Abstract
Objective
Inadequate right ventricular (RV) and pulmonary arterial (PA) functional responses to exercise are important yet poorly understood features of pulmonary arterial hypertension (PAH). This study combined invasive catheterisation with echocardiography to assess RV afterload, RV function and ventricular-vascular coupling in subjects with PAH.Methods
Twenty-six subjects with PAH were prospectively recruited to undergo right heart catheterisation and Doppler echocardiography at rest and during incremental exercise, and cardiac MRI at rest. Measurements at rest included basic haemodynamics, RV function and coupling efficiency (η). Measurements during incremental exercise included pulmonary vascular resistance (Z0), characteristic impedance (ZC, a measure of proximal PA stiffness) and proximal and distal PA compliance (CPA).Results
In patients with PAH, the proximal PAs were significantly stiffer at maximum exercise (ZC =2.31±0.38 vs 1.33±0.15 WU×m2 at rest; p=0.003) and PA compliance was decreased (CPA=0.88±0.10 vs 1.32±0.17 mL/mm Hg/m2 at rest; p=0.0002). Z0 did not change with exercise. As a result, the resistance-compliance (RC) time decreased with exercise (0.67±0.05 vs 1.00±0.07 s at rest; p<10-6). When patients were grouped according to resting coupling efficiency, those with poorer η exhibited stiffer proximal PAs at rest, a lower maximum exercise level, and more limited CPA reduction at maximum exercise.Conclusions
In PAH, exercise causes proximal and distal PA stiffening, which combined with preserved Z0 results in decreased RC time with exercise. Stiff PAs at rest may also contribute to poor haemodynamic coupling, reflecting reduced pulmonary vascular reserve that contributes to limit the maximum exercise level tolerated.Free full text

Reduced hemodynamic coupling and exercise are associated with vascular stiffening in pulmonary arterial hypertension
Abstract
Objective
Inadequate right ventricular (RV) and pulmonary arterial (PA) functional responses to exercise are important yet poorly understood features of pulmonary arterial hypertension (PAH). This study combined invasive catheterization with echocardiography to assess RV afterload, RV function and ventricular-vascular coupling in subjects with PAH.
Methods
Twenty-six subjects with PAH were prospectively recruited to undergo right heart catheterization and Doppler echocardiography at rest and during incremental exercise, and cardiac magnetic resonance imaging (MRI) at rest. Measurements at rest included basic hemodynamics, RV function and coupling efficiency (η). Measurements during incremental exercise included pulmonary vascular resistance (Z0), characteristic impedance (ZC, a measure of proximal PA stiffness) and proximal and distal PA compliance (CPA).
Results
In PAH patients, the proximal PAs were significantly stiffer at maximum exercise (ZC = 2.31 ± 0.38 vs. 1.33 ± 0.15 mmHg min/ml/m2 at rest; p=0.003) and PA compliance was decreased (CPA = 0.88 ± 0.10 vs. 1.32 ± 0.17 mL/mmHg/m2 at rest; p=0.0002). Z0 did not change with exercise. As a result, the resistance-compliance (RC) time decreased with exercise (1.00 ± 0.07 at vs. 0.67 ± 0.05 s; p<10−6). When patients were grouped according to resting coupling efficiency, those with poorer η exhibited stiffer proximal PAs at rest, a lower maximum exercise level, and more limited CPA reduction at maximum exercise.
Conclusions
In PAH, exercise causes proximal and distal PA stiffening, which combined with preserved Z0 results in decreased RC time with exercise. Stiff PAs at rest may also contribute to poor hemodynamic coupling, reflecting reduced pulmonary vascular reserve that contributes to limit the maximum exercise level tolerated.
Introduction
Assessment of right ventricular (RV) and pulmonary arterial (PA) function is critical for monitoring disease progression and identifying successful treatment strategies in patients with pulmonary arterial hypertension (PAH). In particular, metrics of RV-PA hemodynamic coupling and PA compliance have demonstrated better prognostic value than traditional hemodynamic measurements used in the clinical setting, such as mean pulmonary arterial pressure (mPAP) and pulmonary vascular resistance.[1–4] Furthermore, the response of the RV and pulmonary vasculature to exercise may be even more revealing.[5, 6] However, changes in hemodynamic coupling efficiency and PA compliance with exercise have been under-investigated, particularly in subjects with PAH.
In healthy subjects performing physical exercise, vascular resistance typically stays constant or decreases due to pulmonary arterial distension and recruitment of normally collapsed arteries.[7] The effect of exercise on resistance in patients with PAH have been inconsistent.[8–10] Furthermore, it is unclear how PA compliance changes during exercise.[11, 12] The resistance-compliance characteristic time (RC time), which is constant under most conditions, decreases during exercise in healthy subjects, while the results for PAH patients have not been definitive.[12] A more comprehensive measure of cardiopulmonary status is ventricular-vascular coupling efficiency.[13] Coupling efficiency at rest is decreased in PAH patients,[14, 15] and recent findings indicate that uncoupling occurs during exercise.[16] However, the associations of coupling efficiency at rest with vascular stiffness and maximum exercise level have not been investigated.
Here, a novel methodology was used to investigate the effect of exercise on RV afterload, including proximal PA stiffness (by characteristic impedance ZC), proximal and distal PA compliance (by CPA), and total pulmonary vascular resistance (by Z0), in subjects with PAH. Simultaneous PA pressure and flow measured with echocardiography during right heart catheterization (RHC) and exercise were analyzed. The hypothesis tested was that hemodynamic coupling efficiency at rest associates with proximal PA stiffness and maximum exercise level tolerated.
Methods
Study population and design
Subjects with known or suspected PAH who were referred for clinical RHC at Northwestern Memorial Hospital or University of Wisconsin-Hospital were invited to participate. Inclusion criteria were subjects aged 18–80 with diagnosed or suspected PAH, including idiopathic PAH (IPAH), PAH associated with systemic sclerosis (SSc-PAH) or chronic thromboembolic pulmonary hypertension (CTEPH). Exclusion criteria included World Health Organization (WHO) functional class IV patients, as well as a contraindication to either MRI or physical exercise, or recent syncope.
The study included simultaneous RHC and echocardiography at rest as well as during one or more stages of incremental exercise. MRI at rest was performed within 2 weeks of the RHC. All study procedures are summarized in Table 1, which includes the number of subjects who completed each test and the primary endpoints for each test. All patients gave written informed consent. The study was HIPAA-compliant and approved by the Institutional Review Boards of both institutions.
Table 1
Summary of the tests performed in this study.
Test | n | Primary endpoints |
---|---|---|
Clinical RHC | ||
![]() | 26 | Baseline hemodynamics |
Research RHC+echo | ||
![]() | 22 | RV pressure, PA pressure, PA flow, LAP |
![]() | 22 | PA pressure, PA flow, LAP |
![]() | 18 | PA pressure, PA flow, LAP |
![]() | 15 | PA pressure, PA flow, LAP |
MRI | ||
![]() | 18 | RV volume, EDV, ESV, SV, EF, SV/ESV |
RV pressure, PA pressure, PA flow, and RV volume data were collected as time-dependent waveforms, averaged over multiple heartbeats.
RHC = right heart catheterization; MRI = magnetic resonance imaging; RV = right ventricle; PA = pulmonary artery; LAP = left atrial pressure; EDV = end-diastolic volume; ESV = end-systolic volume; SV = stroke volume; EF = ejection fraction.
Cardiac catheterization
The RHC procedure was identical at the 2 sites. Participants underwent recording of invasive hemodynamics using a fluid-filled, 6F PA catheter (Edwards Lifesciences, Irvine, CA) and a properly zeroed pressure transducer. Systolic, diastolic, and mean PA pressure and pulmonary capillary wedge pressure (PCWP) were measured. Pulse pressure was calculated as systolic minus diastolic PA pressure. All hemodynamic pressure measurements were made at end-expiration. Cardiac output (CO) was calculated using thermodilution (average of 3 measurements) and indexed to body surface area (BSA) to calculate cardiac index (CI). Hemodynamic assessment also included RV stroke volume index (RV-SVI = CI/HR), total pulmonary vascular resistance (tPVR = mPAP/CO) and index (tPVRI = mPAP/CI), and PA compliance (CPA = RV-SVI/PAPP).
Pressure measurements at rest were repeated using a high-fidelity solid-state micromanometer-tipped catheter with either a custom 6F dual-sensor unit or a 3.5F single-sensor unit (Millar Instruments, Houston, TX), operating at 1 kHz. Prior to each catheterization, the pressure readout from the solid-state sensor was calibrated using a Veri-Cal™ pressure transducer tester (Utah Medical Products Inc., Midvale, UT).
Exercise protocol and echocardiography
Subjects performed exercise on a bicycle ergometer (Medical Positioning Inc., Kansas City, MO) mounted on the catheterization table. Starting from rest (0 W), the workload was gradually increased every 2–4 min by increments of 15 W until a maximum was achieved per subject exhaustion under careful supervision of a physician. PA pressure was recorded at the end of each stage of exercise. Transthoracic Doppler echocardiography (Vivid 7 or Vivid E9, GE Healthcare, Waukesha, WI) was used to measure blood velocity in the RV outflow tract, as previously described.[17] In addition, echocardiography was used to measure pulse wave Doppler early mitral inflow (E) velocity at the mitral valve leaflet tips, tissue Doppler early diastolic (e′) velocity at the lateral mitral annulus and RV outflow tract diameter. Tracings of PA pressure, pulse wave Doppler profile of flow through the RV outflow tract, and electrocardiographic (ECG) signals were synchronized using a dedicated hemodynamic analysis workstation (Cardiovascular Engineering Inc., Norwood, MA).
Magnetic resonance imaging
Cardiac MRI was performed at Northwestern Memorial Hospital on a 1.5T scanner (Avanto/Espree, Siemens Medical Systems, Erlangen, Germany) and at UW-Hospital, on a 3.0T scanner (MR750, GE Healthcare, Waukesha, WI). RV volume was assessed from ECG-gated, cine-balanced, steady-state, free-precession, axial images. Cycle-averaged areas were calculated for the length of the RV for 20 time frames using OsiriX Imaging Software, (revision 6.5, Pixmeo, Bernex, Switzerland). Areas were then summed into a single RV volume for each time point. From the resulting RV volume waveform and BSA, end-diastolic volume index (RV-EDVI), end-systolic volume index (RV-ESVI), stroke volume index (RV-SVI = RV-EDVI - RV-ESVI), and ejection fraction (RV-EF = RV-SVI/RV-EDVI) were calculated.
Analysis of pulmonary vascular characteristics
Figure 1A summarizes data collection and analytical methods used to assess pulmonary vascular function at rest and exercise. Synchronized PA pressure (from RHC) and flow (from echocardiography) were used to calculate Z0, which is a measure of tPVR, and ZC, which is a measure of proximal PA stiffness.
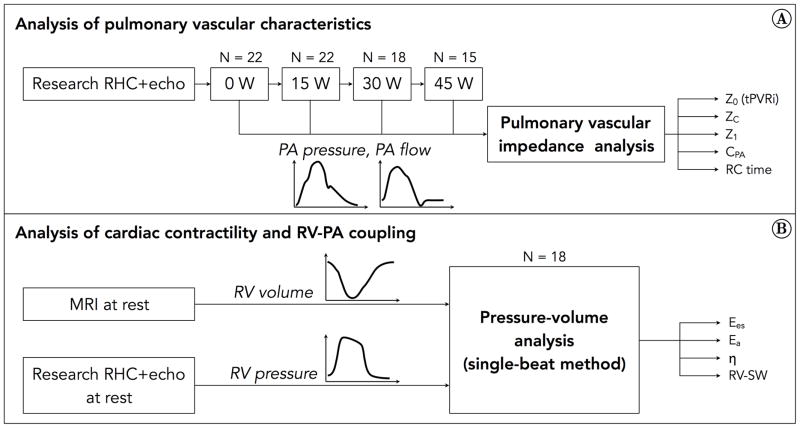
A) Pulmonary vascular impedance analysis was performed using PA pressure data from RHC and PA flow data from echocardiography, at rest and each exercise level. Not every subject was able to exercise up to 45 W, so the group size decreased with increasing exercise intensity. A frequency-domain analysis of the pressure-flow data was performed. Endpoints included various metrics of impedance, pulmonary arterial compliance, and RC time. B) Pressure-volume loop analysis was performed at rest using RV pressure data from RHC and RV volume data from MRI. A single-beat method was used for this analysis. Endpoints included Ees, Ea and η.
Pulmonary vascular impedance was calculated using frequency-domain analysis as the ratio of PA pressure to flow moduli,[18] and normalized by BSA. Z0 was taken as the zero-frequency value of the pulmonary vascular impedance modulus, whereas ZC was computed averaging the 4th through 10th harmonic. CPA was assessed as the ratio of RV-SVI (estimated from echocardiography) to PAPP. The RC time was assessed as the product of CPA and Z0. Left atrial pressure (LAP) was estimated from echocardiography as
as reported previously [19] and recently confirmed to be moderately accurate in patients with PAH.[20]
Analysis of cardiac contractility and RV-PA coupling
Cycle-averaged RV pressures (from RHC) were obtained from 15–25 beats to eliminate variability due to breathing and analyzed using custom LabVIEW routines (National Instruments, Austin, TX). RV pressure and volume waveforms were synchronized into a single pressure-volume loop (Figure 1B), from which maximum isovolumic pressure (Pmax), stroke work (RV-SW), end-systolic elastance (Ees) and arterial elastance (Ea) were computed using a single-beat method.[21] Coupling efficiency was computed as η = Ees/Ea, as well as using a volume-only method (SV/ESV) and a pressure-only method (Pmax/mPAP −1).[1]
Statistical analysis
All results are presented as mean ± standard error, unless indicated otherwise. The comparison between hemodynamics at rest and at maximum exercise was performed using a student’s t-test. The association between hemodynamics and incremental exercise level was analyzed using a linear mixed-effect model with repeated measures. Tukey’s honestly significant difference test was used as a post-hoc test of significance.
Comparisons between the low-η group (η below the median value) and high-η group (η above the median value) were performed using an unpaired t-test. Correlations between variables were investigated using Pearson’s correlation coefficient. The correlation between mPAP and CI was obtained for pooled data after performing Poon’s adjustment for individual variability.[22]
Results
Data were obtained from a study group of 26 patients. After accounting for patients who either declined MRI or had incomplete hemodynamic data, 22 patient datasets were usable for pulmonary vascular analysis at exercise, and 18 patient datasets were usable for cardiac contractility and RV-PA coupling analysis at rest. The demographics for the 26 patients included in the study are summarized in Table 2. One of the subjects included in this study had severe tricuspid regurgitation.
Table 2
Demographic data of the 26 patients included in the study group.
Variable | n | All subjects Mean ± SD | All subjects Range |
---|---|---|---|
Age (years) | 26 | 55.7 ± 10.6 | 36 – 78 |
Female (%) | 15 | 58 | |
BSA (m2) | 26 | 1.90 ± 0.28 | 1.46 – 2.70 |
Diagnosis (%) | |||
![]() | 7 | 27 | |
![]() | 13 | 50 | |
![]() | 6 | 23 | |
WHO class | |||
![]() | 1 | 4 | |
![]() | 11 | 42 | |
![]() | 14 | 54 | |
PAH medications (%) | |||
![]() | 11 | 42 | |
![]() | 4 | 15 | |
![]() | 6 | 23 | |
![]() | 3 | 12 | |
![]() | 1 | 4 | |
![]() | 1 | 4 | |
![]() | 11 | 42 |
BSA = body surface area; IPAH = idiopathic pulmonary arterial hypertension; SSc-PAH = pulmonary arterial hypertension associated with systemic sclerosis; CTEPH = chronic thromboembolic pulmonary hypertension; WHO = World Health Organization.
Effect of exercise on hemodynamics
Hemodynamic data obtained at rest and during exercise are summarized in Table 3. All measured and calculated parameters increased with exercise except LAP, tPVR, tPVRI, and RV-SVI. During RHC, patients were able to perform exercise up to a maximum workload of 46.7 ± 3.5 W, on average.
Table 3
Hemodynamic parameters measured at rest and maximum exercise level.
Variable | n | Rest | Maximum exercise | p-value |
---|---|---|---|---|
Workload | 22 | - | 45.7 ± 3.9 | - |
RHC + echo | 22 | |||
![]() | 65.6± 4.6 | 88.0± 5.9 * | < 0.001 | |
![]() | 29.8± 2.8 | 37.4±3.0 * | 0.0012 | |
![]() | 43.6 ± 3.3 | 58.8 ± 3.8 * | < 0.001 | |
![]() | 35.9 ± 2.9 | 51.2 ± 4.1 * | < 0.001 | |
![]() | 11.1 ± 0.8 | 12.2 ± 1.3 | 0.238 | |
![]() | 80.2 ± 2.7 | 111.8 ± 2.8 * | < 0.001 | |
![]() | 5.86 ± 0.34 | 8.41 ± 0.80 * | < 0.001 | |
![]() | 3.02 ± 0.16 | 4.28 ± 0.35 * | < 0.001 | |
![]() | 7.91 ± 0.74 | 8.17 ± 0.83 | 0.668 | |
![]() | 15.3 ± 1.4 | 15.8 ± 1.6 | 0.689 | |
![]() | 1.32 ± 0.17 | 0.88 ± 0.10 * | 0.0019 | |
![]() | 1.00 ± 0.07 | 0.67 ± 0.05 * | < 0.001 | |
![]() | 38.0 ± 1.9 | 39.1 ± 3.5 | 0.659 | |
![]() | 3.57 ± 0.38 | 4.63 ± 0.57 * | 0.0047 | |
![]() | 1.33 ± 0.15 | 2.31 ± 0.38 * | 0.026 | |
MRI | 18 | |||
![]() | 75.8 ± 8.9 | NA1 | - | |
![]() | 106.1 ± 8.8 | NA1 | - | |
![]() | 0.32 ± 0.03 | NA1 | - | |
RHC + MRI | 18 | |||
![]() | 2398 ± 296 | NA1 | - | |
![]() | 0.89 ± 0.13 | NA1 | - | |
![]() | 1.36 ± 0.25 | NA1 | - | |
![]() | 0.53 ± 0.09 | NA1 | - | |
![]() | 1.46 ± 0.16 | NA1 | - |
Values are expressed as mean ± SE.
At increasing levels of exercise, increases in mean pressure (mPAP) and mean flow (CI) were correlated. When data were normalized using Poon’s adjustment, the slope of the mPAP-CI curve was 8.70 mmHg/(L/min/m2), and the intercept was 18.98 mmHg (R2 = 0.764, p < 0.05), as reported in Figure 2. While Z0 did not change with exercise (Figure 3A), ZC and CPA changed significantly: from resting conditions to 45 W, ZC was nearly doubled (Figure 3B), whereas CPA decreased by about 21% (Figure 3C). The RC time was 1.00 ± 0.07 s at rest, and progressively decreased with exercise (Figure 3D). Online supplementary figure S1 shows a comparison between ZC from frequency-domain analysis (as reported in this study) and time-domain analysis.
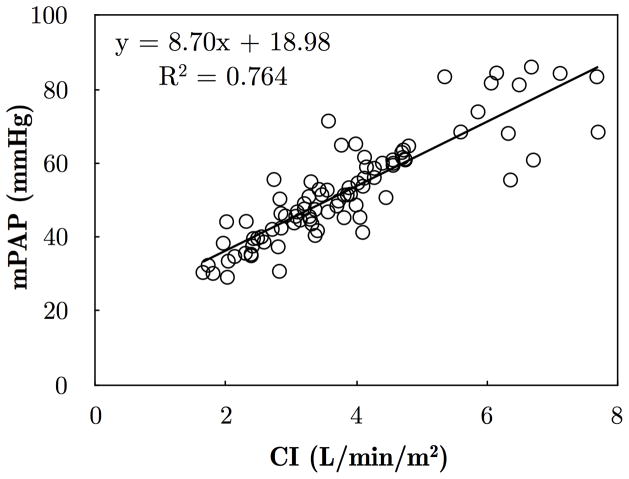
Pooled data include all patients who completed the exercise protocol, and were adjusted for individual variability following Poon’s method.[22] Pearce’s linear correlation (R2 = 0.764) is superimposed on the individual data points.
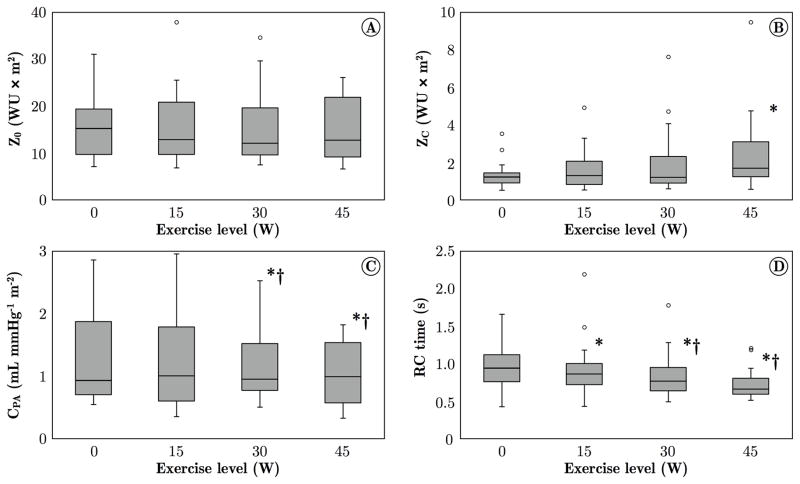
A) Pulmonary vascular resistance (Z0) did not change from rest to 45 W exercise, likely reflecting that in PAH patients the pulmonary vasculature is already fully recruited and distended at rest. B) Characteristic impedance (ZC) increased significantly from rest to 45 W. The exercise-induced increase in ZC is consistent with arterial strain-stiffening and vasoconstriction mechanisms, also observed in healthy subjects at incremental exercise.[12] C) PA compliance (CPA, normalized by BSA) progressively decreased with exercise, consistent with the increase in ZC. D) The RC characteristic time progressively decreased from rest to 45 W exercise. The resting RC time is consistent with observations in healthy controls.[12] The values obtained in this study are generally larger than in other studies in which PVR instead of tPVR was used to calculated the RC time.[23–26]
Data at the four exercise levels were obtained from 22 subjects (0 and 15 W), 18 subjects (30 W) and 15 subjects (45 W). *p < 0.05, compared to rest (0 W); †p < 0.05, compared to 15 W.
Reduced resting coupling efficiency is associated with decreased maximum exercise level
Hemodynamic coupling efficiency η at rest was assessed in the 18 patients who completed an MRI scan. This dataset was split in two groups, using the median of η as the threshold value. The maximum exercise level tolerated was compared between low-η (η = 0.49 ± 0.04) and high-η patients (η = 1.06 ± 0.15). As reported in Table 4, patients with lower η had a significantly lower tolerance to exercise. In fact, low-η patients were able to exercise up to 40.0 ± 5.6 W, compared to a 56.7 ± 4.2 W for the high-η patients (p < 0.05). For comparison, the analysis was repeated for hemodynamics typically assessed in the clinical setting (HR, CI, mPAP, PAPP, Z0), and for non-invasive coupling efficiency (SV/ESV). None of the metrics considered was significantly associated with the maximum exercise level tolerated (Table 4).
Table 4
Association of basic hemodynamics and coupling efficiency with maximum exercise level tolerated.
Variable | Wmax (W) | p-value | ||
---|---|---|---|---|
Name | Median | Below median | Above median | |
HR (bpm) | 80.0 | 51.0 ± 4.6 | 42.7 ± 5.6 | 0.284 |
CI (L/min/m2) | 3.05 | 43.8 ± 6.0 | 49.1 ± 4.6 | 0.491 |
mPAP (mmHg) | 38.8 | 45.0 ± 5.2 | 47.7 ± 5.7 | 0.726 |
PAPP (mmHg) | 41.0 | 49.6 ± 4.9 | 42.0 ± 5.8 | 0.327 |
Z0 (WU × m2) | 6.93 | 50.0 ± 4.6 | 42.3 ± 6.0 | 0.316 |
η | 0.68 | 40.0 ± 5.6 | 56.7 ± 4.2 * | 0.029 |
SV/ESV | 0.40 | 46.7 ± 5.8 | 50.0 ± 5.6 | 0.685 |
Wmax values are expressed as mean ± SE.
Data for basic hemodynamics (HR, CI, mPAP, PAPP, Z0) include all patients who completed the research RHC (N = 22); data for coupling efficiency (both η and SV/ESV) include all patients who completed the resting MRI (N = 18).
The slope of the mPAP-CI curve, after Poon’s adjustment, was 12.10 mmHg/(L/min/m2), and the intercept was 17.54 mmHg for the low-η patients (R2 = 0.769, p < 0.05). For the high-η patients, the slope was 9.19 mmHg/(L/min/m2), and the intercept was 13.25 mmHg (R2 = 0.833, p < 0.05). The difference in slope between the two groups was not significant.
Patients with reduced resting coupling efficiency exhibit increased arterial stiffness at rest and stiffening with exercise
The resting values of typically measured clinical hemodynamics and metrics of RV and PA function were compared between patients with low resting η and high resting η. This analysis included subjects who completed both MRI and research RHC at rest (N = 15). The two groups had similar RV contractility (Ees) and no significant difference in Ea (1.82 ± 0.47 vs. 0.88 ± 0.20 mmHg/mL, p = 0.106) but a lower resting η was associated with reduced CPA at rest (0.80 ± 0.06 vs 1.88 ± 0.34 mL/mmHg, p < 0.05), increased mPAP at rest (49.2 ± 4.0 vs 36.9 ± 3.5 mmHg, p < 0.05) and increased PAPP at rest (46.5 ± 1.6 vs 27.1 ± 5.0 mmHg, p < 0.05) (Table 5). The RC time at rest was significantly decreased in low-η patients (0.85 ± 0.06 vs 1.17 ± 0.13 s, p < 0.05). Resting RV volumes were not different between low-η and high-η, but RV-SW at rest was significantly nearly doubled in low-η patients (p < 0.05).
Table 5
Comparison of resting hemodynamics and RV and PA function between patients with low resting coupling efficiency (below median value) and patients with high resting coupling efficiency (above median value).
Variable | Low resting coupling efficiency (N = 8) | High resting coupling efficiency (N = 7) | p-value |
---|---|---|---|
HR (bpm) | 75.8 ± 4.4 | 78.3 ± 4.0 | 0.679 |
mPAP (mmHg) | 49.2 ± 4.0 | 36.9 ± 3.5 * | 0.040 |
PAPP (mmHg) | 46.5 ± 1.6 | 27.1 ± 5.0 * | 0.002 |
CI (L/min/m2) | 2.81 ± 0.28 | 3.21 ± 0.31 | 0.362 |
Z0 (WU × m2) | 9.17 ± 1.07 | 6.30 ± 1.07 | 0.083 |
Z1 (WU × m2) | 4.60 ± 0.50 | 2.74 ± 0.74 | 0.052 |
ZC (WU × m2) | 0.65 ± 0.11 | 0.59 ± 0.06 | 0.648 |
CPA (mL/mmHg/m2) | 0.80 ± 0.06 | 1.88 ± 0.34 * | 0.005 |
RC time (s) | 0.85 ± 0.06 | 1.17 ± 0.13 * | 0.039 |
RV-ESVI (mL/m2) | 90.9 ± 7.2 | 73.2 ± 16.7 | 0.327 |
RV-EDVI (mL/m2) | 122.0 ± 7.9 | 103.2 ± 15.3 | 0.277 |
RV-EF | 0.25 ± 0.04 | 0.33 ± 0.05 | 0.230 |
RV-SW (mL mmHg) | 3206 ± 451 | 1759 ± 238 * | 0.018 |
Ees (mmHg/mL) | 0.87 ± 0.25 | 0.88 ± 0.15 | 0.975 |
Ea (mmHg/mL) | 1.82 ± 0.47 | 0.88 ± 0.20 | 0.106 |
SV/ESV | 0.37 ± 0.08 | 0.52 ± 0.10 | 0.218 |
Pmax/mPAP - 1 | 1.53 ± 0.28 | 1.45 ± 0.24 | 0.837 |
Values are expressed as mean ± SE.
A positive linear correlation was found between η and CPA at rest (R2 = 0.733, p < 0.05). In addition, η at rest was negatively correlated with the change in CPA between 0 and 30 W (R2 = 0.646, p < 0.05), i.e. patients with lower resting η experienced a smaller decrease in PA compliance with exercise.
Discussion
This study sought to investigate the effect of exercise on PA stiffness in patients with PAH as well as the association of hemodynamic coupling efficiency at rest with exercise ability. One major result was that exercise induced stiffening of pulmonary arteries with no change in vascular resistance. Also, significant associations between coupling efficiency at rest and PA stiffness and maximum exercise level tolerated were demonstrated for the first time.
This is the first study to measure pulmonary vascular impedance in PAH patients during exercise. As a consequence, the findings of increased proximal PA stiffness, quantified by ZC, and decreased proximal and distal compliance, quantified by CPA, with exercise in PAH patients, are novel. These results are consistent with a recent report that exercise reduces compliance in both controls and CTEPH patients.[12] Since Z0 was preserved in the current study, meaning that an increase in CO was associated with an increase in mPAP, PA stiffening with exercise may be the result of (1) strain-stiffening of the proximal PAs due to the increase in mPAP, (2) exercise-induced sympathetic vasoconstriction due to the increase in CO, or (3) a combination of these mechanisms.
In the current study the RC time decreased significantly with exercise and the RC time at rest was significantly lower in low-η patients. The RC time is approximately constant in a wide range of conditions, both in healthy controls and PAH subjects.[23–26] Based on the assumption of a constant RC time, earlier reports postulated a limited contribution of proximal PA stiffness to pulmonary vascular compliance.[27] However, considering the current results as well as other reports that the RC time constant indeed may not always be constant,[12, 26, 28, 29] the role of proximal PA stiffening in PAH progression deserves further investigation, in particular under stress conditions.
The other major finding of the current study was the associations of coupling efficiency at rest with maximum exercise level, resting CPA, and CPA decrease during exercise. Reduced coupling efficiency has been documented in patients with PAH.[14, 15] The prognostic value of resting coupling efficiency has been demonstrated.[1] The current study further suggests that high resting η predicts the ability to accommodate an increase in cardiac output during exercise. In fact, high-η PAH patients have better (i.e., higher) CPA at rest than low-η patients, and during exercise they can reduce CPA to a greater extent. These results suggest that PAH patients with more efficient hemodynamic coupling also have a greater pulmonary vascular reserve, which can be used to hemodynamic advantage when cardiac output increases, resulting in improved tolerance to exercise compared to low-η patients. These findings are consistent with the comparison between exercise-induced CPA reduction in CTEPH patients and healthy controls.[12] In contrast with basic hemodynamics typically assessed clinically, resting coupling efficiency showed a significant association with exercise tolerance. This result indicates that the main reason why patients with PAH become progressively unable to tolerate exercise is the decline in the efficiency in the hemodynamic interaction between ventricle and vasculature, rather than either ventricular or vascular function impairment alone. Assessing resting η from MRI and RHC data may have an important clinical value. Simultaneous MRI and invasive RV pressure recordings is an attractive approach, although not yet clinically viable.[30]
The separation between high-η and low-η PAH patients revealed a number of significant differences between the two groups, which may be analogous to differences between healthy subjects and PAH patients. For instance, the association between reduced η and limited exercise capacity has been observed previously in a comparison between healthy subjects and PAH patients.[16] Also, the mPAP-CI curve was about 32% steeper for patients with low-η compared to high-η in our study, although this difference was not significant, which is consistent with an increase in mPAP-CI slope in patients with pulmonary vascular disease compared to those without.[10, 12]
The comprehensive approach to evaluation of cardiopulmonary performance used in this study (RHC and echocardiography during exercise) is viable for research, but it is unlikely to be used clinically. The clinical significance of this study is pulmonary vascular hemodynamic reserve is an important contributor to exercise intolerance in PAH, based on the relationships found between PA stiffening and ventricular-vascular coupling efficiency at rest and during exercise. Importantly, prior to this study it was unclear whether proximal PAs stiffen during exercise independent of changes in resistance.
These results may help guide the clinical adoption of exercise testing protocols, which have been increasingly recommended to monitor PAH progression.[5] Exercise testing protocols generally include measures of PA resistance only (PVR, mPAP-CI curves). This study suggests that the response to exercise of proximal PAs may also need to be monitored, ideally non-invasively using stress echocardiography.
Study limitations
The number of patients included was limited due to the complex, invasive protocol; therefore, the clinical implications of these results should be confirmed in larger studies. Also, this study did not include a disease-free group. PAH was due to different causes in this study, and the size of the subtype groups was insufficient to investigate this effect. Similarly, sex differences were not investigated. Fifteen patients were on pulmonary vasodilator treatment at the time of this study. These medications may affect cardiopulmonary function and response to exercise; however, an analysis of the data at rest and maximum exercise did not indicate any (data not shown).
PCWP could not be measured during exercise. Instead, PCWP was estimated echocardiography, which may have limited accuracy. Because of the short time available at each exercise increment, the exercise echocardiography protocol was limited to the measurements required for the pulmonary vascular impedance analysis.
Conclusions
In patients with PAH, exercise-induced stiffening of pulmonary arteries aggravates RV afterload. PA stiffening was unrelated to changes in resistance, resulting in a progressive decrease in the RC time with exercise. Patients with less efficient hemodynamic coupling at rest exhibited a reduced pulmonary vascular reserve, which likely limited the maximum exercise level tolerated. The results of this comprehensive approach to evaluating cardiopulmonary performance may shed light on mechanical mechanisms of PAH progression and exercise tolerance, which in turn may lead to better, less invasive prognostic indicators. In addition, therapeutic strategies targeting the efficiency of hemodynamic coupling may be beneficial to exercise tolerance in PAH patients and improve quality of life.
Acknowledgments
Funding
The authors gratefully acknowledge funding support from NIH 1R01HL105598 (NCC).
Footnotes
The Corresponding Author has the right to grant on behalf of all authors and does grant on behalf of all authors, an exclusive license on a worldwide basis to the BMJ Publishing Group Ltd and its Licensees to permit this article (if accepted) to be published in HEART editions and any other BMJPGL products to exploit all subsidiary rights.
Contributorship
AB, JRR, SJS and NCC contributed to the conception and design of the study. RS and JGK contributed to the acquisition, analysis and interpretation of the clinical data. LBN and MB contributed to the acquisition, analysis and interpretation of the echocardiography data. MJC and CJF contributed to the acquisition, analysis and interpretation of MRI data. AB, ED, HM, SJS and NCC contributed to the analysis and interpretation of data. AB, SJS, RN and NCC drafted the manuscript and all authors contributed to revising it critically. All authors have read and had final approval of the manuscript.Competing interests
None.
All authors have reported that they have no relationships to disclose.
References
Full text links
Read article at publisher's site: https://doi.org/10.1136/heartjnl-2016-309906
Read article for free, from open access legal sources, via Unpaywall:
https://europepmc.org/articles/pmc5326695?pdf=render
Citations & impact
Impact metrics
Citations of article over time
Alternative metrics
Smart citations by scite.ai
Explore citation contexts and check if this article has been
supported or disputed.
https://scite.ai/reports/10.1136/heartjnl-2016-309906
Article citations
LOXL2 inhibition ameliorates pulmonary artery remodeling in pulmonary hypertension.
Am J Physiol Lung Cell Mol Physiol, 327(4):L423-L438, 16 Jul 2024
Cited by: 0 articles | PMID: 39010824
A novel complement C3 inhibitor CP40-KK protects against experimental pulmonary arterial hypertension via an inflammasome NLRP3 associated pathway.
J Transl Med, 22(1):164, 16 Feb 2024
Cited by: 2 articles | PMID: 38365806 | PMCID: PMC10870435
Distensibility, an Early Disease Marker of Pulmonary Vascular Health: Ready for Clinical Application.
J Am Heart Assoc, 12(20):e031605, 10 Oct 2023
Cited by: 0 articles | PMID: 37815032 | PMCID: PMC10757520
Classification and Predictors of Right Ventricular Functional Recovery in Pulmonary Arterial Hypertension.
Circ Heart Fail, 16(10):e010555, 04 Sep 2023
Cited by: 2 articles | PMID: 37664964 | PMCID: PMC10592283
The physiological basis of pulmonary arterial hypertension.
Eur Respir J, 59(6):2102334, 16 Jun 2022
Cited by: 48 articles | PMID: 34737219 | PMCID: PMC9203839
Review Free full text in Europe PMC
Go to all (14) article citations
Data
Data behind the article
This data has been text mined from the article, or deposited into data resources.
BioStudies: supplemental material and supporting data
Similar Articles
To arrive at the top five similar articles we use a word-weighted algorithm to compare words from the Title and Abstract of each citation.
RV contractility and exercise-induced pulmonary hypertension in chronic mountain sickness: a stress echocardiographic and tissue Doppler imaging study.
JACC Cardiovasc Imaging, 6(12):1287-1297, 23 Oct 2013
Cited by: 24 articles | PMID: 24269266
Accuracy of Echocardiography to Evaluate Pulmonary Vascular and RV Function During Exercise.
JACC Cardiovasc Imaging, 9(5):532-543, 21 Oct 2015
Cited by: 66 articles | PMID: 26508387
Sex-Related Differences in Dynamic Right Ventricular-Pulmonary Vascular Coupling in Heart Failure With Preserved Ejection Fraction.
Chest, 159(6):2402-2416, 01 Jan 2021
Cited by: 10 articles | PMID: 33388286
Methods for Evaluating Right Ventricular Function and Ventricular-Arterial Coupling.
Prog Cardiovasc Dis, 59(1):42-51, 05 Jul 2016
Cited by: 24 articles | PMID: 27393072
Review
Funding
Funders who supported this work.
NHLBI NIH HHS (1)
Grant ID: R01 HL105598
National Heart, Lung, and Blood Institute (1)
Grant ID: 1R01HL105598