Abstract
Free full text

Off balance: Interferons in COVID-19 lung infections
Abstract
Interferons are innate and adaptive cytokines involved in many biological responses, in particular, viral infections. With the final response the result of the balance of the different types of Interferons. Cytokine storms are physiological reactions observed in humans and animals in which the innate immune system causes an uncontrolled and excessive release of pro-inflammatory signaling molecules. The excessive and prolonged presence of these cytokines can cause tissue damage, multisystem organ failure and death. The role of Interferons in virus clearance, tissue damage and cytokine storms are discussed, in view of COVID-19 caused by Severe Acute Respiratory Syndrome Coronavirus 2 (SARS-CoV-2). The imbalance of Type I, Type II and Type III Interferons during a viral infection contribute to the clinical outcome, possibly together with other cytokines, in particular, TNFα, with clear implications for clinical interventions to restore their correct balance.
Introduction
Interferons (IFNs) are essential cytokines that mediate the host resistance response to viral infections. In the absence of IFNs or compromised cellular programs induced by IFNs, vertebrates may be susceptible to lethal viral infections. There are 21 human IFNs, classified in 3 types (16 Type-I: 12 IFNαs, IFNβ, IFNε, IFNκ, and IFNω; 1 Type-II, IFNγ; and 4 Type-III, IFNλ1, IFNλ2, IFNλ3, and IFNλ4), involved in innate and adaptive immune responses against not only to viruses but also to other pathogens as well as to tumors and in tissue repair. In general, the production of distinct IFNs depends on the cell type and is stimulated during viral infection when the viral nucleic acid in the cytoplasm is detected by intracellular pattern recognition receptors (PRRs). Then, the produced and secreted IFNs will bind and activates heterodimeric receptors (IFNRs) on the cells in autocrine and paracrine mechanisms that initiate a complex cascade of signaling, culminating in transcription of hundreds of different Interferon-Stimulated Genes (ISGs) resulting in strong antiviral innate and adaptive immune responses [[1], [2], [3]].
Briefly, Type I and III IFNs bind to their heterodimeric receptors activating the JAK/STAT pathway, which in turn activate the heterotrimeric transcription factor complex ISGF3, which consists of phosphorylated STAT1/STAT2 and IRF9. However, unphosphorylated STAT molecules and IRF9-STAT2 homodimers have also been described [4]. Type II IFN also signals through the JAK/STAT pathway, resulting in the formation of phosphorylated STAT1 homodimers, also known as IFNγ-activated factor (GAF). Activated ISGF3 and GAF, resulting respectively from type I/III and type II IFN actions, translocate to the nucleus and bind IFN-stimulated response elements (ISRE) and gamma-activated sequences (GAS), respectively, in the promoter regions of ISGs (Figure 1). In this review, we will discuss the recent data showing the association of COVID19 severity with the correct balance between the IFN types and other cytokines for a favorable clinical outcome during infection by SARS-CoV-2.
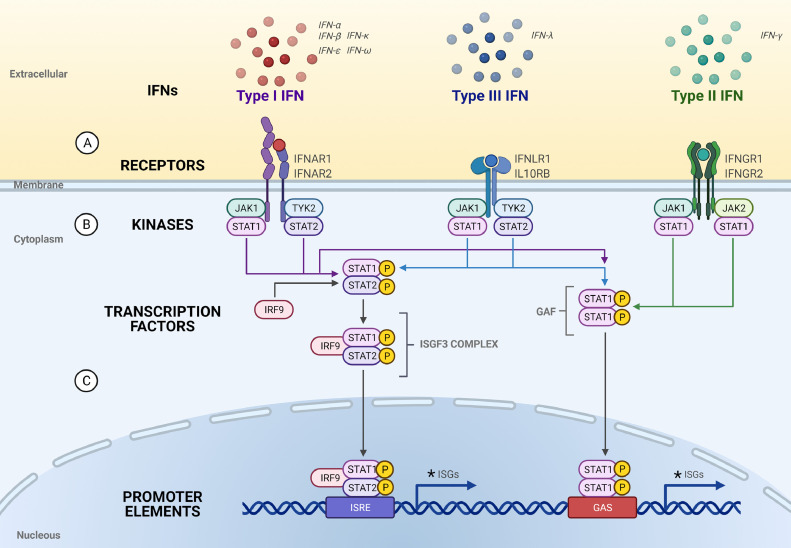
– Type I, II and III Interferons, their receptors and signalings. (A) The Type I, II and III IFN ligands and their receptors; (B) Kinases associated with the Type I, II and III receptors that mediate IFN signallings through receptor activation by ligand binding. Jak1 (Janus Kinase 1) interacts with STAT1 (Signal Transducer and Activator of Transcription 1) as well as to IFNRA1 (Interferon receptor alpha 1). Tyk2 (Tyrosine kinase 2) interacts to STAT2 as well as to IFNRA2. IFNRA1 forms a heterodimer with IFNRA2 that binds with Type I IFN; Jak1 and STAT1 also interacts to IFNLR1. IL-10RB (Interleukin-10 receptor B) also interacts with Tyk2 and STAT2. IFNLR1 forms a heterodimer with IL-10RB that binds to Type III IFNs. Jak1 and STAT1 also interacts to IFNGR1 and JAK2 and STAT1 also interacts with IFNGR2. IFNGR1 forms a heterodimer with IFNGR2 that binds to Type II IFNs; (C) Upon ligand binding and activation, the STAT1 or STAT2 are phosphorylated and the STAT1 homodimers go to the nucleous and activate the transcription of genes with the GAS (gama interferon activation sites) promoters; STAT1 and STAT2 heterodimers binds to IRF9 (interferon regulatory factor 9) and the complex goes to the nucleous and activates the the transcription of genes with the ISRE (Interferon-stimulated responsive element) promoters. Some commom ISGs (trafd1, TNF receptor-associated factor -type zinc finger domain containing 1; parp 9, Poly(ADP-ribose) polymerase family member 9; parp12, Poly(ADP-ribose) polymerase family member 12; parp14, Poly(ADP-ribose) polymerase family member 14; nmi, N-myc and STAT interactor; epsti1, epithelial stromal interaction 1; eif2ak2, eukaryotic translation initiation factor 2 alpha kinase 2; irf1, interferon regulatory factor 1; irf7, interferon regulatory factor 7; irf8, interferon regulatory factor 8; tmem67, transmembrane protein 67; tmem140, transmembrane protein 140; tmem173, transmembrane protein 173; dtx3l, deltex E3 ubiquitin-protein ligase; samhd1, SAM (sterile alpha-motif) and HD (histidine-aspartate) domain containing deoxynucleoside triphosphate truphosphohydrolase 1; stat1, signal transducer and activator of transcription 1; cd274, cluster of differentiation 274) induced by Type I, II and III IFNs are indicated by * [69,70]. (Figure created with BioRender.com).
Coronaviruses (CoVs) are viruses belonging to the family Coronaviridea, with a large positive-sense single-stranded RNA that cause diseases in humans and animals. The new SARS-CoV-2 was identified as a beta-coronavirus responsible for the pandemic COVID-19 (Coronavirus Disease 2019) [5]. Patients with severe COVID-19 present high concentrations of proinflammatory cytokines and chemokines in the plasma, massive infiltration of monocytes and neutrophils (Figure 2).
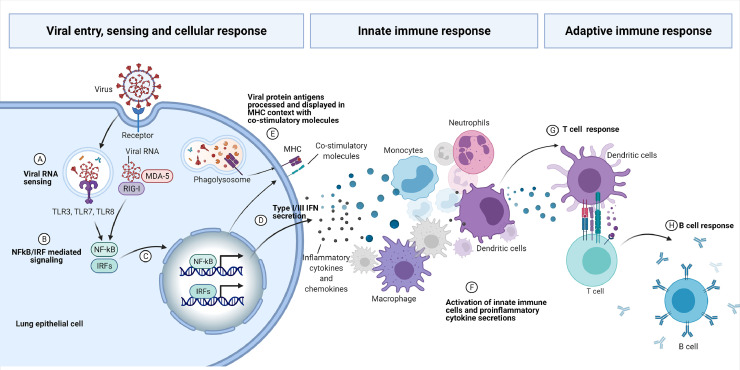
– Schematic view of the viral entry, sensing and host immune response. The RNA virus bind to the cellular receptor and is internalized. Pattern Recognition Receptors (PRRs) sense the viral RNA (A) and activate cellular signaling mediated by NF-κB and IRFs transcription factors (B) to the nucleous (C), activating expression of specific genes, comprising Type I and Type III IFNs and proinflammatory cytokines (D). Viral protein antigens are also processed and displayed in MHC context with co-stimulatory molecules (E). The proinflammatory cytokines and chemokines are responsible for the cellular innate response, with pronounced infiltration of monocytes and neutrophils (F). Activated dendritic cells present the antigens to the adaptive T cells (G) that will secrete cytokines to mature B cells to produce and secrete immunoglobulins (H) (Figure created by BioRender.com).
Types of Interferons (IFNs)
IFNs are components of the innate and adaptive immune systems. Type I IFNs are produced by many cell types including lymphocytes (NK cells, B-cells and T-cells), macrophages, fibroblasts, endothelial cells, osteoblasts, leukocytes, plasmacytoid and dendritic cells [6]. Type II IFN is produced predominantly by natural killer (NK) and natural killer T (NKT) cells as part of the innate immune response, and by CD4+ and CD8+ T cells of the adaptive immune cells [7]. While the main producer of Type III IFNs are type 2 myeloid dendritic cells, epithelial cells and hepatocytes [8,9], the receptors for Type I and Type II interferons are expressed ubiquitously on almost all nucleated cells and Type III interferon receptors are expressed on epithelial, dendritic cells and neutrophils. The antiviral effects of IFNs are dictated by the presence of producer cells and responder cells with IFN receptors. For instance, Type III IFN receptors are restricted to epithelial cells in gastrointestinal, respiratory and reproductive tracts, they are considered as the first line of defense against viruses at these sites [10]. In contrast, all nucleated cells express Type I and II IFN receptors.
Types I and III IFNs in COVID-19
SARS-CoV-2 infects lung alveolar epithelial cells and these cells die due to the virus´ cytopathic effect as viral replication proceeds. Recovery is achieved by proliferation and differentiation of neighboring progenitor epithelial cells. During viral infections, Type I and Type III IFNs are important mediators of the innate immune responses triggered by the recognition of pathogen-associated molecular patterns (PAMPs) by the PRRs of the cell [11]. Type I IFNs are more proinflammatory when compared to Type III IFNs [10]. Surprisingly, although both Type I and Type III IFNs have antiviral activities, mice infected with influenza virus have increased susceptibility to pneumonia caused by Staphylococcus aureus or Streptococcus pneumoniae that were associated with the increase in IFNλ production or IFNλ treatment [[12], [13], [14], [15]]. Similarly, IFNλ mRNAs were observed in bronchoalveolar fluid and naso-oropharyngeal samples of SARS-CoV-2 patients and increases in IFNλ mRNA expression were positively associated with increases in COVID-19 disease morbidity [16]. Interestingly, increases in IFNλ stimulate apoptosis and expression of tumor suppressor p53 that impairs proliferation, differentiation and repair of the lung epithelial cells, increasing disease severity, lung damage and susceptibility to bacterial superinfections (Figure 3F-G) [16,17]. Although Type I (IFNα and IFNβ) also reduces lung epithelial cell proliferation after treatment during influenza, only IFNλ compromises lung epithelial tissue recovery [17]. Moreover, Type I IFN production and activity were highly suppressed in severe COVID-19 patients. These patients present a proinflammatory picture driven by nuclear factor-κB (NF-κB) and characterized by increased IL-6 and TNFα [18]. The reasons for this impaired production, signaling and activity of Type I IFN seems to be related, at least in part, to inborn errors associated to Type I IFN signaling cascade or production of autoantibodies, especially to IFNα or IFNω [[19], [20], [21]]. In addition, it was also shown that the presence of autoantibodies against Type I IFN (IFNα and IFNω) was proportionally higher in males and elderly with severe COVID-19 [20,21]. Altogether, these data suggest an important role of these IFNs in protection and susceptibility to severe pneumonia in COVID-19.
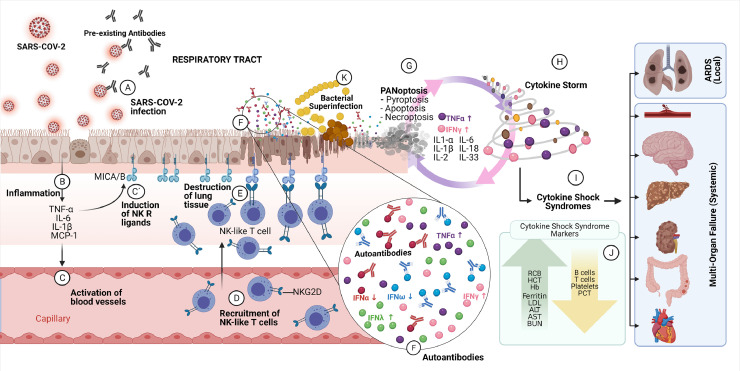
– Respiratory tract infection by SARS-CoV-2. Infection occurs at the epithelial cells of the respiratory tract and the infection may be inhibited by pre-existing cross-reactive antibodies resulted from previous infections with seasonal viruses (A). The infection causes inflammation in the respiratory tract with the secretion of proinflammatory cytokines (B), activation of the endothelial cells (C) and expression of NK receptor ligands (MICA/B, MHC class I chain related protein A or B) on respiratory epithilium (C´). The activated endothelium promotes the infiltration of NK-like T cells expressing NK (Natural Killer) receptors (NKR) exemplified by NKG2D on the cell surface from the capillaries (D) to the respiratory tract epithelium. The infiltrating NK-like T cells binds to NKR receptor ligands (MICA/B) and induce TCR-independent killing of epithelium cells expressing the NKR ligands (E). In response to viral infections, epithelium cells secrete Type I or Type III IFNs. In severe cases of the diseases, the presence of autoantibodies against IFNα and IFNω (F) was observed and associated with higher morbidity, resulting in more viral infections. It was also observed an increase in the secretion of Type III IFN (IFNλ)(F). IFNλ impairs lung epithelial cell proliferation and tissue repair mediated by the expression of the tumor suppressor p53 gene and protein pathway. Cell death programs (PANoptosis) induced by cytokine storms, in special by IFNγ and TNFα, perpetuates the local cytokine storms killing more epithelial cells in the respiratory tract (G) and the cytokine storms propagate (H) to other organs and tissues, provoking cytokine shock syndromes. Acute respiratory distress syndrome (ARDS) will be observed in the patient due to lung damage as well as multi-organ failures (I) due to systemic spread of the proinflammatory cytokines, in special of IFNγ and TNFα. The cytokine shock syndromes can be identified by clinical markers as listed in the figure (J). Susceptibility to bacterial superinfections is increased in damaged respiratory tract (K) due to cell killings by NK-like T cells (E) and by PANoptosis (G) in concert with inhibition of epithelial cell proliferation and repair by IFNλ (F). Cytokine shock sindrome markers: RBC, red blood cells; HCT, hematocrit; Hb, hemoglobin; PCT, Procalcitonin; LDH, lactate dehydrogenase; ALT, alanine aminotransferase; AST; aspartate aminotransferase; BUN, blood urea nitrogen. (Figure created with BioRender.com).
Viral proteins targeting IFN antiviral response
There are many clinical similarities between severe influenza and COVID-19. The COVID-19 clinical manifestations include profound and uncontrolled inflammatory response, massive cytokine release, diffuse alveolar damage and lung infiltration with inflammatory cells, most of them comprised of neutrophils. However, there are also striking differences, related to thrombosis and endothelial dysfunction in COVID-19, which appears to exceed that in influenza patients as observed from lung pathology of fatal cases [22,23].
Both influenza and SARS-CoV-2 have virulence factors targeting the IFN antiviral responses. Influenza hemagglutinins have been shown to facilitate ubiquitination and degradation of Type I IFN receptors, thus reducing the expression levels of Type I IFN receptors and impacting the strength of their responses and ISGs expressions [24,25]. Besides this, the non-structural protein 1 (NS1) inhibits the TRIM25, a RING-finger E3 ubiquitin ligase, blocking RIG-I-mediated innate immunity and suppression of host IFN response. Furthermore, NS1 also inhibits the production of Type I IFNs and downstream effector molecules of IFNs-mediated signal transduction processes by the inhibition of protein kinase RNA-activated (PKR) through the increased expression of host small non-coding RNAs (vault RNAs). As a result, the replication and production of virus particles are also increased [25,26]. NS1 may also affect the phosphorylation of IκB kinases (IKK) and decrease the NF-κB complex in the nucleus and the gene expression mediated by NF-κB. The JAK-STAT pathways of IFNs are also impaired by NS1 that lowers the phosphorylation levels of STAT1, STAT2 and STAT3, preventing proper transcription of ISGs [27]. The transcription of genes is also affected by direct binding of NS1 to host double stranded DNA [28]. Therefore, NS1 is a master key to block the innate immune system, the transcription of IFNs and ISGs through many mechanisms but also of the adaptive immunity by modulating the dendritic cells capacities to induce the T cell responses [29,30]. However, besides NS1, other influenza proteins help to evade Type I IFN actions. PB1-F2 and PB2 influenza viral proteins also interferes with Type I IFN signaling pathway through interaction with MAVS in the mitochondria [[31], [32], [33]]. M2 protein from influenza also affects the generation of IFNs by blocking the host autophagy and TLRs pathways [34].
Although SARS-CoV-2 deregulates cell signaling pathways to generate a permissive environment for viral replication, mainly related to delayed hyperinflammation, generating weakened and delayed IFN responses [35], it was observed that the Spike protein (S) amplifies the activation of IFNβ and the Envelope protein (E) stimulates the transcription of ISGs, in an opposite direction of creating a permissive milieu for SARS-CoV-2 [36]. The Membrane protein (M) and the Nucleocapsid (N) structural proteins inhibits the activation of IFNβ or Type I IFN signaling pathway [36,37]. NSP1 inhibits STAT1 phosphorylation upon IFNα stimulation as well as the IFNβ production in HEK293T cells [36,38]. In contrast, NSP2 accelerates the activation of IFNβ [36]. NSP3, NSP12, NSP13, NSP14, ORF3, ORF6, ORF7 and ORF8 proteins inhibit Sendai virus-induced IFNβ promoter activation, IFNα downstream signaling or NF-κB-responsive promoter [[35], [36], [37], [38], [39]]. ORF9 protein targets the nuclear factor κB (NF-κB) essential modulator NEMO and interrupts its K63-linked polyubiquitination upon viral stimulation, thereby inhibiting the canonical IκB kinase alpha (IKKα)/β/γ-NF-κB signaling and subsequent IFNβ production [40].
Thus, viral proteins in general have diverse roles in disruption of cellular programs to evade and to weaken the host immune response [41] including Influenza and SARS-CoV-2 proteins, most of them as negative effectors targeted to Type I IFN pathway, though positive effectors can also be observed, as in the case of Spike, Envelope and NSP2 viral proteins as discussed above.
Type II IFN in COVID-19
Type II IFN is produced predominantly by natural killer (NK) and natural killer T (NKT) cells as part of the innate immune response, and by CD4+ and CD8+ T cells of the adaptive immune cells [7]. Upon virus infection, IFNγ acts directly on CD8+ T cells to help them to differentiate into cytotoxic T lymphocytes (CTL), which produce cytokines and effector molecules to restrict viral replication and kill virus-infected cells [42] as well as for the increase in CD4+ and CD8+ memory cells [43]. In general, type II IFN connects the innate immune response with the activation of the adaptive immune response [41].
The role of IFNγ during the infection of SARS-CoV-2 is beginning to be elucidated. It was shown that IFNγ is produced in high amount in patients with moderate to severe COVID-19 besides other proinflammatory cytokines. This Type II IFN is found in the serum of these patients and released in the culture medium when peripheral blood mononuclear cells (PBMCs) are infected with SARS-CoV-2 [44]. However, from the ten different cytokines that have their concentration increased in moderate to severe COVID-19 patients (IL-6, IL-18, IFNγ, IL-15, TNFα, IL-1α, TNFβ, IL-1β, IL-33, IL-2), only the combination of IFNγ and TNFα induced inflammatory cell death characterized by pyroptosis, apoptosis and necroptosis in bone marrow-derived macrophages (BMDM), a process known as PANoptosis [44] (Figure 3A-J). Neither IFNγ nor TNFα alone or in combination with the other inflammatory cytokines were able to induce this response. Interestingly, combination of IFNγ and TNFα provoked a lethal cytokine shock in mouse model that was completely abolished by IFNγ and TNFα blockage by neutralizing antibodies [44]. Though these results associate the important role of IFNγ and TNFα combination in the cytokine storm and acute lung damage with patient mortality as observed in severe cases of COVID-19, recent results also associate recovery-like stage of patients with enhanced TNFα and IFN-γ gene expression [45]. Therefore, the role of these cytokines in COVID-19 has to be confirmed in future studies.
COVID-19 severity in male and older people is associated with exacerbated inflammation in the respiratory tract
COVID-19 mostly induces mild to moderate clinical symptoms in younger patients. However, SARS-CoV-2 causes higher morbidity and mortality in older patients, with exacerbated inflammation of their respiratory tracts [46,47]. Interestingly, older healthy individuals present a chronic low-grade sterile systemic inflammation (an inflammatory response not induced by a pathogen), a process known as inflamm-aging, with a higher baseline concentration of C-reactive protein (CRP) and some pro-inflammatory cytokines (IL-1β, IL-6, IL8). The mechanisms that lead to pro- inflamm-aging are not completely understood but may involve impaired clearance of dead and dying cells with sustained inflammation, misfolded proteins, compromised gut barrier function, obesity and senescent cells which no longer divide but keep secreting pro-inflammatory cytokines, chemokines, growth factors and matrix metalloproteinases (MMPs), causing organ dysfunction during the aging process [[48], [49], [50], [51]]. Interestingly, individuals in the same age group that do not present the inflamm-aging profile are healthier and live longer when compared with those with the inflamm-aging signs [48].
Although inflammation is essential to modulate both innate and adaptive immune response at the initial phases of immune stimulation, the inflammatory process is actively regulated by many processes, including the clearance of dead and dying cells and other mechanisms [49]. An excessive and non-resolved inflammation can inhibit antigen-specific immunity in vivo, as shown in the case of viral cancers [52].
It is also compelling that the inflamm-aging phenotype may contribute in exacerbating the hyperinflammation observed in older COVID-19 patients. The inflammation in the lungs of COVID-19 patients may involve the infiltration of monocytes, induction of Natural Killer Receptor (NKR) ligands (MICA/B) by cytokines produced in non-lymphoid cells [53], such as the pulmonary respiratory epithelial cells. These cells can be targeted and killed by NK-like T cells, possibly contributing to the pulmonary tissue damage in COVID-19 patients (Figure 3A-E) [47]. In contrast, NK cell production and proliferation in elderly is reduced and in elderly patients with COVID-19, the NK cells present in addition, an exhausted phenotype which affects the host defense against the SARS-CoV-2 acute infection in addition to the increased secretion of inflammatory cytokines [54,55].
Recent data showing that preexisting antibodies against seasonal human coronavirus able to cross-react with SARS-CoV-2 may also account for the clinical differences observed in children and adolescents versus elderly [[56], [57], [58]]. Children and adolescents are more recurrently infected by seasonal coronaviruses than elderly [58] and they present the highest amounts of cross-reactive antibodies between seasonal coronaviruses and SARS-CoV-2. Therefore, it is conceivable that sustained infection by seasonal coronavirus in children and adolescents induces protective cross-reactive antibodies and these preexisting antibodies influences the severity of COVID-19 by SARS-CoV-2 infection, in contrast to elderly. It is also possible that both mechanisms, inflammaging and preexisting antibodies influences the clinical outcome differences observed in children, adolescents and elderly (Figure 3A).
Males present a significantly higher COVID-19 mortality risk [59,60]. The plasma concentration of inflammatory cytokines and chemokines such as IL-8 and IL-18 are increased in males infected with SARS-CoV-2 when compared to females. The exacerbated systemic inflammation is associated with severe pulmonary pathology, which includes pronounced infiltration of monocytes and neutrophils [[61], [62], [63]]. Interestingly, it is well described that androgens drive the expression of Transmembrane protease serine 2 (TMPRSS2) in the lung and prostate. TMPRSS2 activates the spike protein by cleaving the S1/S2 and S2 sites of SARS-CoV-2 virus spike proteins. This cleavage step is necessary for the virus-host cell membrane fusion and cell entry mediated by the cellular receptor ACE2 that may account for some of the observed gender differences [64]. On the other hand, female patients have higher concentration of Type I IFN (IFNα) in their plasma that might also contribute to less disease severity [60]. Therefore, differences in Type I IFN expression may also account for distinct severities in COVID-19 or other viral pneumonias together with inflamm-aging condition, gender differences and pre-existing antibodies.
Future Directions
Altogether, these observations suggest several complex mechanisms SARS-CoV-2 induces for the diseases severities observed. Activation of NK-like T cells that bind to MICA/B and kill the MICA/B expressing cells is one possible mechanism. This response must be concerted with other mechanisms. Many recent studies have described that a fine balance between type I and type III IFN is critical for optimal protection and minimal host damage [16,17]. Deviation from this finely tuned balance can harm the patient through the unleashing of a cytokine storm. The mechanisms that may contribute to this balance could be the viral and host genetic backgrounds, previous infections leading to the presence of pre-existing viral antibodies, autoantibodies against Type I IFN (in particular, IFNα and IFNω) or increased expression of IFNλ associated with poor lung epithelial cell/tissue repair processes [[8], [9], [10], [11], [12], [13], [14], [15], [16], [17], [18], [19], [20]]. Adding to this complex scenario, IFNγ and TNFα combination induced PANaptosis helps to perpetuate the cytokine storm and the disease progression with more lung epithelial cell death, tissue damage and increased susceptibility to bacterial superinfection [44]. Future therapeutic interventions have to consider these observations and interplays. It seems that binding of NK-like T cells to MICA/B presenting cells, IFNλ, IFNγ, TNFα and their associated signaling pathways should be targeted to increase tissue proliferation and repair, while decreasing damage to lung epithelial cell/tissue (for instance, interventions with neutralizing antibodies against Anti-TNFα, Anti-IFNγ, Anti-IFNλ or with JAK1/2 inhibitors, STAT1 inhibitors, NO inhibitor, PANoptosis inhibitors). Blockage of IFNγ and TNFα by neutralizing antibodies may increase survival and inhibit cell PANoptosis after SARS-CoV-2 infection or in other pathologies associated to cytokine storm. At the same time, treatment with Type I IFN (IFNα, IFNβ IFNω) might be effective in diminishing SARS-CoV-2, influenza and other respiratory viral infections. However, it is not clear how much we have to modulate/change the concentration of these molecules systemically for a proper clinical response and if the activation of these pathways can exacerbate the disease outcome since the SARS-CoV-2 cellular receptor ACE2 expression is increased in human nasal epithelia and lung tissue by Type I and II IFNs and also by Influenza infection [65]. All the possible interventions discussed above have to be monitored and provide qualitative and quantitative data for treatment decision and planning of patients with different ages, genders and co-morbilities. Furthermore, in light of pre-existing antibodies against the SARS-CoV-2, the presence of autoantibodies against Type I IFNs and also of inborn errors of Type I IFN that would affect the therapeutic response, it is important to generate information concerning the Type I IFN pathway integrity for a proper evaluation of the possible therapeutic intervention. At the current stage, the COVID-19 Treatment Guidelines Panel from National Institute of Health (NIH, USA) recommends against the use of interferons for the treatment of patients with severe or critical COVID-19, except in a clinical trial. There are also insufficient data to recommend either for or against the use of interferon beta for the treatment of early (i.e., <7 days from symptom onset) mild and moderate COVID-19 [66], though some recent trials showed some clinical benefits [[67], [68], [69]].
Outstanding Questions
The contributions of IFNs in viral infection responses is becoming clear. In SARS-CoV-2 infections, how can we restore the correct IFNs balances? What are the quantitative values for a proper clinical correction in the IFNs balances? What are the possible adjuvant therapies to be included for viral clearance with acceptable side effects in patients? How does TNFα affect IFNγ activities and vice-versa? Does TNFα interact with Type I or Type III IFNs in epithelial tissue proliferation and repair? Can the use of IFNs or influenza infection increase SARS-CoV-2 infections by the increase of its cellular receptor ACE2? Are these interplays occurring in the infections with the new strains of SARS-CoV-2?
Search Strategy
Data search and selection were identified through searches from PubMed with the following search terms: “COVID-19”, “SARS-CoV-2”, “Influenza”, “Interferons”, “Cytokine storms”, “immune response”. Only articles published in English between 2005 and 2021 were included.
Contributors
All the authors contributed in the discussion, writing, literature searches and revision of the manuscript. All the Figures were mentored and designed by PLH and MAA
Declaration of Competing Interest
The authors declare that the research was conducted in the absence of any commercial or financial relationships that could be construed as a potential conflict of interest.
Acknowledgments
The authors would like to thank the financial support of the Brazilian financial agencies Fapesp (Grant 2020/07040-1 to PLH), CNPq (Grant 305430/2019-0 to PLH) and Fundação Butantan as well as all the comments received from the reviewers. We also declare that the funders had any role in paper design, data collection, data analysis, interpretation and writing of the paper.
References
Articles from eBioMedicine are provided here courtesy of Elsevier
Citations & impact
Impact metrics
Citations of article over time
Alternative metrics

Discover the attention surrounding your research
https://www.altmetric.com/details/115508869
Article citations
Deficiency of Tlr7 and Irf7 in mice increases the severity of COVID-19 through the reduced interferon production.
Commun Biol, 7(1):1162, 17 Sep 2024
Cited by: 0 articles | PMID: 39289468 | PMCID: PMC11408513
Delayed Mucosal Antiviral Responses Despite Robust Peripheral Inflammation in Fatal COVID-19.
J Infect Dis, 230(1):e17-e29, 01 Jul 2024
Cited by: 2 articles | PMID: 39052740 | PMCID: PMC11272059
Select amino acids recover cytokine-altered ENaC function in human bronchial epithelial cells.
PLoS One, 19(7):e0307809, 25 Jul 2024
Cited by: 0 articles | PMID: 39052685 | PMCID: PMC11271875
Necroptosis does not drive disease pathogenesis in a mouse infective model of SARS-CoV-2 in vivo.
Cell Death Dis, 15(1):100, 30 Jan 2024
Cited by: 4 articles | PMID: 38286985 | PMCID: PMC10825138
Airway epithelial CD47 plays a critical role in inducing influenza virus-mediated bacterial super-infection.
Nat Commun, 15(1):3666, 30 Apr 2024
Cited by: 0 articles | PMID: 38693120 | PMCID: PMC11063069
Go to all (30) article citations
Similar Articles
To arrive at the top five similar articles we use a word-weighted algorithm to compare words from the Title and Abstract of each citation.
Dysregulation of the immune response in coronavirus disease 2019.
Cell Biol Int, 45(4):702-707, 11 Dec 2020
Cited by: 33 articles | PMID: 33289192 | PMCID: PMC7753769
Review Free full text in Europe PMC
Mathematical Modeling of Severe Acute Respiratory Syndrome Coronavirus 2 Infection Network with Cytokine Storm, Oxidative Stress, Thrombosis, Insulin Resistance, and Nitric Oxide Pathways.
OMICS, 25(12):770-781, 22 Nov 2021
Cited by: 7 articles | PMID: 34807729
Cytokine storm in COVID-19: from viral infection to immune responses, diagnosis and therapy.
Int J Biol Sci, 18(2):459-472, 01 Jan 2022
Cited by: 53 articles | PMID: 35002503 | PMCID: PMC8741849
Review Free full text in Europe PMC
Autoinflammatory Diseases and Cytokine Storms-Imbalances of Innate and Adaptative Immunity.
Int J Mol Sci, 22(20):11241, 18 Oct 2021
Cited by: 16 articles | PMID: 34681901 | PMCID: PMC8541037
Review Free full text in Europe PMC
Funding
Funders who supported this work.
Conselho Nacional de Desenvolvimento Científico e Tecnológico (1)
Grant ID: 305430/2019-0
Fundação Butantan
Fundação de Amparo à Pesquisa do Estado de São Paulo (1)
Grant ID: 2020/07040-1