstract] [Google Scholar]
- Walrand S. Ornithine alpha-ketoglutarate: could it be a new therapeutic option for sarcopenia? The journal of nutrition, health & aging. 2010;14:570–577. [Abstract] [Google Scholar]
- Wang Q, Zhang M, Xu M, Gu W, Xi Y, Qi L, Li B, Wang W. Brown adipose tissue activation is inversely related to central obesity and metabolic parameters in adult human. PloS one. 2015;10:e0123795. [Europe PMC free article] [Abstract] [Google Scholar]
- Whittle AJ, Carobbio S, Martins L, Slawik M, Hondares E, Vazquez MJ, Morgan D, Csikasz RI, Gallego R, Rodriguez-Cuenca S, et al. BMP8B increases brown adipose tissue thermogenesis through both central and peripheral actions. Cell. 2012;149:871–885. [Europe PMC free article] [Abstract] [Google Scholar]
- Wu H, D’Alessio AC, Ito S, Wang Z, Cui K, Zhao K, Sun YE, Zhang Y. Genome-wide analysis of 5-hydroxymethylcytosine distribution reveals its dual function in transcriptional regulation in mouse embryonic stem cells. Genes & development. 2011a;25:679–684. [Europe PMC free article] [Abstract] [Google Scholar]
- Wu H, D’Alessio AC, Ito S, Xia K, Wang Z, Cui K, Zhao K, Sun YE, Zhang Y. Dual functions of Tet1 in transcriptional regulation in mouse embryonic stem cells. Nature. 2011b;473:389–393. [Europe PMC free article] [Abstract] [Google Scholar]
- Wu H, Zhang Y. Reversing DNA methylation: mechanisms, genomics, and biological functions. Cell. 2014;156:45–68. [Europe PMC free article] [Abstract] [Google Scholar]
- Xu W, Yang H, Liu Y, Yang Y, Wang P, Kim SH, Ito S, Yang C, Wang P, Xiao MT, et al. Oncometabolite 2-hydroxyglutarate is a competitive inhibitor of alpha-ketoglutarate-dependent dioxygenases. Cancer cell. 2011a;19:17–30. [Europe PMC free article] [Abstract] [Google Scholar]
- Xu Y, Wu F, Tan L, Kong L, Xiong L, Deng J, Barbera AJ, Zheng L, Zhang H, Huang S, et al. Genome-wide regulation of 5hmC, 5mC, and gene expression by Tet1 hydroxylase in mouse embryonic stem cells. Molecular cell. 2011b;42:451–464. [Europe PMC free article] [Abstract] [Google Scholar]
- Ye D, Xiong Y, Guan KL. The mechanisms of IDH mutations in tumorigenesis. Cell research. 2012;22:1102–1104. [Europe PMC free article] [Abstract] [Google Scholar]
- Yu W, Dittenhafer-Reed KE, Denu JM. SIRT3 protein deacetylates isocitrate dehydrogenase 2 (IDH2) and regulates mitochondrial redox status. The Journal of biological chemistry. 2012;287:14078–14086. [Europe PMC free article] [Abstract] [Google Scholar]
Full text links
Read article at publisher's site: https://doi.org/10.1016/j.cmet.2016.08.010
Read article for free, from open access legal sources, via Unpaywall:
http://www.cell.com/article/S1550413116304260/pdf
Citations & impact
Impact metrics
Citations of article over time
Alternative metrics
Article citations
Hederagenin from <i>Hedera helix</i> Promotes Fat Browning in 3T3-L1 Adipocytes.
Plants (Basel), 13(19):2789, 04 Oct 2024
Cited by: 0 articles | PMID: 39409659 | PMCID: PMC11478623
Directly targeting PRDM16 in thermogenic adipose tissue to treat obesity and its related metabolic diseases.
Front Endocrinol (Lausanne), 15:1458848, 16 Sep 2024
Cited by: 0 articles | PMID: 39351529 | PMCID: PMC11439700
Review Free full text in Europe PMC
Predicting gestational diabetes mellitus risk at 11-13 weeks' gestation: the role of extrachromosomal circular DNA.
Cardiovasc Diabetol, 23(1):289, 07 Aug 2024
Cited by: 0 articles | PMID: 39113025 | PMCID: PMC11304788
Strongylocentrotus intermedius Extract Suppresses Adiposity by Inhibiting Adipogenesis and Promoting Adipocyte Browning via AMPK Activation in 3T3-L1 Cells.
J Microbiol Biotechnol, 34(8):1688-1697, 12 Jul 2024
Cited by: 0 articles | PMID: 39086228 | PMCID: PMC11380521
AMPK Deficiency Increases DNA Methylation and Aggravates Colorectal Tumorigenesis in AOM/DSS Mice.
Genes (Basel), 15(7):835, 25 Jun 2024
Cited by: 0 articles | PMID: 39062614 | PMCID: PMC11276171
Go to all (142) article citations
Data
Data behind the article
This data has been text mined from the article, or deposited into data resources.
BioStudies: supplemental material and supporting data
Clinical Trials
- (1 citation) ClinicalTrials.gov - NCT01273584
Similar Articles
To arrive at the top five similar articles we use a word-weighted algorithm to compare words from the Title and Abstract of each citation.
Berberine promotes the recruitment and activation of brown adipose tissue in mice and humans.
Cell Death Dis, 10(6):468, 13 Jun 2019
Cited by: 44 articles | PMID: 31197160 | PMCID: PMC6565685
AMPKα1 deficiency suppresses brown adipogenesis in favor of fibrogenesis during brown adipose tissue development.
Biochem Biophys Res Commun, 491(2):508-514, 28 Jun 2017
Cited by: 12 articles | PMID: 28668388 | PMCID: PMC5737707
AMPKα1 regulates Idh2 transcription through H2B O-GlcNAcylation during brown adipogenesis.
Acta Biochim Biophys Sin (Shanghai), 53(1):112-118, 01 Jan 2021
Cited by: 7 articles | PMID: 33219380
Emerging Role of AMPK in Brown and Beige Adipose Tissue (BAT): Implications for Obesity, Insulin Resistance, and Type 2 Diabetes.
Curr Diab Rep, 18(10):80, 17 Aug 2018
Cited by: 86 articles | PMID: 30120579
Review
Funding
Funders who supported this work.
NIA NIH HHS (1)
Grant ID: R21 AG049976
NICHD NIH HHS (1)
Grant ID: R01 HD067449