Abstract
Free full text

The role of m6A modification in the biological functions and diseases
Abstract
N6-methyladenosine (m6A) is the most prevalent, abundant and conserved internal cotranscriptional modification in eukaryotic RNAs, especially within higher eukaryotic cells. m6A modification is modified by the m6A methyltransferases, or writers, such as METTL3/14/16, RBM15/15B, ZC3H3, VIRMA, CBLL1, WTAP, and KIAA1429, and, removed by the demethylases, or erasers, including FTO and ALKBH5. It is recognized by m6A-binding proteins YTHDF1/2/3, YTHDC1/2 IGF2BP1/2/3 and HNRNPA2B1, also known as “readers”. Recent studies have shown that m6A RNA modification plays essential role in both physiological and pathological conditions, especially in the initiation and progression of different types of human cancers. In this review, we discuss how m6A RNA methylation influences both the physiological and pathological progressions of hematopoietic, central nervous and reproductive systems. We will mainly focus on recent progress in identifying the biological functions and the underlying molecular mechanisms of m6A RNA methylation, its regulators and downstream target genes, during cancer progression in above systems. We propose that m6A RNA methylation process offer potential targets for cancer therapy in the future.
Introduction
Epigenetics is a discipline that modulates heritable gene expression without DNA sequence changes. There are many types of well documented epigenetic modifications, such as DNA methylation, histone modification, chromatin remodeling, and non-coding RNA regulation, among which, the methylation modification of DNA and RNA is extremely important1. With the rapid development of specific antibodies and high-throughput sequencing, N6-methyladenine modification has made tremendous breakthroughs from prokaryotic bacteria to eukaryotic human being2. m6A modification has been identified as one of the post-transcriptional regulatory markers in different types of RNAs, such as messenger RNAs (mRNAs), transfer RNAs (tRNAs), ribosomal RNAs (rRNAs), circular RNAs (circRNAs), micro RNAs (miRNA), and long non-coding RNAs (lncRNAs)3,4. Furthermore, RNA m6A modification has been documented to play important roles for regulating RNA splicing, translation, stability, translocation, and the high-level structure4–6. The landscape of m6A in the transcriptome was first uncovered by next-generation sequencing (NGS)7,8, which showed that an average of 3–5 m6A modifications in each mRNA out of one-third of mammalian total mRNAs. Until now, m6A modification has been identified on almost every type of RNAs. MeRIP-seq (methylated RNA immunoprecipitation and sequencing) has been broadly used to profile m6A, detecting m6A containing region around ~100 nucleotide (nt) length7,8. However, the exact location of individual m6A site could not be efficiently and accurately identified, although multiple improved methods have been developed, such as PA-m6A-seq, miCLIP, and m6A-CLIP9–12. Most m6A sites were found in conserved motif DRACH (D=
G/A/U, R
=
G/A, H
=
A/U/C)13, which have been frequently identified around the stop codon by whole-transcriptome m6A maps, indicating the potential functional roles for m6A7,8,14. To detect individual m6A sites, methyl-sensitive ligase, reverse transcriptase and selective dTTP (deoxythymidine triphosphate) analog have been applied15–18.
To examine the chemical properties of m6A sites, all of the above methods dependent on m6A-specific antibodies, which can also recognize the structurally similar cap modifications, leading to poor reproducibility and even controversial result. To solve this problem, Luo’s group recently has developed m6A-sensitive RNA-endoribonuclease–facilitated sequencing method or m6A–REFseq, which can identify transcriptomic m6A sites at specific motifs in single-base resolution19. By applying m6A-REF-seq to different tissues from human, mouse and rat, m6A modification was shown to be highly conserved at both gene and individual base level19. Similarly, Schwartz’s group has developed MAZTER-seq to quantify m6A stoichiometry at individual sites, which highly relies on the ability of the bacterial RNase MazF to cleave RNA immediately upstream of an “ACA” sequence, but not upstream of “m6A-CA”20. Then, Meyer’s group presented DART-Seq (deamination adjacent to RNA modification targets), also an antibody-independent method for detecting individual m6A sites. Meyer et al. altered the sequence near methylation sites by fusing APOBEC1 to the m6A-binding YTH domain, and detected subsequent editing events with RNA-Seq21. With the help of these methods based on single-base resolution, we will be able to apply precious primary patient material or limited primary cancer stem cells to examine the m6A profiling under different physiological and pathological conditions, which could be used as novel biomarkers in the future.
m6A is installed by methyltransferase complex including METTL322, METTL1423, WTAP24, KIAA142925, METTL1626, RBM1527, and ZC3H1328. m6A is removed by demethylases such as FTO29 and ALKBH530. The m6A reader proteins can recognize the m6A-modified RNAs, which are divided into different protein families. One class of direct m6A readers proteins contain the YT521-B homology (YTH) domain4, and several heterogeneous nuclear ribonucleoproteins (HNRNPs) fall into the other category, which mainly regulate alternative splicing or processing of target transcripts31. IGF2 mRNA binding proteins (IGF2BP1/2/3) families32, and eukaryotic initiation factor (eIF) 333, belong to another subfamily members. Different species choose different m6A reader proteins to carry out specific biological functions. For example, in order to adapt to a hypoxia environment, mammals living in high altitude select the YTHDF1, instead of YTHDF2/3, to resist hypoxia-induced cellular apoptosis in a Keap1-Nrf2 axis dependent manner34, suggesting that readers of individual m6A-modified RNAs can have both redundant and specific roles depending on different cellular context.
Numerous studies focusing on m6A RNA methylation have demonstrated that the regulators of m6A RNA methylation are involved in various human diseases, including Nonalcoholic fatty liver disease35, Azoospermia36, heart failure37, especially in human cancers38. In this review, we discuss how m6A RNA methylation influences both the physiological and pathological progressions of hematopoietic, central nervous and reproductive systems. We will mainly focus on recent progress in identifying the biological functions and the underlying molecular mechanisms of m6A RNA methylation, its regulators and downstream target genes, during cancer progression in above systems. We propose that m6A RNA methylation represents the potential target for cancer therapy in the future.
The writers, erasers, and readers of m6A
m6A is installed by the m6A methyltransferases complex, which is a dynamical and reversible biological process (Table (Table1).1). As the first discovered methyltransferase and the core methyltransferase subunit, METTL3 plays a major catalytic role in m6A addition process. It has been well documented that METTL3 methylates its specific target transcripts and participates in various physiological processes, such as embryonic development39, brain development40, spermatogenesis41, cell reprogramming39, and T cell homeostasis42. METTL14, another key enzyme of methyltransferase complex, interacts with METTL3 and forms a stable heterodimer playing an essential role during m6A deposition on nuclear RNAs with increased catalytic efficacy23,43. In addition, deletion of METTL14 inhibits self-renewal and differentiation abilities of embryonic stem cell44. METTL16 has also been indicated to play as a methyltransferase for the U6 spliceosomal small nuclear RNA, which is required for SAM homeostasis45. Wilms’ tumor 1-associating protein (WTAP) forms protein complex with METTL3 and METTL14, deletion of which results in reduced RNA-binding capability of methyltransferase complex and embryonic differentiation46. It has been shown that the loss of RNA-binding motif protein 15 and its paralogue RBM15B (RBM15/RBM15B), results in impaired XIST-mediated gene silencing on the X chromosome during mammalian female development47,48. A recent study shows that KIAA1429 is able to recruit and guide the catalytic core methyltransferase components (METTL3/METTL14/WTAP) to specific RNA region for m6A methylation49. In Drosophila and mice, it has recently been documented that Zc3h13 (zinc finger CCCH domain-containing protein 13) interacts with Flacc (Fl(2)d-associated complex component) as a novel cofactor of m6A methyltransferase complex, which controls the overall m6A levels in sex determination in Drosophila50.
Table 1
The functional roles of m6A regulators in RNA metabolism.
Type | M6ARegulator | Function | References |
---|---|---|---|
m6A writer | METTL3 | Catalyzes m6A modification | 22 |
METTL14 | Assists METTL3 to recognize the subtract | 25 | |
METTL16 | Catalyzes m6A modification | 26 | |
WTAP | Promotes METTL3-METTL14 heterodimer to the nuclear speckle | 24 | |
KIAA1429 | Guides the methyltransferase components to specific RNA region | 25 | |
RBM15 | Binds the m6A complex and recruit it to special RNA site | 47 | |
ZC3H13 | Bridges WTAP to the mRNA-binding factor Nito | 50 | |
m6A eraser | FTO | Removes m6A modification | 29 |
ALKBH5 | Removes m6A modification | 30 | |
m6A reader | YTHDC1 | Promotes RNA splicing and translocation | 55 |
HNRNPA2B1 | Promotes primary microRNA processing | 60 | |
HNRNPC | Mediates mRNA splicing | 58 | |
YTHDF1 | Promotes mRNA translation | 58 | |
YTHDF2 | Reduces mRNA stability | 232 | |
YTHDF3 | Mediates the translation or degradation | 232 | |
YTHDC2 | Enhances the translation of target RNA | 119 | |
IGF2BP1/2/3 | Enhances mRNA stability | 32 |
The demethylases play as erasers to remove the m6A modifications in RNA. In 2011, one study reported that fat mass and obesity-associated protein (FTO) localizes in nuclear speckles to eliminate m6A residues in RNA29. A genome-wide screen for type 2 diabetes susceptibility genes identified a common variant in FTO predisposing to obesity through an effect on body mass index (BMI)51. FTO has also been reported to regulate the adipogenesis via regulating alternative splicing of adipogenic transcription factor RUNX1T1 (Runt-related transcription factor 1) in an m6A depend manner52. ALKBH5 was the second identified m6A demethylase, which has been shown to modulate mRNA export and RNA metabolism by reducing the m6A level in nuclear speckles30. Inactivation of ALKBH5 leads to male infertility in mice through appropriate m6A clearance in the nuclei of spermatocytes essential for correct splicing and the production of longer 3′-UTR mRNAs, and failure of which leads to aberrant splicing and accumulation of shorter transcripts53. DDX46, one member of DEAD-box (DDX) helicases, has been shown to inhibit antiviral innate responses by recruiting ALKBH5 and entrapping selected antiviral transcripts in the nucleus through erasing their m6A modification54.
The m6A reader protein can recognize and bind to the m6A-modified transcript regulating gene expression through regulating diverse processes, such as mRNA stability52, mRNA splicing55, mRNA structure56, mRNA export57, translation efficiency58, and miRNA biogenesis59. Different readers have different m6A positioning function, nuclear m6A readers including YTHDC1, HNRNPA2B1, HNRNPC11, and HNRNPG. A recent study showed that YTHDC1 promotes exon inclusion in targeted transcripts by selectively recruiting pre-mRNA splicing factor SRSF3 while blocking SRSF10 mRNA binding55. The nuclear export of m6A methylated transcripts may be facilitated by YTHDC1 through interacting with nuclear transport receptors57. Another study demonstrated that YTHDC1 recognizes m6A-modified XIST to promote XIST-mediated X chromosome silencing47. It has been shown that hnRNP A2/B1 directly binds to and regulates the processing of m6A-modified transcripts, including subset of primary miRNA transcripts by interacting with the miRNA microprocessor complex protein DGCR831. Similarly, a structure-based study revealed that hnRNP A2/B1 recognizes specific targets containing AGG and UAG motifs by RRM1 and RRM2 domains, respectively through an “m6A switch” mechanism60. Cytoplasmic m6A readers contain YTHDF1/2/3, YTHDC2, and IGF2BP1/2/3. YTHDF1 enhances translation by interacting with translation initiating factors and ribosomes58. YTHDF2 promotes targeted mRNA decay via recruiting RNA decay machinery factors58. YTHDF3 was reported to promote protein synthesis synergizing with YTHDF1, and regulate m6A-modified mRNA decay mediated by YTHDF261. YTHDC2 plays an essential role on the switch from mitosis to meiosis during spermatogenesis62. IGF2BPs, the conserved single-stranded RNA-binding proteins (RBPs), structurally contain six canonical RNA-binding domains, two RNA recognition motif (RRM) domains, and four K homology (KH) domains63. IGF2BPs can enhance mRNA stability by binding to target transcripts through GG(m6A)C, a typical m6A motif64. Different reader proteins present different even opposite functions depending on different cellular context65 (Fig. (Fig.11).
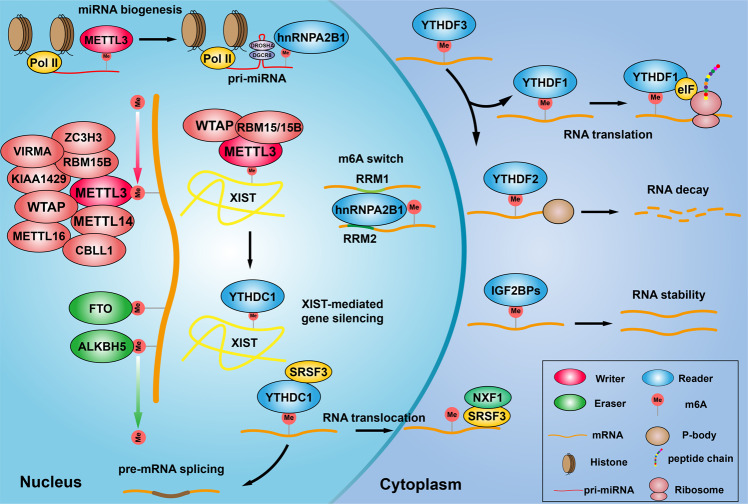
Introduction of m6A RNA modification complex. m6A methylation is catalyzed by the writer complex including METTL3, METTL14, METTL16, WTAP, VIRMA, RBM15/15B, CBLL1, KIAA1429, and ZC3H13. The m6A modification is erased by demethylases including FTO and ALKBH5. The m6A-modified RNA reader proteins include YTHDF1/2/3, YTHDC1/2, IGF2BP1/2/3, and HNRNPC/A2B1. m6A modification modulates miRNA biogenesis, XIST-dependent X chromosome inactivation, m6A switch, RNA translocation, pre-mRNA splicing, RNA translation, RNA decay and RNA stability
Hematopoietic, central nervous and reproductive systems
There are more than 10 different cell types with various functions in hematopoietic system. For examples, leukocytes are mainly involved in innate and acquired immunity; erythrocytes provide O2 and CO2 exchange and transport; megakaryocytes generate platelets during wound healing. Most importantly, hematopoietic or blood system, which sustains the nutrition and O2 bases for all the other animal tissues, develops in several waves during embryogenesis. The first wave emerges from the yolk sac at embryonic day 7.5 (E7.5) in mice66,67, which is characterized by the generation of nucleated megaloblastic erythroblasts referred to as primitive erythroblasts (PEs), together with diploid platelet progenitor cells and macrophages68. PEs are characterized by the predominant expression of embryonic globin genes, which eventually enucleate in the circulation69,70. The second wave has been linked to erythro-myeloid progenitors (EMPs) occurring in the yolk sac, which generates erythroid colonies similar to those derived from adult bone marrow (BM) and eventually migrate to the fetal liver and produce erythroblasts66,71,72. The third wave correlates with hematopoietic stem cells (HSCs) produced from the hemogenic endothelium identified in the dorsal aorta of the aorta-gonad-mesonephros (AGM) region, which can also emerge from umbilical, vitelline, yolk sac, cranial, and placental regions67. In addition, before HSCs finally become quiescent in the BM, they migrate to the fetal liver and undergo an expansion period73. Until now, many studies have demonstrated that epigenetic modification including DNA methylation, histone modification, and m6A modification plays important roles during hematopoietic development74–84.
The central nervous system (CNS) is a complex network composed of different types of nervous cells. During development, neural stem/progenitor cells have the potential to self-renewal, which can differentiate to produce various types of nervous cells, including neurons, astrocytes, and oligodendrocytes85. By using the retroviral lineage-tracing methods in mouse cortex, researchers found that neural stem/progenitor cells at midgestation generated small clones that could migrate quite widely86–88, and traced that these earlier infected clones could span multiple cortical layers with multipotency for neuronal production89. Embryonic neural progenitor cells (NPCs) are mainly classified into multipotent neural stem cells and transit amplifying/intermediate progenitor cells (IPCs)90. Hereafter, more results showed that most embryonic progenitors produce clones of neurons, while others of glia, but only a small population produce both neuronal and glial progeny91–95. Recently studies have demonstrated that neural stem/progenitor cells reside in the adult mammalian brain and contribute to brain plasticity throughout life96,97. These discoveries led to the concept that the CNS development is similar to that of the hematopoietic system, which relies on multipotent stem cells that have capacity for self-renewal and potency to produce more types of differentiated progeny. Yet today, although the key aspects of the mechanisms that underlie neural stem/progenitor cells remain enigmatic, many results have shown that various types of epigenetic modifications including m6A modification play critical roles in regulating the maintenance and differentiation of NSCs98–106.
Early in development, the germ cell lineage undergoes a series of complex developmental processes that culminate in the generation of the oocytesthe and spermatozoa107. In mouse, the germ cell lineage emerges in the most proximal posterior epiblasts at the beginning of gastrulation around embryonic day (E) 6.0108,109. Human PGCs migrate to the gonadal ridge (the precursor of the gonads) at approximately week 5 postfertilization and undergo proliferation before sexual differentiation110,111. The mouse primordial germ cells (PGCs) then migrate through the hindgut endoderm and mesentery and colonize the embryonic gonadal primordia111–113. In males, mPGCs continue to proliferate in embryonic testes until they enter into mitotic arrest and differentiate into gonocytes. In females, mPGCs undergo proliferation as cysts in embryonic ovaries until they enter into the first meiotic prophase and differentiate into oocytes113–115. Epigenetic reprogramming including m6A modification, DNA demethylation, and histone modification has been indicated to be crucial for this complex process41,116–119.
Based on the following facts that (1) the critical roles of hematopoietic, central nervous and reproductive systems for individual and the species, and (2) the continuous existence of multipotent stem cells under physiological conditions throughout life-time of animals and human, and (3) numerous documented findings corroborating that epigenetic reprogramming especially RNA m6A modification plays crucial roles in hematopoietic, central nervous and reproductive systems, we decided to mainly summarize and discuss how m6A RNA methylation influences both the physiological and pathological progressions in the above three systems (Table (Table22).
Table 2
The functional roles of m6A modification complex during normal development in hematopoietic, central nervous, and reproductive systems.
Organs | m6A regulator | Cells /Organisms | Effect of gene knockdown/depletion | Mechanism | References |
---|---|---|---|---|---|
Hematopoietic | METTL3 | mHSPCs | ↓Endothelial to hematopoietic transition | ↓METTL3/YTHDF2↑/notch1a↓ | 84 |
METTL3 | HSPCs | ↑Differentiation, ↓cell proliferation | ↑METTL3/↑c-MYC/BCL2/ PTEN | 171 | |
METTL3/L14 | mESCs | ↓Self-renewal | ↓METTL3/14/HuR↓/↓IGFBP3 | 43 | |
YTHDF2 | HSCs | ↑Regeneration | ↓YTHDF2/ ↑Wnt target genes | 124 | |
METTL3 | HSCs | ↓Proliferation, ↓differentiation | ↓METTL3/ ↑MDA5/RIG-I | 125 | |
Central nervous | METTL3/L14 | RGCs | ↑Neurogenesis, ↑cell cycle | ↓METTL3/14/↑Tbr2/Neurog2/Neurod1 | 106 |
METTL14 | OPCs | ↓Oligodendrocyte numbers | ↓METTL14/↓NF155 | 129 | |
ALKBH5 | RGCs | ↑Proliferation, ↑differentiation | ↓ALKBH5/ ↓Cacna2d3/Notch3/ Jam3 | 131 | |
FTO | NSCs | ↓Proliferation, ↓differentiation | ↓FTO/ ↓BDNF/PI3K/ Akt2/Akt3 | 127 | |
YTHDF1 | Mice | ↓Learning, memory defects | ↓YTHDF1/↓Camk2a | 133 | |
YTHDF1 | Mouse | ↓Axon guidance | ↓YTHDF1/↓Robo3.1 | 105 | |
YTHDF2 | mNSPC | ↓Self-renewal | ↓YTHDF1/↓JAK-STAT | 135 | |
YTHDF1/3 | Mouse | ↓Synaptic transmission | ↓YTHDF1/3/↓GluA1 | 136 | |
PRRC2A | OPCs | ↓Proliferation | ↓PRRC2A/↓Olig2 | 137 | |
Reproductive | YTHDF2 | Mouse | ↓Oocyte maturation | ↓YTHDF2/↓Trpc5 | 139 |
METTL3 | Zebrafish | ↓Sperm motility | ↓METTL14/↓11-KT/ 17β-E2 | 140 | |
YTHDC1 | Germ cells | ↓Oocyte growth, maturation | ↓YTHDC1/↓CPSF6/ SRSF3 | 141 | |
ALKBH5 | Mice | ↓Fertility | ↓ALKBH5/↑Dnmt1 | 30 |
m6A RNA modification regulates hematopoietic development
The hematopoietic system provides the lifelong supply of blood cells, which are derived from a rare population of multipotent HSCs120. Recent studies have indicated that m6A modification of RNAs plays pivotal roles during hematopoietic development, which is an essential process for blood system maturation121. The first evidence showed that m6A RNA modification determines cell fate during the endothelial-to-hematopoietic transition (EHT) to specify the earliest hematopoietic stem/progenitor cells (HSPCs) during Zebrafish embryogenesis, and METTL3 knockdown significantly inhibits EHT in a Notch signaling dependent manner84. Consistently, another study showed that depletion of METTL3 in vascular endothelial cells significantly represses the function of HSPCs122. However, shRNA-mediated knockdown of the METTL3 in human hematopoietic stem/progenitor cells (HSPCs) preferentially promotes cell differentiation but not cell proliferation, and vice versa123. Similarly, result has been shown that deletion of METTL3 and METTL14 leads to loss of self-renewal capability in mouse embryonic stem cells (mESCs)43. In addition, YTHDF2 was identified to regulate transcriptome switch during zebrafish maternal-to-zygotic transition84, and another study showed that YTHDF2 depletion is able to expand mouse and human hematopoietic stem cells dramatically, highlighting its potential role in clinical blood transplantation124. Furthermore, loss of METTL3 in the murine fetal liver can promote the formation of endogenous double-stranded RNAs (dsRNAs), which activates MDA5-RIG-I, PKR-eIF2α, and OAS-RNase L signaling pathways in hematopoietic stem/progenitor cells, resulting in hematopoietic failure and perinatal lethality125. The above findings indicate that m6A RNA methylation plays different roles during different developmental stages in various tissues. Thus, deciphering the underlying molecular mechanism will help us to better understand the mechanism of development and how to treat human diseases in the future (Fig. (Fig.22).

m6A RNA modification regulates hematopoietic system development. In the hematopoietic system, m6A methylation is essential for the proliferation and differentiation of hematopoietic stem/progenitor cells. Depletion of METTL3 promotes the formation of endogenous double-stranded RNAs (dsRNAs), which activates MDA5-RIG-I, PKR-eIF2α, and OAS-RNase L signaling pathways in hematopoietic stem/progenitor cells, resulting in hematopoietic development failure. YTHDF2 inhibits the Wnt signaling pathway by degrading the mRNA of ccnd1, c-Myc and Axin2, leading to reduced the proliferation and differentiation of hematopoietic stem/progenitor cells. METTL3 promotes the translation of c-Myc, PTEN, and BCL2 by increasing the methylation levels of reciprocal mRNAs, and promotes the proliferation of stem cells. METTL3 and YTHDF2 cooperate to inhibit the Notch signaling pathway in hematopoietic system
m6A RNA methylation regulates CNS development
Emerging studies have indicated that m6A is more abundant in the CNS than that in other organs, which increases in overall abundance from the embryonic to adult brain, suggesting its critical roles during normal brain development and function40. Recent studies have demonstrated that m6A modification modulates adult neurogenesis in the mammalian midbrain40, embryonic brain development126, learning, and memory127. For example, depletion of either METTL3 or METTL14 in the embryonic mouse brains prolongs the cell cycle of radial glia cells and extends cortical neurogenesis into postnatal stages. In addition, m6A signaling has also been indicated to regulate human cortical neurogenesis in forebrain organoids128. In vivo conditional ablation of METTL14 leads to reduced oligodendrocyte numbers and CNS hypomyelination. Furthermore, depletion of Mettl14 disrupts postmitotic oligodendrocyte maturation129. Depleting METTL3 reduces memory consolidation ability in mouse hippocampus, while overexpression of METTL3 significantly enhances long-term memory consolidation130. Forced expression of METTL3 using lentivirus infection results in the disorganized structure of both Purkinje and glial cells.
Under hypoxia conditions, deletion of ALKBH5 has been shown to promote cell proliferation and differentiation in the cerebellum by destroying the balance of RNA m6A methylation in different cell fate determination genes131. FTO was highly expressed in adult neural stem cells (NSCs) and neurons, loss of which not only reduces the proliferation and neuronal differentiation of NSCs, but also results in decreased brain size and body weight, leading to impaired learning and memory127. In the mouse brains, YTHDF1, one of the YTH domain-containing m6A-modified mRNA binding protein family members, whose mRNA is preferentially increased in the hippocampus, a key region in spatial learning and memory132. YTHDF1 was also found to promote protein translation of targeted m6A-modified transcripts in response to neuronal stimuli in the adult mouse hippocampus, thereby facilitating learning and memory133. Importantly, recent findings showed that YTHDF1 binds to and promotes translation of m6A-modified Robo3.1 transcript, which is essential for midline crossing of spinal commissural axons105. It has been shown that sciatic nerve lesion increases m6A-modified transcripts encoding many regeneration associated genes in the adult mouse dorsal root ganglion (DRG), and inhibition of METTL14 or YTHDF1 dramatically reduces injury induced functional axon regeneration134. Similar results showed that depletion of YTHDF2 inhibits NSCs self-renewal and spatiotemporal generation of neurons in embryonic neocortex, leading to lethality at late embryonic developmental stages135. One in vitro study showed that knockdown of YTHDF1 or YTHDF3 m6A readers, respectively, in cultured primary hippocampal neurons, causes decreased spine head volume and dampened spontaneous excitatory synaptic transmission136. A novel m6A reader PRRC2A (Proline rich coiled-coil 2A) was found to control oligodendrocyte specification and myelination. Conditional ablation of PRRC2A induces marked hypomyelination, cognitive defects in mouse, and decreased lifespan137 (Fig. (Fig.33).
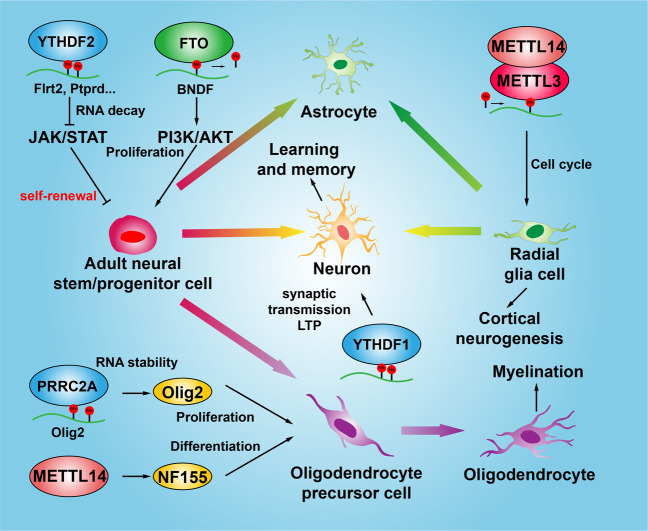
m6A RNA methylation regulates central nervous system development. Neural stem/progenitor cells have the potential to self-renewal, which can differentiate to produce various types of nervous cells, including neurons, astrocytes, and oligodendrocytes. YTHDF2 and FTO promote the self-renewal and proliferation of NSCs by regulating the JAK/STAT and PI3K/AKT signaling pathways. METTL14 and METTL3 promote cortical biogenesis by accelerating the cell cycle of radial glial cells. PRRC2A and METTL14 promote the proliferation and differentiation of oligodendrocyte precursor cells and the myelination process by promoting the expression of Olig2 and NF155, respectively. YTHDF1 regulates learning and memory by promoting synaptic transmission and transcription of LTP-related target genes in neurons
m6A RNA methylation regulates reproductive system development
A number of studies have shown that m6A modification plays pivotal roles in both oocyte maturation and spermatogenesis. Sexual reproduction starts with gametogenesis in both parents through meiosis, and fertilization then occurs with the merging of oocyte and sperm, which then initiates the developmental program of the offspring. Ivanova et al. reported that YTHDF2 is required for oocyte maturation and early zygotic development138. Qi et al. sequenced the N6-methyladenosine (m6A) modified mRNAs in various stages of oocytes in Xenopus laevis, and identified bunch of mRNAs with m6A peaks exhibiting lower protein levels than those of the hypomethylated mRNAs. The hypomethylated mRNAs were mainly involved in regulating the cell cycle and translation pathways, whereas the highly m6A-modified mRNAs were mainly associated with the protein phosphorylation, both of which are important for controlling oocyte meiotic maturation and early embryo development139. Using zebrafish as model, it has been demonstrated that METTL3 mutation disrupts gamete maturation and reduces fertility through decreasing overall m6A levels and expressions of critical genes required for sex hormone synthesis and gonadotropin signaling140. The nuclear m6A reader YTHDC1 has been indicated to interact with the pre-mRNA 3’ end processing factors CPSF6, SRSF3, and SRSF7, which regulates the processing of pre-mRNA transcripts in the oocyte nucleus during fetal development141.
A recent study showed that m6A determines cell fate to specify the earliest hematopoietic stem cells during the endothelial-to-hematopoietic transition (EHT) in zebrafish, and YTHDF2 knockdown decelerates the decay of mA-modified maternal mRNAs and impedes zygotic genome activation [66]. In addition, depletion of YTHDF2 in mice is partially permissive, which results in the failure during oocyte maturation leading to female-specific infertility138. One study indicated that Drosophila Dmime4, the homolog of the Inducer of MEiosis 4 (IME4) gene catalyzing m6A modification in Saccharomyces cerevisiae, was highly expressed in ovaries and testes, which regulates the m6A installation of Notch transcript during follicle development142. The m6A eraser ALKBH5 has been identified to be highly expressed in male mice testes, ablation of which shows increased m6A in mRNAs mainly involved in p53 functional network leading to testicular atrophy, remarkably reduced rate of breeding and decreased fertility in Mice30. Consistently, a negative association between m6A modification and autophagy in Leydig cells (LCs) during testosterone synthesis was discovered, and a gradual decrease of METTL14 and an increase of ALKBH5 were detected in LCs during their differentiation by promoting translation of PPM1A but decreasing CAMKK2 RNA stability36. Furthermore, deficiency of the m6A reader protein YTHDC2 in mice has been shown to result in significant smaller testes and ovaries compared to control littermates62 (Fig. (Fig.44).
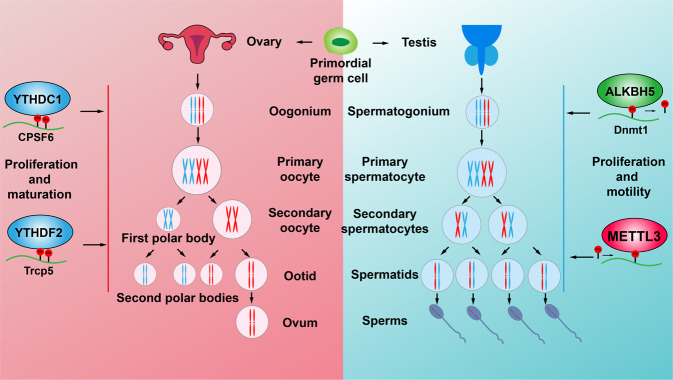
m6A RNA methylation regulates the reproductive system development. Primordial germ cells proliferate and differentiate into spermatocytes and oogonium in embryonic testes and ovaries, respectively. Spermatocytes and oogonium then undergo meiosis to become mature sperms and ovum, respectively. METTL3 and ALKBH5 regulate the levels of overall m6A mRNA methylation to promote the proliferation and motility of sperm cells. YTHDC1 and YTHDF1 regulate the maturation and translation of CPSF6 and Trcp5 transcripts, respectively, to promote the proliferation and maturation of oocytes
m6A RNA methylation regulates other developmental processes
m6A methylation has been demonstrated to regulate various aspects of mRNA metabolism during adipose tissue expansion143–145. For examples, FTO has been demonstrated to associate with obesity and inhibit mitochondrial functions in adipocyte precursors via ARID5B-mediated repression of IRX3 (iroquois-related homeobox 3) and IRX5, and silencing FTO resulted in high m6A methylation on ATG5 and ATG7 mRNAs recognized by YTHDF2, leading to mRNA degradation and reduced autophagy and adipogenesis146,147. Consistently, FTO was uncovered to regulate exonic splicing of RUNX1T1 by regulating m6A modifications around splice sites, and the expression of cell-cycle regulators including CCNA2 and CDK2, and thereby modulates adipogenesis52,148. Furthermore, YTHDF1 was recently revealed to specifically recognize m6A-modified transcript of MTCH2 (mitochondrial carrier homology 2) in Jinhua pigs (obese-type breed with higher levels of intramuscular fat), which promotes MTCH2 mRNA translation and facilitates adipogenesis in intramuscular preadipocytes149. It has also been shown that fat-specific knockout of human antigen R (HuR), represses myogenesis program in brown fat by modulating the stability of Insig1, a negative regulator during adipogenesis150. Yao et al. showed that deletion of METTL3 in porcine bone marrow stem cells (BMSCs) activated JAK1/STAT5/C/EBPβ signaling pathway, and then promoted adipogenesis in an m6A-dependent manner151. Cen et al. also found that TRAF4 bound to PKM2 and negatively regulated mesenchymal stem cells (MSCs) adipogenesis by activating β-catenin signaling, while the reduced expression of TRAF4 during adipogenesis was controlled by ALKBH5152.
One study by Yuan’s group showed that deletion of METTL3 reduced the translational efficiency of MSCs lineage allocator Pth1r (parathyroid hormone receptor-1), and disrupted the PTH (parathyroid hormone)-induced osteogenic and adipogenic responses, leading to impaired bone formation153. Another study showed that METTL3 cooperates with ALKBH5 to regulate osteogenic differentiation, and knockdown of METTL3 reduces MYD88 expression, a critical upstream activator of NF-κB signaling, and therefore increases osteogenic progression154. In line with the above two findings, the inhibitory roles of METTL3 in osteogenesis by regulating RUNX2 or phosphatidylinositol 3-kinase/AKT (PI3K-Akt) signaling pathways, have also been documented by other groups155,156. In addition, the dynamic patterns of m6A methylation during liver development have been revealed by profiling transcriptome-wide m6A modification in porcine liver at three developmental stages: newborn (0 day), suckling (21 days) and adult (2 years)157.
Pivotal roles of m6A RNA methylation in cancer
Besides the above findings that m6A RNA methylation is critical for normal hematopoietic, central nervous and reproductive systems development, aberrant m6A modification has also been indicated to be associated with various types of human cancers65,158–164. Here, we only focus on m6A RNA methylation in human cancers related to hematopoietic, central nervous, and reproductive systems (Table (Table33).
Table 3
The functional roles of RNA m6A modification complex in various types of human cancers
Cancer type | m6A regulator | Cell lines | Roles | Functions | Mechanism | References |
---|---|---|---|---|---|---|
AML | METTL3 | CB-CD34![]() ![]() | Oncogene | ↑Proliferation, ↓differentiation | ↑METTL3/↑c-MYC/BCL2 | 123 |
METTL14 | HPC, LSK | Oncogene | ↑Proliferation, ↓differentiation | SPI-METTL14↑/↑MYB/MYC | 173 | |
FTO | NK-AMLs | Oncogene | ↑Proliferation | ↑FTO/↑ASB2 / RARA | 174 | |
FTO | NOMO-1, MA9.3ITD | Oncogene | ↑Proliferation | ↑FTO/↑MYC/CEBPA | 175 | |
ALKBH5 | MMC6 | Oncogene | ↑Self-renewal | ↑ALKBH5 /↑TACC3 | 177 | |
ALKBH5 | MOMO1 | Oncogene | ↑Self-renewal | ↑KDM4C/↑ALKBH5↑/↑AXL | 178 | |
YTHDF2 | CNI, CNG | Oncogene | ↓Apoptosis | ↑YTHDF2/↑Tnfrsf2 | 179 | |
IGF1BP1 | SKNO1, TANOUE | Oncogene | ↓Differentiation, ↓ cell death | ↑IGF2BP1 /↑HOXB4 / MYB | 180 | |
GBM | METTL3 | PBT003, PBT707 | Suppressor | ↓Growth, ↓self-renewal | ↑METTL3 /↑ADAM19 | 184 |
METTL3 | GSC17 | Oncogene | ↑Self-renewal, ↑proliferation | ↑METTL3/↑SRSF↑/↑BCL-X/ NCOR2 | 186 | |
METTL3 | U251, U87 | Oncogene | ↑Self-renewal, ↑DNA repair | ↑METTL3/↑SOX2 | 234 | |
ALKBH5 | GSC11, GSC23 | Oncogene | ↑Proliferation | ↑FOXM1-AS-AKBH5/↑FOXM1 | 185 | |
hnRNPA1 | U251, U87 | Oncogene | ↑Proliferation | ↑hnRNPA1/↑Myc | 188 | |
HnRNPA2 | U251, A172 | Oncogene | ↑Proliferation, ↑migration, | ↑HnRNPA2/↑p-STAT3 and MMP-2 | 189 | |
IGF2BP2 | GSC88 | Oncogene | ↑Self-renewal, ↑proliferation | ↑HIF1A-AS2-IGF2BP2/↑ HMGA1 | 196 | |
YTHDF2 | GSC4121 | Oncogene | ↑Self-renewal,↑proliferation | ↑YTHDF2/↑ MYC/IGFBP3 | 257 | |
BC | METTL3 | T24, EJ | Oncogene | ↑Proliferation | ↑METTL3/↑pri-miR221/222↓PTEN | 198 |
METTL3 | BCa cells | Oncogene | ↑Proliferation | ↑METTL3/↑AFF4/NF-κB/MYC | 199 | |
METTL3 | T24,UMUC3 | Oncogene | ↑Proliferation, ↑metastasis | ↑METTL3/YTHDF2/↑SETD7/KLF4 | 200 | |
METTL3 | SW780, T24, | Oncogene | ↑Proliferation,↑malignant t | ↑METTL3/↑CDCP1 | 201 | |
METTL14 | T24,UMUC3 | Suppressor | ↓Proliferation, ↓self-renewal, | ↓METTL14/↑Notch1 | 202 | |
OVC | ALKBH5 | HEY, HO8910 | Oncogene | ↑Proliferation, ↓apoptosis | ↑TLR4/↑ALKBH5/↑NANOG | 205 |
ALKBH5 | SKOV3,COC1 | Oncogene | ↑Proliferation, ↓autophagy | ↑ALKBH5/↑EGFR- AKT-mTOR | 204 | |
YTHDF1 | A2780, SKOV3 | Oncogene | ↑Proliferation, ↑migration, | ↑YTHDF1/↑EIF3C | 38 | |
HnRNPA2 | A2780 | Oncogene | ↑Poliferation, ↑migration, | ↑hnRNPA2B1/↑ Lin28B | 206 | |
IGF2BP1 | ES-2 | Oncogene | ↑Growth, ↑ invasion | ↑SRF/IGF2BP1/↑ PDLIM7 / FOXK1 | 207 | |
CVC | FTO | SiHa, c-33a | Oncogene | ↑Chemo-radiotherapy resistance | ↑FTO /↑β-catenin | 210 |
PC | METTL3 | PC-3 | Oncogene | ↑Proliferation, ↑migration, | ↑METTL3/↑ LEF1 | 211 |
m6A RNA methylation in acute myelocytic leukemia (AML)
AML is a malignant blood cell cancer of the immature myeloid hematopoietic cells in the bone marrow (BM), and a highly heterogeneous human disease with rising mortality165. More recently, numerous studies have focused on studying m6A RNA methylation in AM, as many factors were found to be involved in AML before uncovering their functional roles during m6A modification. For example, WTAP was initially identified as Wilms’ tumor gene (WT1) interactor, which is highly expressed in AML and associated with poor prognosis166,167. In line with its role in splicing regulation, WTAP was then demonstrated to form a complex with METTL3 and METTL14 proteins responsible for m6A modification22,168. Another example is RBM15, found to be highly expressed in hematopoietic system, its knockdown inhibited AML cell differentiation and induced cellular apoptosis, possibly through reducing Notch signaling169,170. Later on, Notch signaling pathway was uncovered as relevant m6A targets modified by RBM15 in AML171. Vu et al. provided evidence showing that METTL3 was highly expressed in AML, which elevates the installed m6A level in BCL2 and PTEN transcripts with, increased translation efficiency, leading to activation of AKT signaling to modulate the cell differentiation and self-renewal of acute myeloid leukemia cells172. In particular, METTL3 knockdown in MOLM-13 AML cell lines resulted in an m6A-dependent reduction of c-MYC, BCL2, and PTEN mRNA translation. c-MYC is a well-known oncogene in leukemia, whereas BCL2 and PTEN are negative regulators of PI3K/AKT pathway. Interestingly, forced expression of a non-functional METTL3 was also able to activate PI3K/AKT pathway, indicating that additional mechanism might be involved123.
Another recent finding showed that METTL14 plays an oncogenic role through elevating the expression of its mRNA targets including MYB and MYC in AML, while MEETL14 is negatively regulated by SPI1173. By contrast, with the m6A writers in AML, high-level expression of the eraser FTO demethylase and its oncogenic effect has also been demonstrated in AML. It was suggested that overexpression of FTO promotes cell proliferation and viability, while decreasing apoptosis and the global mRNA m6A level through reducing ASB2 and RARA expression174. Chen’s group found that R-2-hydroxyglutarate (R-2HG), inhibits FTO activity and increases global m6A RNA methylation levels in R-2HG-sensitive AML cells, which reduces the stability of MYC/CEBPA transcripts and related activities of relevant signaling pathways175. A study by Liu’s group showed that tyrosine kinase inhibitor (TKI) therapy resistant phenotypes depend on FTO overexpression and in turn m6A reduction in leukemia cells176. Interestingly, ALKBH5 was also found to be aberrantly overexpressed in AML, whose high expression correlates with poor prognosis in AML patients. A mechanistic study demonstrated that ALKBH5 is essential for the self-renewal of leukemia stem or initiating cells but not for normal hematopoietic, through post-transcriptional regulation of its target TACC3177. In addition, a recent study identified that KDM4C regulates ALKBH5 expression by increasing chromatin accessibility of ALKBH5 locus, and in turn promoting recruitment of MYB and Pol II. Furthermore, ALKBH5 regulates the stability of receptor tyrosine kinase AXL transcript in an m6A-dependent manner, indicating that ALKBH5 is involved in another signaling axis in AML178. Paris et al. found that the mRNA m6A reader YTHDF2 is overexpressed in AML, its decreased the clonogenic potential of AML cells and enhanced hematopoietic stem cells (HSC) activity by targeting to tumor necrosis factor receptor Tnfrsf2, which contributes to the overall integrity of LSC function179. Another mRNA m6A reader IGF2BP1 was found to be highly expressed in AML cells, and its decreased the proliferation and tumorigenic potential of leukemia cells through regulating the expression of the aldehyde dehydrogenase (ALDH1A1)180 (Fig. (Fig.55).
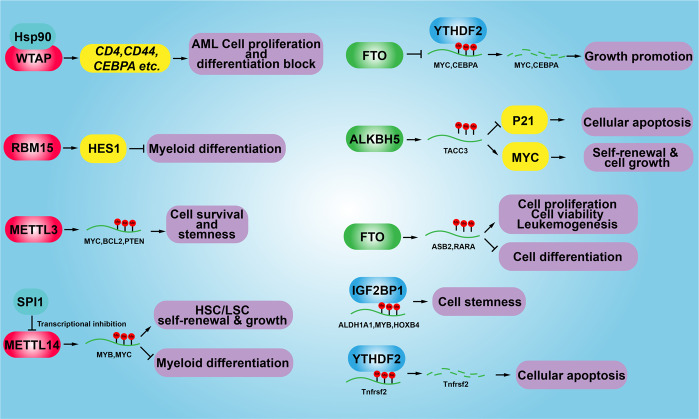
The functional role of m6A modification in human acute myelocytic leukemia (AML). During the development of AML, aberrant methylation or demethylation of the corresponding cancer-related genes contribute differentially during AML progression, including the cell proliferation, cell differentiation, cancer stem cell self-renewal and cellular apoptosis. HSC hematopoietic stem cells, LSC leukemia stem cells
m6A RNA methylation in brain tumor
Recently, increased evidence has demonstrated that writers, erasers, and readers of m6A RNA modification are associated with various types of human cancer, contributing to the self-renewal of cancer stem cell, promotion of cancer cell proliferation, and resistance to radio- or chemotherapy181. Gliomas are the common malignant primary brain tumors, and the standard therapy includes surgery followed by concurrent radiotherapy with temozolomide chemotherapy182. One bioinformatics study using The Chinese Glioma Genome Atlas (CGGA) and The Cancer Genome Atlas (TCGA) datasets showed that METTL3, METTL14, WTAP, RBM15, RBM15B, YTHDC2, YTHDF1, YTHDF2, YTHDF3, hnRNPA2B1, and hnRNPC are differentially expressed in gliomas accompanied with increased WHO grade, indicating that m6A RNA methylation regulators play important role in glioma malignancy183. For example, METTL3 or METTL14 knockdown robustly promotes glioma stem cell (GSC) self-renewal and tumorigenesis through increasing expression of stemness marker genes (e.g., ADAM19), while overexpression of METTL3 or inhibition of FTO inhibits GSC self-renewal and grafted tumor formation in vivo184. Consistently, m6A demethylase ALKBH5 has been identified to be highly expressed in GSC, the silencing of which suppressed the cell proliferation of patient-derived GSC by increasing FOXM1 expression resulted from reduced m6A methylation levels and increased HuR binding in FOXM1 transcript185. However, a controversial study showed that elevated expression of METTL3 is associated with the clinical aggressiveness of malignant gliomas, METTL3 knockdown or overexpression of dominant-negative mutant METTL3 suppressed the growth and self-renewal of GSC. A mechanistic study showed that METTL3 depletion decreased m6A modification levels of serine- and arginine-rich splicing factors (SRSF), leading to YTHDC1-dependent nonsense mediated mRNA decay (NMD) of SRSF transcripts186.
WTAP, identified as a nuclear protein associating with the regulation of cell proliferation and apoptosis, was recently uncovered to be overexpressed in GBM, ablation of which reduces cancerous cell migration and invasion possibly by regulating the EGFR activity187. As a nuclear localized m6A reader protein, hnRNPA1 is upregulated by EGFRvIII, leading to increased glycolytic gene expression and shorter survival time in GBM. More evidence demonstrated that hnRNPA1 promotes splicing of Max transcript and then generating Delta Max, which enhances Myc-dependent cell transformation188. Similarly, hnRNPA2 was uncovered to be highly expressed in gliomas, which is associated with advanced glioma grades. Knockdown of hnRNPA2 can reduce cancerous cell viability, migration, invasion, and chemoresistance for TMZ, by reducing the expressions of phospho-STAT3 and MMP-2, which have been considered as oncogenic drivers in gliomas189. Another study showed that hnRNPA2 high expression causes PKM2 accumulation, suggesting that hnRNPA2 is required for cell proliferation and GBM progression190. Insulin-like growth factor 2 mRNA-binding protein 2 (IG2BP2) was recently found to support GSC and neural stem cell specification by binding to let-7 miRNA recognition elements (MREs) and preventing let-7 targeted gene silencing191. Consistent with this observation, another study demonstrated that IGF2BP2 binds to several mRNAs that encode mitochondrial respiratory chain complex subunits, such as complex I (NADH: ubiquinone oxidoreductase) factors, to regulate oxidative phosphorylation (OXPHOS), leading to increased self-renewal of GSC and tumor initiation by192. Forced expression of miR-873 in GBM cancerous cell lines dramatically reduces the cell proliferation, migration, and invasion by decreasing IGF2BP1 expression193. Furthermore, miRNA138 was identified to be downregulated in low grade gliomas (LGG), which is associated with worse clinical outcome. Ectopic expression of miR-138 suppresses cell proliferation, invasion, and xenograft tumor formation, by directly repressing IGF2BP2 expression194. A similar result found that miRNA129-1 acts as a tumor suppressor and induces cell-cycle arrest of GBM cells through targeting IGF2BP3 and MAPK1195. Except for miRNAs, long no-coding RNA HIF1A-AS2 was uncovered to be upregulated in mesenchymal GSC, whose deregulation affects GSC growth and self-renewal by interacting with IGF2BP2 to maintain the expression of HMGA1196. Circular RNA (circRNA) circHIPK3 was reported to be upregulated in gliomas, which is associated with poor prognosis. circHIPK3 promotes glioma cell proliferation and invasion via interacting with miR-654 leading to the stabilization of IGF2BP3197 (Fig. (Fig.66).
m6A RNA methylation in reproductive system related cancers
In bladder cancer, METTL3 binds to DGCR8 and positively accelerates pri-miR221/222 maturation process, which results in the reduction of PTEN and ultimately increased cell proliferation198. Consistent findings demonstrated that METTL3 is significantly upregulated in bladder cancer, knockdown of which dramatically repressed cancerous cell proliferation, invasion, and xenograft tumor formation via AFF4/NF-kB/Myc axis signaling pathway199. Interestingly, a recent study showed that METTL3 recruits YTHDF2 to degrade the m6A-modified transcripts of the tumor suppressors including SETD7 and KLF4, contributing to the progression of bladder cancer200. Furthermore, new evidence indicated that METTL3 cooperates with YTHDF1 to promote the translation of oncogene CDCP1 in bladder cancer201. However, METTL14 has been identified to be decreased in bladder cancer and related tumor initiating cells (TICs), knockdown of which promotes the cell proliferation, self-renewal, metastasis and tumor initiating capacity through reducing the stability of m6A-modified Notch1 mRNA202.
Decreased mRNA and protein expressions of FTO results in an increase of the overall m6A levels in mRNA leading to premature ovarian insufficiency203. ALKBH5 is elevated in epithelial ovarian cancer, silencing of ALKBH5 enhances the autophagy signaling and inhibits the proliferation and invasion abilities of ovarian cancer cells by reducing EGFR/PI3K/AKT signaling activity through physically interacting with HuR204. Additional evidence showed that ALKBH5 was highly expressed in ovarian cancer tissue, but decreased in cancerous cell lines, whose expression pattern is consistent with Toll-like receptor (TLR4). However, when ovarian cancer cells were co-cultured with M2 macrophages, the expressions of ALKBH5 and TLR4 were both increased. TLR4 was then uncovered to increase ALKBH5 expression via activating NF-kB signaling pathway mediated by m6A modification205. Furthermore, YTHDF1 and hnRNPA2 promote ovarian cancer progression through elevating EIF3C and Lin 28B expression, respectively38,206. In addition, IGF2BP1 was reported to impair the miRNA-directed decay of the SRF mRNA and then promote the expression of SRF in a m6A-dependent manner, sustaining the expression of PDLIM7 and FOXK1, which promotes tumor cell growth and cell invasion of ovarian cancer207. IGF2BP3, as another RNA-binding protein modulating gene expression by post-transcriptional action, was found to be overexpressed in Ovarian Clear Cell Carcinoma (OCCC), which elevates the proliferation and tumorigenicity of OCCC208. The reduced m6A level has been identified in the cervical cancer comparing with the adjacent normal tissue, and increased FTO expression in cervical squamous cell carcinoma (CSCC) tissues can enhance the chemo-radiotherapy resistance of cancer cells by targeting β-catenin through mRNA demethylation209,210. While, METTL3 has been found to be upregulated in prostate cancer, and METTL3 increases the cell proliferation and migration of prostate cancer cells by activating expression of LEF1 or Myc in an m6A methylation dependent manner211,212 (Fig. (Fig.77).

The functional role of m6A mRNA modification in reproductive system related cancers. Aberrant m6A methylation or demethylation of the corresponding cancer-related genes plays different roles in bladder cancer and ovarian cancer. The cell proliferation, cell metastasis, cancer stem cell maintenance, cellular apoptosis, and cell invasion were regulated by different m6A modifiers. BC bladder cancer, OVC ovarian cancer
m6A RNA methylation in other human diseases
Considering the pivotal roles of m6A modification on gene expression, m6A has been increasingly implicated in other human diseases, including psychiatric disorders, metabolic syndrome, and cardiovascular diseases. For example, m6A modification was documented to be impaired in major depressive disorder (MDD) patients, and depletion of METTL3 or FTO in adult neurons has been shown to alter the m6A epitranscriptome and increase fear memory213. YTHDC2 has recently been identified as one of the autism spectrum disorder (ASD) risk gene by comparing ASD family with healthy control group obtained from East Asian populations214, and polymorphisms in ZC3H13 have also been shown to associate with schizophrenia215. Consistently, the overall m6A level was elevated in the cortex and the hippocampus of APP/PS1 (Alzheimer’s disease) mice compared to C57BL/6 control mice, and FTO was found to interact with APOE, which is associated with Alzheimer’s disease risk in a prospective cohort study216,217. In addition, circSTAG1 was recently identified to be decreased in the chronic unpredictable stress-treated mouse hippocampus and in peripheral blood of patients with major depressive disorder, and forced expression of circSTAG1 can attenuate astrocyte dysfunction and depressive-like behaviors in a ALKBH5/FAAH axis dependent manner218.
One study has identified FTO as one of the type 2 diabetes susceptibility genes regulating the adipogenesis51,52. Knockdown of Zinc finger protein 217 (ZFP217) was shown to increase expression of METTL3, which led to YTHDF2-mediated decay of cyclin D1 transcript and reduced mitotic clonal expansion and adipogenesis219. METTL3 was also found to be increased in the liver tissues from patients with type 2 diabetes (T2D), and METTL3 depletion decreased the m6A methylated mRNA of Fatty acid synthase (Fasn), leading to repressed fatty acid metabolism220. Consistently, it has been shown that METTL3 expression and overall mRNA m6A methylation level was upregulated in the livers of mice with high fat diet (HFD)-induced metabolic disorders, while hepatocyte-specific knockout of METTL3 alleviated lipid accumulation and improved insulin sensitivity221. Furthermore, YTHDC2 was shown to be significantly reduced in multiple obese mouse models and nonalcoholic fatty liver disease (NAFLD) patients, and knockdown of YTHDC2 led to excessive triglycerides (TGs) accumulation in hepatocytes, via inhibiting the expressions of lipogenic genes including sterol regulatory element-binding protein 1c, fatty acid synthase and acetyl-CoA carboxylase 1222. Importantly, m6A sequencing in human type 2 diabetes islets revealed multiple hypomethylated transcripts involved in insulin secretion, cell-cycle progression and the Insulin/IGF1 pathway, and METTL14 knockout in mouse β-cell resulted in reduced m6A levels, leading to similar islet phenotype in human T2D and mortality223.
There is emerging evidence showing that m6A modification is closely related to the occurrence and progression of cardiovascular diseases, such as cardiac hypertrophy, heart failure, ischemic heart disease and pulmonary hypertension224,225. For example, the level of m6A methylation was significantly increased on transcripts involved in regulating kinases and intracellular signaling pathways in cardiomyocyte upon hypertrophic stimulation, and overexpression of METTL3 has been shown to promote cardiomyocyte hypertrophy both in vitro and in vivo226. In line with this finding, FTO was uncovered to be decreased in failing mammalian hearts and hypoxic cardiomyocytes, and cardiomyocyte restricted knockout of FTO showed an impaired cardiac function in mice, via selectively demethylating and enhancing the stability of Serca2a mRNA37,227. One more study showed that m6A modification level was increased in hypoxia/reoxygenation (H/R) treated cardiomyocytes and ischemia/reperfusion (I/R)-treated mice heart, and deletion of METTL3 enhanced autophagic flux and inhibited apoptosis in H/R-treated cardiomyocytes dependent on TFEB, a master regulator of lysosomal biogenesis and autophagy228. Furthermore, METTL14 expression was indentified to be upregulated in calcific arteries and primary human artery smooth muscle cell (HASMC) induced by indoxyl sulfate, which selectively methylates vascular osteogenic transcripts229. In addition, total Panax notoginseng saponin (TPNS) was recently found to inhibit the intimal hyperplasia and reverse the reduced m6A quantity in balloon catheter-injured rat carotid artery, by increasing WTAP expression, indicating that WTAP may serve as a novel biomarker and therapeutic target for arterial stenosis in the future230.
Conclusion and future direction
Although numerous findings related to the functional roles of RNA m6A modification have been reported, there are many major knowledge gaps remained to be filled. Whether the nucleic acid position (e.g., 5ʹ-UTR, coding sequence, 3′-UTR, splicing sites) and the level of m6A RNA methylation in RNA transcripts affect the recognition and functions of different m6A reader proteins remains elusive. Various m6A readers exhibit opposite functions. For example, YTHDF2 can promote the degradation of m6A methylated transcripts, whereas IGF2BP proteins can protect their targeted m6A-modified mRNAs from degradation under physiological and pathological conditions64. The competition and cooperation relationship among different reader proteins should be further clarified. Regarding YTH containing family members, YTHDF1 interacts with YTHDF3 in an m6A-independent manner to promote targeted mRNAs, while YTHDF3 interacts with YTHDF2 to promote the degradation of targeted transcripts231,232. A unified functional model for YTHDFs has recently been described233. YTHDF proteins can bind the same m6A-modified mRNAs rather than different mRNAs, and YTHDFs have been found not to be able to induce translation in HeLa cells. Interestingly, in this working model, different YTHDF proteins act redundantly to mediate mRNA degradation and cellular differentiation233. Elevated levels of m6A RNA methylation can promote the proliferation of some types of cancer cells. However, overexpression of METTL3 and METTL14 has also been indicated to suppress cancer cell growth184,185,234. Even in the same type of human cancer, different groups have drawn controversial conclusions, which might be cellular context dependent or differential expression levels of interested genes184,185,234. In addition, recent finding by our group found that, under normoxic conditions, YTHDF1 is highly expressed in non-small cell lung cancer cancerous tissues and cell lines to promote cell proliferation via increasing cell-cycle related factor expression. However, under hypoxic conditions or chemotherapy stressful conditions, YTHDF1 is downregulated which leads to reduced Keap1 mRNA translational efficiency and Nrf2 protein stabilization34.
Recently, Somasundaram’s group found that METTL3-mediated m6A modification in Sox2 is crucial for glioma stem-like cells (GSCs) maintenance and dedifferentiation both in vitro and in vivo234. In line with this finding, other studies also showed an oncogenic role for METTL3142,235–242. However, multiple reports have demonstrated that METTL3 and the associated m6A modification inhibited tumorigenicity of GSCs, while high levels of ALKBH5 was critical for promoting tumorigenicity of GSCs184,185. The phenotypic differences observed could be argued by different reliance on m6A-modified RNAs in different cell state and the dominant RNA species in GBMs. Furthermore, Somasundaram’s group performed a comparison of expression levels of METTL3/METTL14 and FTO/ALKBH5 in multiple GBM transcriptome and pan caner datasets, and found that METTL3 is significantly upregulated in the majority of tumors compared with METTL14, FTO, and ALKBH5. Based on the fact that readers mainly defined the fate of m6A-modified mRNAs, their cell-specific expression levels and mRNA binding affinity in GBM will eventually determine the differential functions of m6A modification. In addition, controversial findings about the role of m6A modification were also detected in mouse embryonic development, which could be explained by using naïve ESCs and primed epiblast stem cells (EpiSC) for functional assays39,43,243,244. m6A modification preserves the stability of EpiSC, while promotes naïve ESCs to undergo epiblast transition by reducing expressions of stem marker genes245. Therefore, further experiments are required for explaining the above discrepancies by performing context and compartment dependent functional studies of m6A modification. The antibody-independent methods for detecting individual m6A sites will also benefit our understanding of these conflict findings resulted from the inter-play between m6A modification and reciprocal reader proteins. In addition, numerous studies have been focused on the functional roles of RNA m6A modifiers in AML but not Chronic myeloid leukemia (CML), resulted from the formation of the BCR-ABL1 fusion protein246. The cross-talk between RNA m6A modification and BCR-ABL1 should be further characterized in future.
Considering different cancers with different genetic background, m6A RNA methylation can regulate oncogene expression (e.g., RNA processing, splicing, translocation, degradation), cancer stem/initiating cell pluripotency, cell differentiation, cell proliferation, migration, angiogenesis, and tumor microenvironment to control cancer progression. Therefore, targeting m6A RNA modification factors could provide potential therapeutic target for various human cancers. For example, R-2-hydroxyglutarate (R-2HG), produced by mutant isocitrate dehydrogenase 1/2 enzymes, was recently found to be able to restrain leukemia cell proliferation and induced cell apoptosis by targeting FTO/m6A/MYC/CEBPA signaling247. A non-steroidal anti-inflammatory drug, meclofenamic acid (MA) and N-(5-Chloro-2,4-dihydroxyphenyl)-1-phenylcyclobutanecarboxamide have recently been identified as selective inhibitors for FTO by competing with FTO binding for the m6A containing nucleic acid248,249. Two promising FTO inhibitors named as FB23 and FB23-2 were developed to selectively inhibit FTO’s m6A demethylase activity, which dramatically suppresses the proliferation and promotes apoptosis of AML cells250. Furthermore, two compounds referred as CS1 and CS2 were shown to bind tightly to FTO protein and block its catalytic pocket, thereby exhibited strong antitumor effects in multiple types of cancers251. MO-I-500 was recently identified as a selective inhibitor of FTO, which was shown to repress the proliferation of triple-negative breast cancer cells252. A more recent study validated the therapeutic potential of targeted mRNA demethylation using an engineered dCas13b-ALKBH5 fusion protein253.
Using lymphopaenic mouse adoptive transfer model, m6A mRNA methylation has been identified to control T cell homeostasis by targeting the IL-7/STAT5/SOCS signaling pathways42. Interestingly, METTL3 has also been reported to promote dendritic cell (DC) activation and function through upregulating the expression of costimulatory molecules CD40, CD80, and cytokine IL-12254. Furthermore, a study by He’s group recently showed that durable neoantigen-specific immunity is regulated by mRNA m6A modification in a YTHDF1 dependent manner, and Ythdf1-depletion mice exhibited an elevated antigen-specific CD8+ T cell antitumor response, and an increased therapeutic efficacy of PD-L1 checkpoint blockade255. In addition, FTO level was found to be increased in human melanoma, knockdown of which increases m6A methylation in PD-1, CXCR4, and SOX10, leading to increased RNA decay through the m6A reader YTHDF2. Therefore, FTO depletion sensitizes melanoma cells to interferon gamma (IFNγ) and anti-PD-1 treatment in mice256. These data suggest that targeting m6A mRNA methylation key regulators could promote anti-cancer therapies in the future.
Acknowledgements
This work was supported by the National Key Research and Development Program of China, Stem Cell and Translational Research (to Y.B.C., 2016YFA0100900), and also National Nature Science Foundation of China (U1902216, 81772996, 81672764, 82060515), Yunnan Applied Basic Research Projects (2019FJ009, 202001AS070037, 2019FB106, 2019FB111 2019HB076 and AMHD-2020-1). C.P.Y was also supported by Youth Innovation Promotion Association, CAS; Yunnan Ten Thousand Talents Plan Young & Elite Talents Project. We thank Dr. Jumin Zhou from KIZ, CAS for his instructive comments on the manuscript writing.
Contributor Information
Cuiping Yang, Email: nc.ca.zik.liam@gnaygnipiuc.
Yongbin Chen, Email: nc.ca.zik.liam@nehcby.
References
Articles from Signal Transduction and Targeted Therapy are provided here courtesy of Nature Publishing Group
Full text links
Read article at publisher's site: https://doi.org/10.1038/s41392-020-00450-x
Read article for free, from open access legal sources, via Unpaywall:
https://www.nature.com/articles/s41392-020-00450-x.pdf
Citations & impact
Impact metrics
Citations of article over time
Alternative metrics

Discover the attention surrounding your research
https://www.altmetric.com/details/100577157
Article citations
METTL14-mediated m6A modification upregulates HOXB13 expression to activate NF-κB and exacerbate cervical cancer progression.
Mol Cell Oncol, 11(1):2423986, 11 Nov 2024
Cited by: 0 articles | PMID: 39534063
METTL3 alters capping enzyme expression and its activity on ribosomal proteins.
Sci Rep, 14(1):27720, 12 Nov 2024
Cited by: 0 articles | PMID: 39532922 | PMCID: PMC11557883
m6A-related genes and their role in Parkinson's disease: Insights from machine learning and consensus clustering.
Medicine (Baltimore), 103(45):e40484, 01 Nov 2024
Cited by: 0 articles | PMID: 39533574 | PMCID: PMC11557064
Silencing METTL3 Increases HSP70 Expression and Alleviates Fibrosis in Keratocytes.
Invest Ophthalmol Vis Sci, 65(13):9, 01 Nov 2024
Cited by: 0 articles | PMID: 39504046 | PMCID: PMC11562871
The role of epigenetic methylations in thyroid Cancer.
World J Surg Oncol, 22(1):281, 25 Oct 2024
Cited by: 0 articles | PMID: 39456011 | PMCID: PMC11515417
Review Free full text in Europe PMC
Go to all (654) article citations
Similar Articles
To arrive at the top five similar articles we use a word-weighted algorithm to compare words from the Title and Abstract of each citation.
The potential role of RNA N6-methyladenosine in Cancer progression.
Mol Cancer, 19(1):88, 12 May 2020
Cited by: 469 articles | PMID: 32398132 | PMCID: PMC7216508
Review Free full text in Europe PMC
The role of m6A RNA methylation in human cancer.
Mol Cancer, 18(1):103, 29 May 2019
Cited by: 588 articles | PMID: 31142332 | PMCID: PMC6540575
Review Free full text in Europe PMC
RNA N6-methyladenosine modification in solid tumors: new therapeutic frontiers.
Cancer Gene Ther, 27(9):625-633, 20 Jan 2020
Cited by: 25 articles | PMID: 31956264 | PMCID: PMC8627172
Review Free full text in Europe PMC
Comprehensive Analysis of N6-Methyladenosine (m6A) Writers, Erasers, and Readers in Cervical Cancer.
Int J Mol Sci, 23(13):7165, 28 Jun 2022
Cited by: 8 articles | PMID: 35806168 | PMCID: PMC9266832