Abstract
Free full text

Selective Genome Packaging Mechanisms of Influenza A Viruses
Abstract
The genome of influenza A virus (IAV) comprises eight segmented, single-stranded, negative-sense RNAs. The genome packaging mechanism of IAV was a long-standing enigma, but it is now widely accepted that IAV packages one copy of each of the eight viral RNA (vRNA) segments in a selective manner. Accumulating evidence over the last decade suggests that the eight unique vRNAs are selected via intersegment interactions mediated by their segment-specific genome packaging signals; however, the characteristics of these RNA-based interactions largely remain unknown. This review summarizes our current knowledge of IAV selective genome packaging and the possible mechanisms underlying the selection of the eight unique vRNAs.
Influenza A virus (IAV) is a member of the Orthomyxoviridae, which is a family of enveloped viruses with a segmented, single-stranded, negative-sense RNA genome. Each of the eight viral RNA (vRNA) segments of IAV, which encode distinct viral protein(s) essential for efficient virus replication, associates with multiple viral nucleoproteins (NPs) and a heterotrimeric RNA-dependent RNA polymerase composed of PB2, PB1, and PA to form a ribonucleoprotein (vRNP) complex. The eight vRNPs are independently responsible for the transcription and replication of the vRNA. The segmented nature of the IAV genome is advantageous in that it allows IAV to evolve rapidly through the exchange of the vRNA segments, a process termed reassortment, in which two or more different IAV strains co-infect the same cell to generate genetically different viruses from the parental viruses. IAV is classified into 18 hemagglutinin (HA) subtypes and 11 neuraminidase (NA) subtypes on the basis of their antigenic properties. All subtypes except for the H17N10 and H18N11 subtypes circulate in their natural reservoir of wild aquatic birds (Tong et al. 2012, 2013). Since the twentieth century, we have experienced four pandemics: H1N1 Spanish influenza in 1918, H2N2 Asian influenza in 1957, H3N2 Hong Kong influenza in 1968, and H1N1 pandemic influenza in 2009. Although the origin of the H1N1 Spanish IAV remains controversial (Taubenberger et al. 2005; Worobey et al. 2014), the H2N2 Asian IAV was generated by the introduction of the HA, NA, and PB1 vRNA segments of an H2N2 avian virus into the circulating human H1N1 virus through reassortment (Kawaoka et al. 1989; Kilbourne 2006). Reassortment between the human H2N2 virus and avian H3 viruses led to the generation of the H3N2 Hong Kong IAV, in which HA and PB1 vRNA segments were introduced from the avian H3 viruses into the human H2N2 virus background. Multiple reassortment events among avian, swine, and human IAVs in swine resulted in the generation of the 2009 H1N1 pandemic virus (Garten et al. 2009; Neumann et al. 2009; Smith et al. 2009). Thus, the introduction and exchange of vRNA segments between different IAVs through reassortment plays an important role in the emergence of pandemic strains, which acquire an HA with novel antigenic properties and the ability to transmit efficiently among humans, allowing the rapid evolution of IAVs.
Despite these evolutional advantages, the reassortment event must surmount substantial difficulties in order to produce infectious IAVs with adequate viral fitness (Villa and Lässig 2017). One such difficulty is overcoming RNA-based incompatibility among vRNA segments. Reassortment is tightly associated with the selective genome packaging process in which eight distinct vRNAs are selected from a large pool of viral and host genetic material in the virus-infected cell and are packaged into each virion to be replicated (Hutchinson et al. 2010; Noda and Kawaoka 2010; Gerber et al. 2014). During this process, the eight vRNA segments are thought to interact with each other. Correct intersegment interactions would ensure the integrity of the genome packaging process and produce novel viruses with pandemic potential within a co-infected cell. Although a large body of recent research clearly supports the idea that IAV packages eight distinct vRNAs through their interactions, the underlying mechanism remains largely unknown. In this review, we summarize our current understanding of selective genome packaging and the possible mechanism by which the eight unique vRNAs are selected.
STRUCTURE OF THE IAV vRNP
The eight single-stranded negative-sense vRNA segments of IAV (PB2, PB1, PA, HA, NP, NA, M, and NS) vary in length from 2341 to 890 bases, but share the same structure: a central coding region in the antisense orientation flanked by segment-specific noncoding regions (NCRs) (19–58 nucleotides) and the terminal promotor regions U12 and U13 (12 and 13 nucleotides from the 3′ and 5′ ends, respectively), which are highly conserved among all eight vRNA segments (Fig. 1A). The sequences of the terminal regions containing U12 and U13 are partially complementary to each other (Robertson 1979) and form a corkscrew structure through base-pairing (Fodor et al. 1994; Flick et al. 1996; Tomescu et al. 2014), where the heterotrimeric RNA-dependent RNA polymerase complex is associated (Klumpp et al. 1997). The rest of the single-stranded vRNA is bound by multiple NPs through its phosphate-sugar backbone in a sequence-independent manner (Baudin et al. 1994). In this way, each vRNA forms a twisted rod-like vRNP complex (Fig. 1B), which is about 12 nm in diameter but varies in length from about 30 to 120 nm, which correlates with the nucleotide length of each vRNA (Pons et al. 1969; Compans et al. 1972; Murti et al. 1988; Sugita et al. 2013). Recent cryo-electron microscopy (cryo-EM) has yielded a more detailed structure of the twisted rod-like vRNP, in which a single strand of a multiple NP-vRNA complex is folded back to form a loop at the opposite end to the polymerase-bound end and coils back on itself to form an antiparallel double-stranded helix (Arranz et al. 2012; Moeller et al. 2012).

Structure of influenza A virus (IAV) viral RNA (vRNA) and ribonucleoprotein (vRNP). (A) A protein-coding region in the antisense orientation is flanked by segment-specific noncoding regions (NCRs) and terminal U12 and U13 promoter regions. The bipartite conventional packaging signal, bundling signal, and incorporation signal regions are indicated by arrows. (B) Conventional model of vRNP. Predicted promoter regions, NCRs, packaging signal regions, and central coding regions in the rod-like vRNP are shown. (C) Revised model of vRNP. Secondary and/or tertiary structures composed of nucleoprotein (NP)-free vRNAs protrude from the vRNP at multiple positions.
One of the most important findings regarding the vRNP structure in recent years came from the RNA sequencing technique CLIP (UV-cross-linking and immunoprecipitation). Previously, it had been believed that NPs uniformly bind to the entire length of the single-stranded vRNA, where secondary and tertiary structures of the vRNA are completely melted by the binding of the NPs (Baudin et al. 1994), although there is a report that a bacteriophage PP7 RNA tag inserted into the NA vRNA maintains the hairpin structure in the context of the vRNP (York et al. 2013). Recently, Lakdawala and her colleagues performed a HITS-CLIP (high-throughput sequencing of RNA isolated by CLIP) analysis to determine the NP-binding sites of vRNAs within virion-derived vRNPs (Lee et al. 2017; Le Sage et al. 2018). They found that NPs associate with vRNAs in a nonuniform and nonrandom manner, in which NPs preferentially bind to guanine-rich and uracil-poor regions, and that there are NP-free regions on vRNAs. Similarly, Boon and colleagues performed a PAR-CLIP (photoactivatable ribonucleoside-enhanced CLIP) analysis to assess NP–vRNA interactions in virus-infected cells. They demonstrated that NPs bind to about 12 nucleotides of vRNA with a mean distance of 25 nucleotides between adjacent NP binding sites without apparent sequence specificity (Williams et al. 2018). Interestingly, ~10% of the viral genome comprises low-NP binding regions and the vRNAs of low-NP binding regions are predicted by use of in silico analysis to form secondary and/or tertiary structures. Collectively, these studies led to a revised vRNP model, in which certain vRNA regions are free of NP and form secondary and/or tertiary structures that protrude from the twisted rod-like body of the vRNP (Fig. 1C). According to the vRNP structure revealed by cryo-EM (Arranz et al. 2012; Moeller et al. 2012), vRNA nucleotides located in the spaces between two neighboring NP molecules are “naked” (i.e., not associated with NP) and potentially form secondary and/or tertiary structures on the vRNP. In agreement with this revised vRNP model, several bioinformatics studies have suggested the importance of predicted stem-loop and pseudoknot structures in the HA and NP vRNAs for viral fitness (Gultyaev et al. 2014, 2016). In addition, Kobayashi et al. predicted several stem-loop structures in the M vRNA that are highly conserved in IAVs and they experimentally demonstrated that disruption of one of the stem-loop structures consisting of nucleotide positions 219–240 (throughout, nucleotide numbering refers to positive-sense cRNA) attenuates virus replication (Kobayashi et al. 2016). Taken together, it is now believed that vRNPs display multiple protruding secondary and/or tertiary structures, some of which likely play important roles in virus replication and selective genome packaging as described later.
PACKAGING MODELS OF THE SEGMENTED IAV GENOME
Genome packaging of IAV occurs during the late stage of the infection cycle, when eight-segmented vRNAs are incorporated into progeny virions at the surface of virus-infected cells (Hirst 1962; McGeoch et al. 1976). Historically, two conflicting models had been proposed to explain the mechanism: the random packaging model and the selective packaging model (Hutchinson et al. 2010; Noda and Kawaoka 2010). In the random packaging model, any number and combination of vRNAs are packaged randomly into progeny virions through a genome packaging signal common to all eight vRNAs, which theoretically differentiates between vRNAs and cellular RNAs but not among the eight vRNAs, resulting in the generation of considerable numbers of noninfectious and semi-infectious particles (Enami et al. 1991; Bancroft and Parslow 2002). In contrast, in the selective packaging model, one copy of each of the eight vRNAs is selectively packaged into each progeny virion through distinct packaging signals specific to each vRNA (Duhaut and McCauley 1996; Odagiri and Tashiro 1997). Although a substantial proportion of released virus particles fails to express one or more viral gene product(s) in virus-infected cells (Martin and Heleniust 1991; Brooke et al. 2013), which seemingly supports the random packaging model, these observations do not necessarily refute the selective packaging model (Diefenbacher et al. 2018), because these phenomena can be explained by failures of uncoating or nuclear import of vRNPs, or lethal mutations or deletions in the vRNAs for transcription, replication, and/or viral protein synthesis, or by incomplete genome packaging of less than eight vRNA segments (Nakatsu et al. 2018). Rather, almost all of the evidence obtained over the past 15 years strongly supports the selective packaging model and indicates that the eight distinct vRNAs are selectively packaged into most, but not all, progeny virions.
SEGMENT-SPECIFIC GENOME PACKAGING SIGNALS OF THE IAV GENOME
Although several early studies suggested that the genome packaging process was not purely random (Laver and Downie 1976; Nakajima and Sugiura 1977; Lubeck et al. 1979; Duhaut and McCauley 1996; Odagiri and Tashiro 1997; Duhaut and Dimmock 2000, 2002), the first direct evidence in support of selective genome packaging was established by our group. Using a reverse genetics approach, Fujii et al. systematically searched for the genome packaging signal of the NA vRNA and demonstrated that, in addition to its NCRs (Luytjes et al. 1989; Bancroft and Parslow 2002), about 180 and 160 nucleotides from the 3′ and 5′ ends of the coding region, respectively, are required for efficient and stable packaging of the NA vRNA into virus particles, indicating that the bipartite regions act as the packaging signal of NA vRNA (Fujii et al. 2003). Because the regions contain protein-coding sequences unique to NA vRNA, this finding indicates that NA vRNA is packaged into progeny virions in a selective manner. Subsequently, the genome packaging signals unique to each of the other seven vRNA segments have been identified (Watanabe et al. 2003; Dos Santos Afonso et al. 2005; Fujii et al. 2005; Liang et al. 2005; de Wit et al. 2006; Muramoto et al. 2006; Ozawa et al. 2007, 2009); all of the segment-specific packaging signals are bipartite, being located in both NCRs and adjacent terminal coding regions (within 100–300 nucleotides from each end of the coding region, depending on the vRNA) (Fig. 1A). Collectively, the existence of the segment-specific packaging signal sequences in all eight vRNAs indicates that eight unique vRNAs are selectively packaged into progeny virions. Because there seem to be no boundaries in the packaging signals that separate whether they are functional or nonfunctional, the packaging signals appear to comprise multiple short functional regions, and therefore the minimal packaging signal sequences have not been determined. In line with this assumption, the introduction of synonymous point mutations into the packaging signal region sometimes can cause a significant reduction in the genome packaging efficiency of mutated vRNA, although no significant impact on the genome packaging efficiency may be observed with other synonymous point mutations (Fujii et al. 2005; Gog et al. 2007; Marsh et al. 2007, 2008; Hutchinson et al. 2008, 2009; Liang et al. 2008). Therefore, it is thought that each segment-specific packaging signal is a cluster that comprises multiple, discontinuous, short-nucleotide elements (Gog et al. 2007). This idea is compatible with the revised vRNP model, in which multiple vRNA regions are free of NP and form secondary and/or tertiary structures on the surface of the vRNP; such structured short vRNA regions would cooperatively and redundantly act as packaging signals.
ORGANIZATION OF vRNPs WITHIN THE IAV VIRION
In addition to the reverse genetics studies described above, multicolor single-molecule fluorescence in situ hybridization (FISH) analysis has provided additional evidence in support of selective packaging. Chou et al. (2012) examined colocalization of various combinations of two different vRNAs within individual virus particles and quantitatively showed, by using a photobleaching technique, that most virus particles likely contain one copy of each of the eight different vRNAs. However, it had remained unclear how many vRNPs are exactly packaged into each virion and how they are arranged within a virion. By using thin-section electron microscopy, we revealed that each budding virion packages eight vRNPs arranged in a specific “1 + 7” pattern, in which a central vRNP is surrounded by seven vRNPs (Fig. 2A; Noda et al. 2006). The specific arrangement of the eight vRNPs is commonly observed in virions of various IAV strains (Harris et al. 2006; Noda et al. 2006) and also in influenza B viruses (Nakatsu et al. 2016). These observations provided strong evidence that eight vRNPs are packaged into each virion in a selective manner. Interestingly, we also revealed that a mutant virus that possessed only seven vRNAs and lacked the HA vRNA also packaged eight vRNPs arranged in a specific “1 + 7” arrangement (Noda et al. 2018). This is consistent with the finding that influenza C and D viruses, which naturally possess a seven-segmented RNA genome, package eight vRNPs arranged in the same configuration (Nakatsu et al. 2018) and suggests that assembly of eight vRNPs into the specific arrangement is an important step for the selective genome packaging process. When budding virions are longitudinally sectioned, a set of eight vRNPs arranged in the specific pattern is always found at the top of each budding virion, even in huge filamentous virions of ~1 μm in length (Noda et al. 2006), suggesting that there are as-yet-unidentified viral and/or host factors at the budding tip that are responsible for the incorporation of the set of eight vRNPs (Fig. 2B). Regarding the directionality of the eight vRNPs within the virions, our immunoelectron microscopic analysis using antipolymerase antibodies revealed that some of the eight vRNPs direct their polymerase end to the budding tip, but others direct their loop ends to the budding tip (Sugita et al. 2013), indicating antiparallel alignment of some of the vRNPs (Fig. 2B). Because both of the bipartite packaging signal sequences are located close to the polymerase end of the respective rod-like vRNPs (Fig. 1A), this finding suggests that the respective packaging signals, in the context of the vRNPs, are not always close to each other within a virion (Fig. 2B). It remains unclear which vRNAs direct their polymerase ends to the budding tip and whether the directionality of the eight vRNAs is always the same.
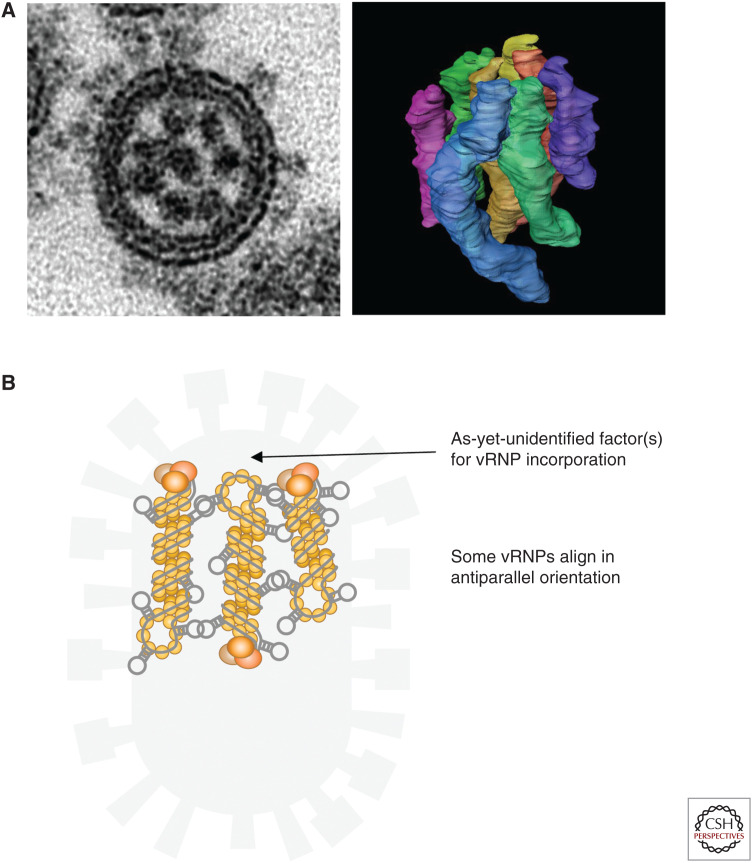
Eight vRNPs within a virion. (A) (Left) Transverse section of a budding virion (left side of A is reprinted from Noda et al. [2006] with permission from the authors); (right) three-dimensional model of eight vRNPs within a virion reconstructed by electron tomography (right side of A is reprinted from Noda et al. [2012] under a Creative Commons Attribution-NonCommercial-Share Alike 3.0 Unported License). Each of the eight vRNPs is colored differently. (B) A single bundle of eight vRNPs arranged in a “1 + 7” pattern, in which some vRNPs align in antiparallel orientation, associates with the tip of the budding virion, even in huge filamentous virions. Note that only three vRNPs are depicted here for clarity.
Three-dimensional (3D) analysis of the eight vRNPs within budding virions by use of electron tomography showed that the eight vRNPs are different in length and that their positions within the specific “1 + 7” arrangement are not purely random, although their exact, assigned positions have not yet been determined because of technical difficulties (Fournier et al. 2012a, 2012b; Noda et al. 2012). Importantly, in our 3D analysis, we found multiple nucleic acid–like string structures connecting neighboring vRNPs; such interactions were observed across the entire length of the rod-like vRNPs (Fig. 3A; Noda et al. 2012). Although the identity of the nucleic acid–like string structures was unclear at that time, we now believe that they may be NP-free, secondary, and/or tertiary structures of vRNA protruding from the surface of the rod-like vRNPs. These structural findings suggest that the eight vRNPs are assembled into the specific “1 + 7” pattern through multiple interactions via NP-free vRNAs. Because the interconnections through the string-like structures are observed at multiple positions spanning the entire length, it is feasible that the interactions do not only occur between the packaging signals. Rather, given that the eight vRNPs align in antiparallel orientation within budding virions (Sugita et al. 2013), it has been suggested that certain packaging signals may interact not only with the packaging signals of other vRNAs but also with secondary or tertiary RNA structures located in the protein-coding regions (Fig. 3B).
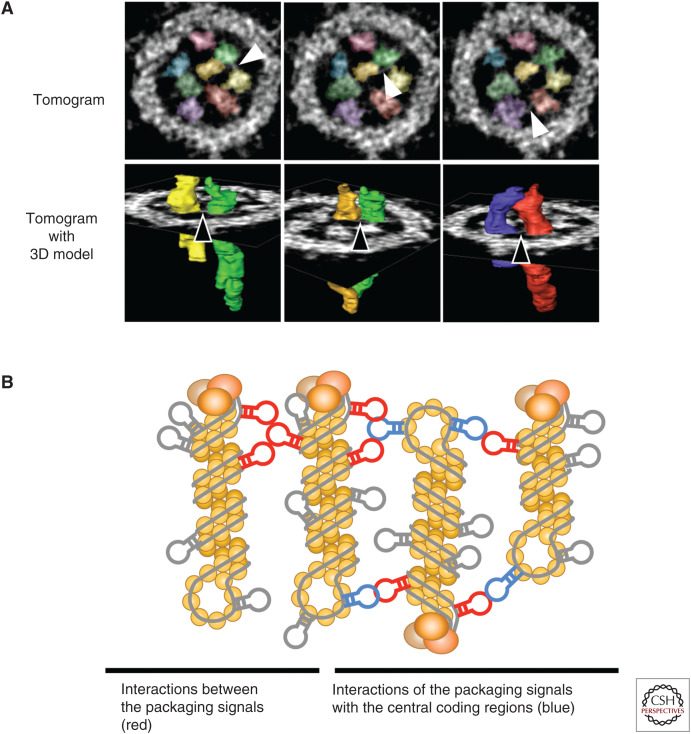
Interactions among vRNPs. (A) Nucleic acid–like string structures connect vRNPs at multiple positions. Note that the string structures are not restricted to the top of the rod-like vRNPs. Arrowheads indicate nucleic acid–like string structures. (B) Intersegment interactions. Interactions between the genome packaging signals (red) as well as interactions of the packaging signals with nonpackaging signals (blue) are indicated. (A, reprinted from Noda et al. [2012] under a Creative Commons Attribution-NonCommercial-Share Alike 3.0 Unported License.)
FUNCTIONAL vRNA–vRNA INTERACTIONS DURING GENOME PACKAGING
An attractive model to explain the mechanisms underlying the selective genome packaging of eight unique vRNAs is that specific interactions take place among the eight distinct vRNAs through their genome packaging signals. The initial evidence suggesting vRNA–vRNA interactions came from our group. Muramoto et al. (2006) searched for the genome packaging signals of the PB2, PB1, and PA vRNAs and showed that those packaging signals are necessary not only for the packaging of the respective residential vRNAs, but that they also affect the packaging efficiencies of the other vRNAs. Subsequently, similar findings were reported for the other vRNAs, in which mutations in the packaging signal of a certain vRNA were shown to affect the packaging efficiency of one or more other vRNAs (Gog et al. 2007; Marsh et al. 2007, 2008; Hutchinson et al. 2008, 2009). It appears that the eight vRNAs are not independently, but rather cooperatively, packaged into virions and interact with each other during the genome packaging process. Importantly, Muramoto et al. (2006) also demonstrated that the trans-acting effect on the packaging efficiency of the other vRNAs is most prominent with PB2 vRNA, indicating that there is a hierarchy among the eight vRNAs for the selective genome packaging. Similarly, Gao et al. (2012) used a different IAV strain to show that all eight vRNAs are not equally important for the packaging of the other vRNAs, and that in addition to the PB2 vRNA, the PA, NP, and M vRNAs play important roles. Thus, selective genome packaging likely involves intersegment associations among the eight unique vRNAs in a hierarchal process, in which certain vRNA(s) (e.g., the PB2 vRNA) may act as a master segment for assembling multiple vRNAs through their interactions.
Our group has also identified important regions in the packaging signal for intersegment association. Goto et al. (2013) showed that the NCRs of a vRNA, comprising the promoter regions and segment-specific NCRs, are sufficient for its efficient packaging into virus particles and that the terminal protein-coding regions of the packaging signal are essential for the packaging of other vRNAs. The finding indicates that the conventional packaging signal, which is composed of the conserved promotor region, segment-specific NCRs, and adjacent terminal protein-coding regions, can be divided into two regions based on their different roles: the NCRs (i.e., the promoter regions and the segment-specific NCRs) of vRNAs serve as the “incorporation signal” that is responsible for the packaging of the residential vRNA, whereas the terminal coding regions serve as the “bundling signal” that ensures the packaging of a complete set of eight vRNAs (Fig. 1A). In this scenario, the bundling signal regions would be important for intersegment associations during selective genome packaging. This is partially consistent with a bioinformatics-based study by Gog et al. (2007), which showed that codons in the terminal protein-coding regions of the vRNAs are more conserved than those in the central protein-coding regions. Intriguingly, our group also showed that the bundling signals could have another role in selective genome packaging. Inagaki et al. (2012) demonstrated that two artificial vRNAs that had identical bundling signals but encoded different reporter proteins competed with each other for packaging into virions, leading to the packaging of a single copy of the vRNA. Such self-repulsion of identical vRNAs through the bundling signals would strengthen the selectivity of a single copy of each of the eight unique vRNAs (Venev and Zeldovich 2013).
Although intersegment associations are predicted to be mediated by the genome packaging signals through base-pairing, NP proteins are also involved in selective genome packaging. Moreira et al. (2016) introduced mutations into certain NP residues and showed that such mutations altered the packaging efficiencies of specific vRNA subsets. In addition, a defect in packaging efficiency could be partially restored by mutations in specific residues of NP (Bolte et al. 2019). These results strongly suggest that intersegment associations are regulated not only by vRNA–vRNA interactions but also by vRNA–NP interactions. The manner of vRNA–NP interaction would affect the formation of stable secondary and/or tertiary structures of vRNA protruding from the vRNP, and such local structural changes caused by mutations in NP as well as in the vRNA would affect the vRNA–vRNA interactions and subsequent packaging of specific vRNAs (Takizawa et al. 2019).
EVIDENCE FOR DIRECT vRNA–vRNA ASSOCIATIONS
Early evidence for direct intersegment associations was obtained by using in vitro electrophoretic mobility shift assays and in vitro–synthesized naked vRNAs. In both human H3N2 and avian H5N2 viruses, all eight vRNAs interact with one or more vRNAs and form single networks of intersegment associations (Fournier et al. 2012a, 2012b; Gavazzi et al. 2013a, 2013b). Similar findings have been reported for human H1N1 virus, in which each vRNA interacts with at least one vRNA through terminal coding regions in many cases (Miyamoto and Noda 2020). Curiously, the networks of intersegment interactions are different among these three strains, and only a few combinations are conserved. Because vRNA–vRNA associations are partially regulated by vRNA–NP interactions (Moreira et al. 2016), such in vitro interaction networks do not necessarily reflect actual networks in the context of vRNPs and could include false-positive associations while missing actual associations because of the in vitro experimental conditions and the absence of NPs and host proteins. However, despite the limited efficacy, such an in vitro study identified a direct interaction between the PB1 and NS vRNAs of an H5N2 virus, which was mediated by stem-loop structures composed of nucleotides 257–277 of the NS vRNA and nucleotides 289–309 of the PB1 vRNA; the study also showed the importance of this interaction for efficient virus replication (Gavazzi et al. 2013b). In addition, a direct interaction between the PB1 and NA vRNAs of a human H3N2 virus has also been shown to play an important role in selective genome packaging, in which preferential co-packaging of the PB1 vRNA with the NA vRNA into progeny virions was shown to be mediated by an RNA-based direct interaction through nucleotides 1776–2070 of the PB1 vRNA (Cobbin et al. 2014; Gilbertson et al. 2016). To date, these two reports are the only experimental demonstrations of direct intersegment interactions being important for co-packaging. However, these regions are located slightly outside the bundling signals (more than 250 nucleotides from the termini of the coding regions). Direct interactions through the bundling signals (or conventional packaging signal) have not yet been experimentally proven to be essential for co-packaging.
Recently, Dadonaite et al. (2019) employed a SPLASH (sequencing of psoralen cross-linked, ligated, and selected hybrids) approach to examine direct vRNA–vRNA interactions in the context of vRNPs in virio. They found that most vRNAs establish multiple and redundant RNA-mediated intersegment interactions at both the terminal and central protein-coding regions. The interaction of the PB1 vRNA with the NA vRNA through nucleotides 1776–2070 (Gilbertson et al. 2016) was detected by using SPLASH, and the association was experimentally shown to be important for efficient co-packaging, confirming the importance of direct vRNA–vRNA interactions for selective genome packaging. However, many of the intersegment interactions detected by use of SPLASH and electrophoretic mobility shift assays have not yet been evaluated in the context of virus replication, and the contributions of the respective vRNA–vRNA interactions to co-packaging remain uncertain. In addition, because of the lack of ultrastructural information, it is not clear how the vRNAs are interconnected in the context of the vRNPs to form complexes of multiple rod-like vRNPs.
CONCLUDING REMARKS
Accumulating evidence indicates that intersegment interactions among the eight unique vRNAs of IAVs are involved in the selective genome packaging process and that these interactions are likely mediated by secondary and/or tertiary structures of NP-free vRNA regions protruding from the vRNPs. In addition to packaging signals (i.e., the incorporation signal and the bundling signal), other regions (i.e., central protein-coding regions) are likely involved in the intersegment interactions. Although many reverse genetics studies have shown the importance of the bundling signals for the co-packaging of multiple vRNAs, no direct interaction sites between two vRNAs have yet been identified in the bundling signals. Accordingly, there is a long way to go before the overall picture of the interactive network is revealed. Further studies are needed to understand the precise mechanisms by which the eight unique vRNPs are specifically interconnected and form the specific “1 + 7” arrangement during selective genome packaging.
ACKNOWLEDGMENTS
We thank Susan Watson for editing the manuscript. This work was supported by Japan Society for the Promotion of Science (JSPS) Grant-in-Aid for Challenging Research (Exploratory) (19K22529), by JSPS Grant-in-Aid for Scientific Research (B) (20H03494), by JSPS Core-to-Core Program A, the Advanced Research Networks, by a Ministry of Education, Culture, Sports, Science and Technology (MEXT) Grant-in-Aid for Scientific Research on Innovative Area (19H04831), by the Japan Agency for Medical Research and Development (AMED) Research Program on Emerging and Re-emerging Infectious Disease grants (19fk0108113, 20fk0108270h0001), by a Grant for Joint Research Projects of the Institute of Medical Science, University of Tokyo, by the Joint Usage/Research Center program of the Institute for Frontier Life and Medical Sciences Kyoto University, by the Daiichi Sankyo Foundation of Life Science, by the Uehara Memorial Foundation, and by the Takeda Science Foundation.
This article has been made freely available online courtesy of TAUNS Laboratories.
Footnotes
Editors: Gabriele Neumann and Yoshihiro Kawaoka
Additional Perspectives on Influenza: The Cutting Edge available at www.perspectivesinmedicine.org
REFERENCES
- Arranz R, Coloma R, Chichon FJ, Conesa JJ, Carrascosa JL, Valpuesta JM, Ortin J, Martin-Benito J. 2012. The structure of native influenza virion ribonucleoproteins. Science 338: 1634–1637. 10.1126/science.1228172 [Abstract] [CrossRef] [Google Scholar]
- Bancroft CT, Parslow TG. 2002. Evidence for segment-nonspecific packaging of the influenza A virus genome. J Virol 76: 7133–7139. 10.1128/JVI.76.14.7133-7139.2002 [Europe PMC free article] [Abstract] [CrossRef] [Google Scholar]
- Baudin F, Bach C, Cusack S, Ruigrok RW. 1994. Structure of influenza virus RNP. I. Influenza virus nucleoprotein melts secondary structure in panhandle RNA and exposes the bases to the solvent. EMBO J 13: 3158–3165. 10.1002/j.1460-2075.1994.tb06614.x [Europe PMC free article] [Abstract] [CrossRef] [Google Scholar]
- Bolte H, Rosu ME, Hagelauer E, García-Sastre A, Schwemmle M. 2019. Packaging of the influenza virus genome is governed by a plastic network of RNA- and nucleoprotein-mediated interactions. J Virol 93: e01861-18. 10.1128/JVI.01861-18 [Europe PMC free article] [Abstract] [CrossRef] [Google Scholar]
- Brooke CB, Ince WL, Wrammert J, Ahmed R, Wilson PC, Bennink JR, Yewdell JW. 2013. Most influenza A virions fail to express at least one essential viral protein. J Virol 87: 3155–3162. 10.1128/JVI.02284-12 [Europe PMC free article] [Abstract] [CrossRef] [Google Scholar]
- Chou YY, Vafabakhsh R, Doğanay S, Gao Q, Ha T, Palese P. 2012. One influenza virus particle packages eight unique viral RNAs as shown by FISH analysis. Proc Natl Acad Sci 109: 9101–9106. 10.1073/pnas.1206069109 [Europe PMC free article] [Abstract] [CrossRef] [Google Scholar]
- Cobbin JC, Ong C, Verity E, Gilbertson BP, Rockman SP, Brown LE. 2014. Influenza virus PB1 and neuraminidase gene segments can cosegregate during vaccine reassortment driven by interactions in the PB1 coding region. J Virol 88: 8971–8980. 10.1128/JVI.01022-14 [Europe PMC free article] [Abstract] [CrossRef] [Google Scholar]
- Compans RW, Content J, Duesberg PH. 1972. Structure of the ribonucleoprotein of influenza virus. J Virol 10: 795–800. 10.1128/JVI.10.4.795-800.1972 [Europe PMC free article] [Abstract] [CrossRef] [Google Scholar]
- Dadonaite B, Gilbertson B, Knight ML, Trifkovic S, Rockman S, Laederach A, Brown LE, Fodor E, Bauer DLV. 2019. The structure of the influenza A virus genome. Nat Microbiol 4: 1781–1789. 10.1038/s41564-019-0513-7.13-7 [Europe PMC free article] [Abstract] [CrossRef] [Google Scholar]
- de Wit E, Spronken MI, Rimmelzwaan GF, Osterhaus AD, Fouchier RA. 2006. Evidence for specific packaging of the influenza A virus genome from conditionally defective virus particles lacking a polymerase gene. Vaccine 24: 6647–6650. 10.1016/j.vaccine.2006.06.001 [Abstract] [CrossRef] [Google Scholar]
- Diefenbacher M, Sun J, Brooke CB. 2018. The parts are greater than the whole: the role of semi-infectious particles in influenza A virus biology. Curr Opin Virol 33: 42–46. 10.1016/j.coviro.2018.07.002 [Europe PMC free article] [Abstract] [CrossRef] [Google Scholar]
- Dos Santos Afonso E, Escriou N, Leclercq I, van der Werf S, Naffakh N. 2005. The generation of recombinant influenza A viruses expressing a PB2 fusion protein requires the conservation of a packaging signal overlapping the coding and noncoding regions at the 5′ end of the PB2 segment. Virology 341: 34–46. 10.1016/j.virol.2005.06.040 [Abstract] [CrossRef] [Google Scholar]
- Duhaut SD, McCauley JW. 1996. Defective RNAs inhibit the assembly of influenza virus genome segments in a segment-specific manner. Virology 216: 326–337. 10.1006/viro.1996.0068 [Abstract] [CrossRef] [Google Scholar]
- Duhaut S, Dimmock NJ. 2000. Approximately 150 nucleotides from the 5′ end of an influenza A segment 1 defective virion RNA are needed for genome stability during passage of defective virus in infected cells. Virology 275: 278–285. 10.1006/viro.2000.0502 [Abstract] [CrossRef] [Google Scholar]
- Duhaut SD, Dimmock NJ. 2002. Defective segment 1 RNAs that interfere with production of infectious influenza A virus require at least 150 nucleotides of 5′ sequence: evidence from a plasmid-driven system. J Gen Virol 83: 403–411. 10.1099/0022-1317-83-2-403 [Abstract] [CrossRef] [Google Scholar]
- Enami M, Sharma G, Benham C, Palese P. 1991. An influenza virus containing nine different RNA segments. Virology 185: 291–298. 10.1016/0042-6822(91)90776-8 [Abstract] [CrossRef] [Google Scholar]
- Flick R, Neumann G, Hoffmann E, Neumeier E, Hobom G. 1996. Promoter elements in the influenza vRNA terminal structure. RNA (New York, NY) 2: 1046–1057. [Europe PMC free article] [Abstract] [Google Scholar]
- Fodor E, Pritlove DC, Brownlee GG. 1994. The influenza virus panhandle is involved in the initiation of transcription. J Virol 68: 4092–4096. 10.1128/JVI.68.6.4092-4096.1994 [Europe PMC free article] [Abstract] [CrossRef] [Google Scholar]
- Fournier E, Moules V, Essere B, Paillart JC, Sirbat JD, Cavalier A, Rolland JP, Thomas D, Lina B, Isel C, et al. 2012a. Interaction network linking the human H3N2 influenza A virus genomic RNA segments. Vaccine 30: 7359–7367. 10.1016/j.vaccine.2012.09.079 [Abstract] [CrossRef] [Google Scholar]
- Fournier E, Moules V, Essere B, Paillart JC, Sirbat JD, Isel C, Cavalier A, Rolland JP, Thomas D, Lina B, et al. 2012b. A supramolecular assembly formed by influenza A virus genomic RNA segments. Nucleic Acids Res 40: 2197–2209. 10.1093/nar/gkr985 [Europe PMC free article] [Abstract] [CrossRef] [Google Scholar]
- Fujii Y, Goto H, Watanabe T, Yoshida T, Kawaoka Y. 2003. Selective incorporation of influenza virus RNA segments into virions. Proc Natl Acad Sci 100: 2002–2007. 10.1073/pnas.0437772100 [Europe PMC free article] [Abstract] [CrossRef] [Google Scholar]
- Fujii K, Fujii Y, Noda T, Muramoto Y, Watanabe T, Takada A, Goto H, Horimoto T, Kawaoka Y. 2005. Importance of both the coding and the segment-specific noncoding regions of the influenza A virus NS segment for its efficient incorporation into virions. J Virol 79: 3766–3774. 10.1128/JVI.79.6.3766-3774.2005 [Europe PMC free article] [Abstract] [CrossRef] [Google Scholar]
- Gao Q, Chou YY, Doganay S, Vafabakhsh R, Ha T, Palese P. 2012. The influenza A virus PB2, PA, NP, and M segments play a pivotal role during genome packaging. J Virol 86: 7043–7051. 10.1128/JVI.00662-12 [Europe PMC free article] [Abstract] [CrossRef] [Google Scholar]
- Garten RJ, Davis CT, Russell CA, Shu B, Lindstrom S, Balish A, Sessions WM, Xu X, Skepner E, Deyde V, et al. 2009. Antigenic and genetic characteristics of swine-origin 2009 A(H1N1) influenza viruses circulating in humans. Science 325: 197–201. 10.1126/science.1176225 [Europe PMC free article] [Abstract] [CrossRef] [Google Scholar]
- Gavazzi C, Isel C, Fournier E, Moules V, Cavalier A, Thomas D, Lina B, Marquet R. 2013a. An in vitro network of intermolecular interactions between viral RNA segments of an avian H5N2 influenza A virus: comparison with a human H3N2 virus. Nucleic Acids Res 41: 1241–1254. 10.1093/nar/gks1181 [Europe PMC free article] [Abstract] [CrossRef] [Google Scholar]
- Gavazzi C, Yver M, Isel C, Smyth RP, Rosa-Calatrava M, Lina B, Moules V, Marquet R. 2013b. A functional sequence-specific interaction between influenza A virus genomic RNA segments. Proc Natl Acad Sci 110: 16604–16609. 10.1073/pnas.1314419110 [Europe PMC free article] [Abstract] [CrossRef] [Google Scholar]
- Gerber M, Isel C, Moules V, Marquet R. 2014. Selective packaging of the influenza A genome and consequences for genetic reassortment. Trends Microbiol 22: 446–455. 10.1016/j.tim.2014.04.001 [Abstract] [CrossRef] [Google Scholar]
- Gilbertson B, Zheng T, Gerber M, Printz-Schweigert A, Ong C, Marquet R, Isel C, Rockman S, Brown L. 2016. Influenza NA and PB1 gene segments interact during the formation of viral progeny: localization of the binding region within the PB1 gene. Viruses 8: 238. 10.3390/v8080238 [Europe PMC free article] [Abstract] [CrossRef] [Google Scholar]
- Gog JR, Afonso Edos S, Dalton RM, Leclercq I, Tiley L, Elton D, von Kirchbach JC, Naffakh N, Escriou N, Digard P. 2007. Codon conservation in the influenza A virus genome defines RNA packaging signals. Nucleic Acids Res 35: 1897–1907. 10.1093/nar/gkm087 [Europe PMC free article] [Abstract] [CrossRef] [Google Scholar]
- Goto H, Muramoto Y, Noda T, Kawaoka Y. 2013. The genome-packaging signal of the influenza A virus genome comprises a genome incorporation signal and a genome-bundling signal. J Virol 87: 11316–11322. 10.1128/JVI.01301-13 [Europe PMC free article] [Abstract] [CrossRef] [Google Scholar]
- Gultyaev AP, Tsyganov-Bodounov A, Spronken MI, van der Kooij S, Fouchier RA, Olsthoorn RC. 2014. RNA structural constraints in the evolution of the influenza A virus genome NP segment. RNA Biol 11: 942–952. 10.4161/rna.29730 [Europe PMC free article] [Abstract] [CrossRef] [Google Scholar]
- Gultyaev AP, Spronken MI, Richard M, Schrauwen EJ, Olsthoorn RC, Fouchier RA. 2016. Subtype-specific structural constraints in the evolution of influenza A virus hemagglutinin genes. Sci Rep 6: 38892. 10.1038/srep38892 [Europe PMC free article] [Abstract] [CrossRef] [Google Scholar]
- Harris A, Cardone G, Winkler DC, Heymann JB, Brecher M, White JM, Steven AC. 2006. Influenza virus pleiomorphy characterized by cryoelectron tomography. Proc Natl Acad Sci 103: 19123–19127. 10.1073/pnas.0607614103 [Europe PMC free article] [Abstract] [CrossRef] [Google Scholar]
- Hirst GK. 1962. Genetic recombination with Newcastle disease virus, polioviruses, and influenza. Cold Spring Harbor Symp Quant Biol 27: 303–309. 10.1101/SQB.1962.027.001.028 [Abstract] [CrossRef] [Google Scholar]
- Hutchinson EC, Curran MD, Read EK, Gog JR, Digard P. 2008. Mutational analysis of cis-acting RNA signals in segment 7 of influenza A virus. J Virol 82: 11869–11879. 10.1128/JVI.01634-08 [Europe PMC free article] [Abstract] [CrossRef] [Google Scholar]
- Hutchinson EC, Wise HM, Kudryavtseva K, Curran MD, Digard P. 2009. Characterisation of influenza A viruses with mutations in segment 5 packaging signals. Vaccine 27: 6270–6275. 10.1016/j.vaccine.2009.05.053 [Europe PMC free article] [Abstract] [CrossRef] [Google Scholar]
- Hutchinson EC, von Kirchbach JC, Gog JR, Digard P. 2010. Genome packaging in influenza A virus. J Gen Virol 91: 313–328. 10.1099/vir.0.017608-0 [Abstract] [CrossRef] [Google Scholar]
- Inagaki A, Goto H, Kakugawa S, Ozawa M, Kawaoka Y. 2012. Competitive incorporation of homologous gene segments of influenza A virus into virions. J Virol 86: 10200–10202. 10.1128/JVI.01204-12 [Europe PMC free article] [Abstract] [CrossRef] [Google Scholar]
- Kawaoka Y, Krauss S, Webster RG. 1989. Avian-to-human transmission of the PB1 gene of influenza A viruses in the 1957 and 1968 pandemics. J Virol 63: 4603–4608. 10.1128/JVI.63.11.4603-4608.1989 [Europe PMC free article] [Abstract] [CrossRef] [Google Scholar]
- Kilbourne ED. 2006. Influenza pandemics of the 20th century. Emerging Infect Dis 12: 9–14. 10.3201/eid1201.051254 [Europe PMC free article] [Abstract] [CrossRef] [Google Scholar]
- Klumpp K, Ruigrok RW, Baudin F. 1997. Roles of the influenza virus polymerase and nucleoprotein in forming a functional RNP structure. EMBO J 16: 1248–1257. 10.1093/emboj/16.6.1248 [Europe PMC free article] [Abstract] [CrossRef] [Google Scholar]
- Kobayashi Y, Dadonaite B, van Doremalen N, Suzuki Y, Barclay WS, Pybus OG. 2016. Computational and molecular analysis of conserved influenza A virus RNA secondary structures involved in infectious virion production. RNA Biol 13: 883–894. 10.1080/15476286.2016.1208331 [Europe PMC free article] [Abstract] [CrossRef] [Google Scholar]
- Laver WG, Downie JC. 1976. Influenza virus recombination. I. Matrix protein markers and segregation during mixed infections. Virology 70: 105–117. 10.1016/0042-6822(76)90240-3 [Abstract] [CrossRef] [Google Scholar]
- Lee N, Le Sage V, Nanni AV, Snyder DJ, Cooper VS, Lakdawala SS. 2017. Genome-wide analysis of influenza viral RNA and nucleoprotein association. Nucleic Acids Res 45: 8968–8977. 10.1093/nar/gkx584 [Europe PMC free article] [Abstract] [CrossRef] [Google Scholar]
- Le Sage V, Nanni AV, Bhagwat AR, Snyder DJ, Cooper VS, Lakdawala SS, Lee N. 2018. Non-uniform and non-random binding of nucleoprotein to influenza A and B viral RNA. Viruses 10: 522. 10.3390/v10100522 [Europe PMC free article] [Abstract] [CrossRef] [Google Scholar]
- Liang Y, Hong Y, Parslow TG. 2005. cis-Acting packaging signals in the influenza virus PB1, PB2, and PA genomic RNA segments. J Virol 79: 10348–10355. 10.1128/JVI.79.16.10348-10355.2005 [Europe PMC free article] [Abstract] [CrossRef] [Google Scholar]
- Liang Y, Huang T, Ly H, Parslow TG, Liang Y. 2008. Mutational analyses of packaging signals in influenza virus PA, PB1, and PB2 genomic RNA segments. J Virol 82: 229–236. 10.1128/JVI.01541-07 [Europe PMC free article] [Abstract] [CrossRef] [Google Scholar]
- Lubeck MD, Palese P, Schulman JL. 1979. Nonrandom association of parental genes in influenza A virus recombinants. Virology 95: 269–274. 10.1016/0042-6822(79)90430-6 [Abstract] [CrossRef] [Google Scholar]
- Luytjes W, Krystal M, Enami M, Parvin JD, Palese P. 1989. Amplification, expression, and packaging of a foreign gene by influenza virus. Cell 59: 1107–1113. 10.1016/0092-8674(89)90766-6 [Abstract] [CrossRef] [Google Scholar]
- Marsh GA, Hatami R, Palese P. 2007. Specific residues of the influenza A virus hemagglutinin viral RNA are important for efficient packaging into budding virions. J Virol 81: 9727–9736. 10.1128/JVI.01144-07 [Europe PMC free article] [Abstract] [CrossRef] [Google Scholar]
- Marsh GA, Rabadán R, Levine AJ, Palese P. 2008. Highly conserved regions of influenza A virus polymerase gene segments are critical for efficient viral RNA packaging. J Virol 82: 2295–2304. 10.1128/JVI.02267-07 [Europe PMC free article] [Abstract] [CrossRef] [Google Scholar]
- Martin K, Heleniust A. 1991. Nuclear transport of influenza virus ribonucleoproteins: the viral matrix protein (M1) promotes export and inhibits import. Cell 67: 117–130. 10.1016/0092-8674(91)90576-K [Abstract] [CrossRef] [Google Scholar]
- McGeoch D, Fellner P, Newton C. 1976. Influenza virus genome consists of eight distinct RNA species. Proc Natl Acad Sci 73: 3045–3049. 10.1073/pnas.73.9.3045 [Europe PMC free article] [Abstract] [CrossRef] [Google Scholar]
- Miyamoto S, Noda T. 2020. In vitro vRNA–vRNA interactions in the H1N1 influenza A virus genome. Microbiol Immunol 64: 202–209. 10.1111/1348-0421.12766 [Abstract] [CrossRef] [Google Scholar]
- Moeller A, Kirchdoerfer RN, Potter CS, Carragher B, Wilson IA. 2012. Organization of the influenza virus replication machinery. Science 338: 1631–1634. 10.1126/science.1227270 [Europe PMC free article] [Abstract] [CrossRef] [Google Scholar]
- Moreira EA, Weber A, Bolte H, Kolesnikova L, Giese S, Lakdawala S, Beer M, Zimmer G, García-Sastre A, Schwemmle M, et al. 2016. A conserved influenza A virus nucleoprotein code controls specific viral genome packaging. Nat Commun 7: e01248-16. 10.1038/ncomms12861 [Europe PMC free article] [Abstract] [CrossRef] [Google Scholar]
- Muramoto Y, Takada A, Fujii K, Noda T, Iwatsuki-Horimoto K, Watanabe S, Horimoto T, Kida H, Kawaoka Y. 2006. Hierarchy among viral RNA (vRNA) segments in their role in vRNA incorporation into influenza A virions. J Virol 80: 2318–2325. 10.1128/JVI.80.5.2318-2325.2006 [Europe PMC free article] [Abstract] [CrossRef] [Google Scholar]
- Murti KG, Webster RG, Jones IM. 1988. Localization of RNA polymerases on influenza viral ribonucleoproteins by immunogold labeling. Virology 164: 562–566. 10.1016/0042-6822(88)90574-0 [Abstract] [CrossRef] [Google Scholar]
- Nakajima K, Sugiura A. 1977. Three-factor cross of influenza virus. Virology 81: 486–489. 10.1016/0042-6822(77)90165-9 [Abstract] [CrossRef] [Google Scholar]
- Nakatsu S, Sagara H, Sakai-Tagawa Y, Sugaya N, Noda T, Kawaoka Y. 2016. Complete and incomplete genome packaging of influenza A and B viruses. MBio 7: e01248-16. 10.1128/mBio.01248-16 [Europe PMC free article] [Abstract] [CrossRef] [Google Scholar]
- Nakatsu S, Murakami S, Shindo K, Horimoto T, Sagara H, Noda T, Kawaoka Y. 2018. Influenza C and D viruses package eight organized ribonucleoprotein complexes. J Virol 92: e02084-17. 10.1128/JVI.02084-17 [Europe PMC free article] [Abstract] [CrossRef] [Google Scholar]
- Neumann G, Noda T, Kawaoka Y. 2009. Emergence and pandemic potential of swine-origin H1N1 influenza virus. Nature 459: 931–939. 10.1038/nature08157 [Europe PMC free article] [Abstract] [CrossRef] [Google Scholar]
- Noda T, Kawaoka Y. 2010. Structure of influenza virus ribonucleoprotein complexes and their packaging into virions. Rev Med Virol 20: 380–391. 10.1002/rmv.666 [Europe PMC free article] [Abstract] [CrossRef] [Google Scholar]
- Noda T, Sagara H, Yen A, Takada A, Kida H, Cheng RH, Kawaoka Y. 2006. Architecture of ribonucleoprotein complexes in influenza A virus particles. Nature 439: 490–492. 10.1038/nature04378 [Abstract] [CrossRef] [Google Scholar]
- Noda T, Sugita Y, Aoyama K, Hirase A, Kawakami E, Miyazawa A, Sagara H, Kawaoka Y. 2012. Three-dimensional analysis of ribonucleoprotein complexes in influenza A virus. Nat Commun 3: 639. 10.1038/ncomms1647 [Europe PMC free article] [Abstract] [CrossRef] [Google Scholar]
- Noda T, Murakami S, Nakatsu S, Imai H, Muramoto Y, Shindo K, Sagara H, Kawaoka Y. 2018. Importance of the 1 + 7 configuration of ribonucleoprotein complexes for influenza A virus genome packaging. Nat Commun 9: 54. 10.1038/s41467-017-02517-w [Europe PMC free article] [Abstract] [CrossRef] [Google Scholar]
- Odagiri T, Tashiro M. 1997. Segment-specific noncoding sequences of the influenza virus genome RNA are involved in the specific competition between defective interfering RNA and its progenitor RNA segment at the virion assembly step. J Virol 71: 2138–2145. 10.1128/JVI.71.3.2138-2145.1997 [Europe PMC free article] [Abstract] [CrossRef] [Google Scholar]
- Ozawa M, Fujii K, Muramoto Y, Yamada S, Yamayoshi S, Takada A, Goto H, Horimoto T, Kawaoka Y. 2007. Contributions of two nuclear localization signals of influenza A virus nucleoprotein to viral replication. J Virol 81: 30–41. 10.1128/JVI.01434-06 [Europe PMC free article] [Abstract] [CrossRef] [Google Scholar]
- Ozawa M, Maeda J, Iwatsuki-Horimoto K, Watanabe S, Goto H, Horimoto T, Kawaoka Y. 2009. Nucleotide sequence requirements at the 5′ end of the influenza A virus M RNA segment for efficient virus replication. J Virol 83: 3384–3388. 10.1128/JVI.02513-08 [Europe PMC free article] [Abstract] [CrossRef] [Google Scholar]
- Pons MW, Schulze IT, Hirst GK, Hauser R. 1969. Isolation and characterization of the ribonucleoprotein of influenza virus. Virology 39: 250–259. 10.1016/0042-6822(69)90045-2 [Abstract] [CrossRef] [Google Scholar]
- Robertson JS. 1979. 5′ and 3′ terminal nucleotide sequences of the RNA genome segments of influenza virus. Nucleic Acids Res 6: 3745–3758. 10.1093/nar/6.12.3745 [Europe PMC free article] [Abstract] [CrossRef] [Google Scholar]
- Smith GJ, Vijaykrishna D, Bahl J, Lycett SJ, Worobey M, Pybus OG, Ma SK, Cheung CL, Raghwani J, Bhatt S, et al. 2009. Origins and evolutionary genomics of the 2009 swine-origin H1N1 influenza A epidemic. Nature 459: 1122–1125. 10.1038/nature08182 [Abstract] [CrossRef] [Google Scholar]
- Sugita Y, Sagara H, Noda T, Kawaoka Y. 2013. Configuration of viral ribonucleoprotein complexes within the influenza A virion. J Virol 87: 12879–12884. 10.1128/JVI.02096-13 [Europe PMC free article] [Abstract] [CrossRef] [Google Scholar]
- Takizawa N, Ogura Y, Fujita Y, Noda T, Shigematsu H, Hayashi T, Kurokawa K. 2019. Local structural changes of the influenza A virus ribonucleoprotein complex by single mutations in the specific residues involved in efficient genome packaging. Virology 531: 126–140. 10.1016/j.virol.2019.03.004 [Abstract] [CrossRef] [Google Scholar]
- Taubenberger JK, Reid AH, Lourens RM, Wang R, Jin G, Fanning TG. 2005. Characterization of the 1918 influenza virus polymerase genes. Nature 437: 889–893. 10.1038/nature04230 [Abstract] [CrossRef] [Google Scholar]
- Tomescu AI, Robb NC, Hengrung N, Fodor E, Kapanidis AN. 2014. Single-molecule FRET reveals a corkscrew RNA structure for the polymerase-bound influenza virus promoter. Proc Natl Acad Sci 111: E3335–E3342. 10.1073/pnas.1406056111 [Europe PMC free article] [Abstract] [CrossRef] [Google Scholar]
- Tong S, Li Y, Rivailler P, Conrardy C, Castillo DA, Chen LM, Recuenco S, Ellison JA, Davis CT, York IA, et al. 2012. A distinct lineage of influenza A virus from bats. Proc Natl Acad Sci 109: 4269–4274. 10.1073/pnas.1116200109 [Europe PMC free article] [Abstract] [CrossRef] [Google Scholar]
- Tong S, Zhu X, Li Y, Shi M, Zhang J, Bourgeois M, Yang H, Chen X, Recuenco S, Gomez J, et al. 2013. New World bats harbor diverse influenza A viruses. PLoS Pathog 9: e1003657. 10.1371/journal.ppat.1003657 [Europe PMC free article] [Abstract] [CrossRef] [Google Scholar]
- Venev SV, Zeldovich KB. 2013. Segment self-repulsion is the major driving force of influenza genome packaging. Phys Rev Lett 110: 098104. 10.1103/PhysRevLett.110.098104 [Abstract] [CrossRef] [Google Scholar]
- Villa M, Lässig M. 2017. Fitness cost of reassortment in human influenza. PLoS Pathog 13: e1006685. 10.1371/journal.ppat.1006685 [Europe PMC free article] [Abstract] [CrossRef] [Google Scholar]
- Watanabe T, Watanabe S, Noda T, Fujii Y, Kawaoka Y. 2003. Exploitation of nucleic acid packaging signals to generate a novel influenza virus-based vector stably expressing two foreign genes. J Virol 77: 10575–10583. 10.1128/JVI.77.19.10575-10583.2003 [Europe PMC free article] [Abstract] [CrossRef] [Google Scholar]
- Williams GD, Townsend D, Wylie KM, Kim PJ, Amarasinghe GK, Kutluay SB, Boon ACM. 2018. Nucleotide resolution mapping of influenza A virus nucleoprotein-RNA interactions reveals RNA features required for replication. Nat Commun 9: 465. 10.1038/s41467-018-02886-w [Europe PMC free article] [Abstract] [CrossRef] [Google Scholar]
- Worobey M, Han GZ, Rambaut A. 2014. Genesis and pathogenesis of the 1918 pandemic H1N1 influenza A virus. Proc Natl Acad Sci 111: 8107–8112. 10.1073/pnas.1324197111 [Europe PMC free article] [Abstract] [CrossRef] [Google Scholar]
- York A, Hengrung N, Vreede FT, Huiskonen JT, Fodor E. 2013. Isolation and characterization of the positive-sense replicative intermediate of a negative-strand RNA virus. Proc Natl Acad Sci 110: E4238–E4245. 10.1073/pnas.1315068110 [Europe PMC free article] [Abstract] [CrossRef] [Google Scholar]
Articles from Cold Spring Harbor Perspectives in Medicine are provided here courtesy of Cold Spring Harbor Laboratory Press
Full text links
Read article at publisher's site: https://doi.org/10.1101/cshperspect.a038497
Read article for free, from open access legal sources, via Unpaywall:
http://perspectivesinmedicine.cshlp.org/content/11/7/a038497.full.pdf
Citations & impact
Impact metrics
Citations of article over time
Smart citations by scite.ai
Explore citation contexts and check if this article has been
supported or disputed.
https://scite.ai/reports/10.1101/cshperspect.a038497
Article citations
Incompatible packaging signals and impaired protein functions hinder reassortment of bat H17N10 or H18N11 segment 7 with human H1N1 influenza A viruses.
J Virol, 98(9):e0086424, 20 Aug 2024
Cited by: 0 articles | PMID: 39162567
IAVCP (Influenza A Virus Consensus and Phylogeny): Automatic Identification of the Genomic Sequence of the Influenza A Virus from High-Throughput Sequencing Data.
Viruses, 16(6):873, 29 May 2024
Cited by: 0 articles | PMID: 38932165 | PMCID: PMC11209090
Genetic Reassortment in a Child Coinfected with Two Influenza B Viruses, B/Yamagata Lineage and B/Victoria-Lineage Strains.
Viruses, 16(6):983, 19 Jun 2024
Cited by: 0 articles | PMID: 38932274 | PMCID: PMC11209448
Deep mining of the Sequence Read Archive reveals major genetic innovations in coronaviruses and other nidoviruses of aquatic vertebrates.
PLoS Pathog, 20(4):e1012163, 22 Apr 2024
Cited by: 3 articles | PMID: 38648214 | PMCID: PMC11065284
H3N2 influenza A virus gradually adapts to human-type receptor binding and entry specificity after the start of the 1968 pandemic.
Proc Natl Acad Sci U S A, 120(31):e2304992120, 19 Jul 2023
Cited by: 3 articles | PMID: 37467282 | PMCID: PMC10401031
Go to all (11) article citations
Similar Articles
To arrive at the top five similar articles we use a word-weighted algorithm to compare words from the Title and Abstract of each citation.
Contribution of RNA-RNA Interactions Mediated by the Genome Packaging Signals for the Selective Genome Packaging of Influenza A Virus.
J Virol, 96(6):e0164121, 19 Jan 2022
Cited by: 10 articles | PMID: 35044211 | PMCID: PMC8941900
In vitro vRNA-vRNA interactions in the H1N1 influenza A virus genome.
Microbiol Immunol, 64(3):202-209, 09 Jan 2020
Cited by: 6 articles | PMID: 31840833
Packaging signal of influenza A virus.
Virol J, 18(1):36, 17 Feb 2021
Cited by: 28 articles | PMID: 33596956 | PMCID: PMC7890907
Review Free full text in Europe PMC
The genome-packaging signal of the influenza A virus genome comprises a genome incorporation signal and a genome-bundling signal.
J Virol, 87(21):11316-11322, 07 Aug 2013
Cited by: 63 articles | PMID: 23926345 | PMCID: PMC3807325