Abstract
Free full text

Prenatal and prepubertal exposures to tobacco smoke in men may cause lower lung function in future offspring: a three-generation study using a causal modelling approach
Associated Data
Abstract
Mechanistic research suggests that lifestyle and environmental factors impact respiratory health across generations by epigenetic changes transmitted through male germ cells. Evidence from studies on humans is very limited.
We investigated multigeneration causal associations to estimate the causal effects of tobacco smoking on lung function within the paternal line. We analysed data from 383 adult offspring (age 18–47 years; 52.0% female) and their 274 fathers, who had participated in the European Community Respiratory Health Survey (ECRHS)/Respiratory Health in Northern Europe, Spain and Australia (RHINESSA) generation study and had provided valid measures of pre-bronchodilator lung function. Two counterfactual-based, multilevel mediation models were developed with: paternal grandmothers’ smoking in pregnancy and fathers’ smoking initiation in prepuberty as exposures; fathers’ forced expiratory volume in 1 s (FEV1) and forced vital capacity (FVC), or FEV1/FVC z-scores as potential mediators (proxies of unobserved biological mechanisms that are true mediators); and offspring's FEV1 and FVC, or FEV1/FVC z-scores as outcomes. All effects were summarised as differences (Δ) in expected z-scores related to fathers’ and grandmothers’ smoking history.
Fathers’ smoking initiation in prepuberty had a negative direct effect on both offspring's FEV1 (Δz-score –0.36, 95% CI −0.63–−0.10) and FVC (−0.50, 95% CI −0.80–
−0.20) compared with fathers’ never smoking. Paternal grandmothers’ smoking in pregnancy had a negative direct effect on fathers’ FEV1/FVC (−0.57, 95% CI −1.09–
−0.05) and a negative indirect effect on offspring's FEV1/FVC (−0.12, 95% CI −0.21–
−0.03) compared with grandmothers’ not smoking before fathers’ birth nor during fathers’ childhood.
Fathers’ smoking in prepuberty and paternal grandmothers’ smoking in pregnancy may cause lower lung function in offspring. Our results support the concept that lifestyle-related exposures during these susceptibility periods influence the health of future generations.
Short abstract
Fathers’ prepuberty and paternal grandmothers’ pregnancy are vulnerable periods to the adverse effects of smoking on offspring's lung function. Preventing smoking in these susceptibility time windows might improve the next generation's health. https://bit.ly/3vvgjsN
Introduction
Asthma and chronic obstructive pulmonary disease are major health challenges across the world [1, 2]. Lifestyle and environmental exposures are important risk factors for these diseases [3, 4], not least when exposure occurs in early life. Emerging evidence suggests that the environment before conception may impair respiratory health of the offspring [5, 6], as supported by animal research [7–9]. In line with this hypothesis, we previously found negative associations between fathers’ smoking before 15 years of age (a period compatible with prepuberty, as 15 years is the mean age of completed puberty in boys [10]) and their offspring's asthma phenotypes [11, 12]. We also identified the same susceptibility time window for fathers’ overweight [13].
Studies on human health [14], including respiratory diseases [15–18], are currently more focused on epigenetics. Heritable modification of DNA methylation may be a key mechanism for the effects of preconception and early-life exposures on disease phenotypes in subsequent generations. Fetal life and prepuberty are time windows with a higher susceptibility to environmental exposures through their impact on epigenetic programming of the male germ cell line [19, 20]. These vulnerable periods may represent windows of opportunity for preventive interventions in males aimed at improving the health of current and future generations.
Causal statistical inference [21] can contribute to shed light on the mechanisms involved in the pathways from exposures in different generations and susceptibility periods to health outcomes in subsequent generations. A multiple-exposure, multiple-mediator, multiple-outcome (MEMMMO) framework has recently been proposed for the identification of complex multigeneration effects [22]. In line with this new perspective, we investigated causal associations to estimate the causal effects of fathers’ smoking initiation in prepuberty (generation G1) and grandmothers’ smoking in pregnancy (generation G0) on offspring's lung function (generation G2) within the paternal line. As part of the Ageing Lungs in European Cohorts (ALEC) Study (www.alecstudy.org), we analysed data from an ongoing survey on respiratory health in adults (European Community Respiratory Health Survey (ECRHS); www.ecrhs.org) and its extension to the generation of their offspring (Respiratory Health in Northern Europe, Spain and Australia (RHINESSA); www.rhinessa.net).
Methods
Study design
ECRHS is an international, population-based, cohort study on respiratory health in subjects aged 20–44 years at the time of recruitment (ECRHS I; 1991–1993) [23]. At baseline, each participant was sent a brief screening questionnaire (stage 1) and, from those who responded, a 20% “random sample” was invited to undergo a more detailed clinical examination (stage 2). An additional “symptomatic sample” of adults with asthma-like symptoms was also recruited at stage 2. The follow-up of the participants in stage 2 took place in 1998–2002 (ECRHS II) [24] and in 2010–2013 (ECRHS III) [25]. These subjects underwent a standardised clinical interview and lung function and laboratory tests on all the occasions. RHINESSA is an international study on health and disease in the offspring of the ECRHS participants in 10 centres from Northern Europe, Spain and Australia [13]. Extensive questionnaire and lung function data were collected in 2013–2016, based on protocols adapted to those used in the ECRHS. Written informed consent was obtained from each participant in the ECRHS/RHINESSA studies. Ethics approval was obtained by the appropriate ethics committee in each centre (supplementary material).
Lung function and definitions
Maximum pre-bronchodilator forced expiratory volume in 1 s (FEV1) and forced vital capacity (FVC) from at least two technically satisfactory manoeuvres were measured according to the American Thoracic Society (ATS) criteria for repeatability [26], as part of the ECRHS and RHINESSA clinical examinations. Post-bronchodilator spirometry was also measured in ECRHS at the last follow-up contact (ECRHS III) and in RHINESSA. In the present analyses, we used fathers’ lung function measurements at baseline (ECRHS I) or at the first available occasion (ECRHS II or ECRHS III) if baseline spirometry had not been performed or had not fulfilled the ATS criteria. The Global Lung Function Initiative z-scores [27] were calculated by using the European Respiratory Society freeware software (www.ers-education.org/guidelines/global-lung-function-initiative/spirometry-tools/desktop-sheet-calculator.aspx), in order to control for the dependency of lung function on sex, age and height.
The fathers provided detailed information on their own smoking history, including when they had started smoking, during interviews at each ECRHS examination. Fathers’ smoking was categorised as “smoking initiation before 15 years of age” (i.e. during prepuberty), “smoking initiation at 15 years of age or older” or “never smoking”. Fathers’ education level was self-reported at ECRHS I and was considered “low” if less than or equal to the minimum school-leaving age in their country before the start of the ECRHS study [28]. Fathers’ occupational class was derived from the longest-held job during the follow-up period between ECRHS I and II, with categories based on the major group classification in the International Standard Classification of Occupations [29]. At ECRHS I, the fathers reported whether their mother had smoked before or after their birth. Consequently, grandmothers’ smoking was classified as “smoking when the father was in utero”, “smoking before pregnancy or during father's childhood (or unknown smoking period)” or “not smoking before father's birth nor during father's childhood”. At ECRHS III, the fathers provided information on their parents’ education level (defined as “low” if both grandparents were reported to have only studied up to the minimum school-leaving age). In RHINESSA, the offspring reported their own smoking history and education level (defined as “low” if a subject had only studied up to the minimum school-leaving age), and whether their mother had smoked before or after their birth, during interviews with similarly worded questions to the ECRHS.
Study subjects
Of the 2302 males from Estonia (Tartu), Norway (Bergen), Spain (Albacete and Huelva) and Sweden (Gothenburg, Umeå and Uppsala) who had undergone a clinical examination in ECRHS I–III, 1241 participated in ECRHS III. Of these, 913 males reported having at least one offspring and 297 of them had at least one adult offspring who participated in the RHINESSA clinical stage and were thus eligible for inclusion in the present analyses (figure 1). These fathers had valid lung function measurements, and reported complete information on their own and their mother's smoking history. Of the 420 adult offspring who had originated from these 297 fathers, 383 individuals had valid lung function measurements. These offspring and their 274 fathers (92.7% from the ECRHS “random sample”) were included in our study.
Statistical analyses
Counterfactual-based mediation analyses [30] within a hierarchical framework were carried out to investigate the pathways among grandmothers’ and fathers’ tobacco smoking in vulnerable periods and offspring's lung function within the paternal line. Our data have a hierarchical structure because the offspring siblings (level 1 units) have the same biological father (level 2 units) and because their fathers were sampled from different centres (level 3 units). The total effect of each exposure on each outcome was decomposed into its natural direct effect (i.e. the effect of the exposure on the outcome via a pathway that does not involve the mediator) and its natural indirect (mediated) effect (i.e. the effect of the exposure on the outcome due to the effect of the exposure on the mediator) [31]. The main requirement for mediation is that the indirect effect is statistically significant [32], when the observed mediated effect is robust to potential confounding by some unmeasured variable [33].
Two multilevel mediation models were used within the paternal line. Model 1 and model 2 were aimed at estimating the multigeneration effects of smoking on pre-bronchodilator FEV1 and FVC (figure 2), or FEV1/FVC (figure 3), respectively. Model 1 included fathers’ FEV1 and FVC z-scores as the normally distributed, correlated, parallel mediators, and offspring's FEV1 and FVC z-scores as the normally distributed, correlated, parallel outcomes. Model 2 included fathers’ FEV1/FVC z-score as the normally distributed mediator and offspring's FEV1/FVC z-score as the normally distributed outcome. Each lung function variable in fathers (measured in adulthood) was considered a proxy of unobserved biological mechanisms that are true mediators. Latent mediators were not included in the models as we had a single indicator for each mediator and using single-indicator latent variables often causes identification problems in model specification [34]. In both models, grandmothers’ and fathers’ smoking were the exposures of interest, and grandparents’ and fathers’ education level and fathers’ occupational class were analysed as potential confounders. We verified whether these potential confounders represent the “minimal sufficient adjustment set” (i.e. the smallest set of measured covariates that needs to be included in order to eliminate confounding) by using directed acyclic graphs [35] in DAGitty (http://dagitty.net; see supplementary material). In addition, fathers’ age, mothers’ smoking before or after offspring's birth, and offspring's sex, age, education level and smoking were analysed as adjusting variables of the exposure–mediator–outcome relationships.

Mediation model for forced expiratory volume in 1 s (FEV1) and forced vital capacity (FVC) within the paternal line (model 1). The green boxes represent the exposures of interest, the yellow boxes the mediators and the blue boxes the outcomes. The dotted boxes represent the set of potential confounders and adjusting variables of the mediators (X1: grandparents’ education level, and fathers’ age, education level and occupational class) and of the outcomes (X2: fathers’ education level and occupational class, mother's smoking before or after offspring's birth, and offspring's age, sex, education level and smoking). The two ellipses represent the level 2 unit (father; the arrows indicate the random intercept terms at level 2) and the cluster variable (country; no arrow indicates that cluster-robust standard errors were computed in order to take the correlation among fathers within countries into account).
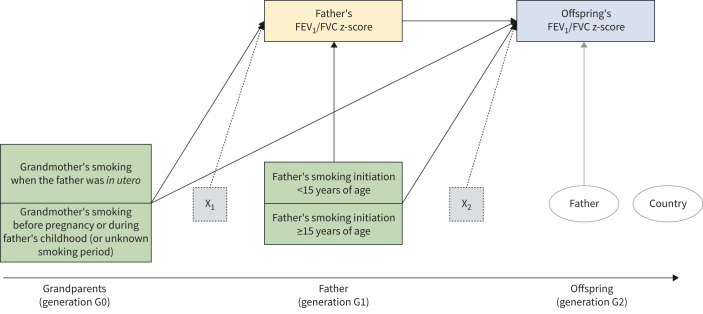
Mediation model for forced expiratory volume in 1 s (FEV1)/forced vital capacity (FVC) within the paternal line (model 2). The green boxes represent the exposures of interest, the yellow box the mediator and the blue box the outcome. The dotted boxes represent the set of potential confounders and adjusting variables of the mediator (X1: grandparents’ education level, and fathers’ age, education level and occupational class) and of the outcome (X2: fathers’ education level and occupational class, mother's smoking before or after offspring's birth, and offspring's age, sex, education level and smoking). The two ellipses represent the level 2 unit (father; the arrow indicates the random intercept term at level 2) and the cluster variable (country; no arrow indicates that cluster-robust standard errors were computed in order to take the correlation among fathers within countries into account).
Model 1 and model 2 had random intercept terms at level 2 (father), cluster-robust standard errors (country=cluster variable) and a “2→2→1” configuration, i.e. the exposures and the mediators were measured at level 2 (father), whereas the outcomes were measured at level 1 (offspring) [36]. The natural (counterfactual-based) direct and indirect effects of the exposures (grandmothers’ and fathers’ smoking) on the normally distributed mediators (fathers’ z-scores) and outcomes (offspring's z-scores) were summarised as differences (Δ) in expected z-scores. The natural direct effect is offspring's Δz-score for the change in exposure status, keeping fathers’ z-score at its expected value when the exposure is absent. The natural indirect effect is offspring's Δz-score when the exposure is present, but fathers’ z-score changes from its expected value when the exposure is absent to its expected value when the exposure is present. The magnitude of the direct and indirect effects and their 95% confidence intervals were computed based on the maximum likelihood estimator with robust standard errors [36] and the distribution-of-the-product method [37], respectively.
Stata version 15 (StataCorp, College Station, TX, USA), Mplus version 8 (Muthén & Muthén, Los Angeles, CA, USA) and R version 3.6.1 (www.R-project.org) were used for the statistical analyses.
Sensitivity analyses
Sensitivity analyses were performed in order to assess: 1) whether the estimated effects changed after the inclusion of up to two unmeasured confounders (U1 and U2) in model 1 and model 2, by using probabilistic (Monte Carlo) simulations in the Umediation package [38] (https://github.com/SharonLutz/Umediation; see supplementary material); 2) which is the minimum strength of association that an unmeasured confounder would need to have with both the mediator and outcome, conditional on the measured confounders, to fully explain away the observed direct or indirect effects (mediational E-values) [39], by using the Evalue package [40] (https://github.com/mayamathur/evalue_package). The mediational E-values were returned on the risk ratio scale through an approximate conversion [41, 42]; 3) whether the estimated effects of fathers’ smoking in prepuberty changed when the analyses were repeated by excluding the 182 offspring whose father had smoked after their birth; and 4) whether the estimated effects changed when using offspring's post-bronchodilator lung function measurements (available from 369 offspring).
Results
Main characteristics of the study subjects
At the time of lung function assessment, the median age of the 274 fathers and their 383 offspring (52.0% female) was 37 and 28 years, respectively (tables 1 and and2).2). In our sample, 10.2%, 8.4% and 7.7% of the fathers had FEV1, FVC or FEV1/FVC z-scores below −1.645 (fifth percentile), respectively, whereas these values were 6.0%, 3.7% and 6.8% for their offspring. Among the fathers, 9.1% had started smoking before 15 years of age (i.e. during prepuberty) and 7.7% reported that their mother (grandmothers in the present analyses) had smoked during their pregnancy. Among the offspring, the percentage of ever-smokers was 30.3%, and 50.7% reported that their mother had smoked before or after their birth.
TABLE 1
Main characteristics of the fathers (n=274) and father-reported information on their parents (grandparents)
Grandparents (generation G0) | |
![]() | |
![]() ![]() | 31.0 |
![]() ![]() | 36.9 |
![]() ![]() | 32.1 |
![]() | |
![]() ![]() | 7.7 |
![]() ![]() | 18.6 |
![]() ![]() | 73.7 |
Father (generation G1) | |
![]() | 37 (21–63) |
![]() | −0.35±1.09 |
![]() | −0.27±0.97 |
![]() | −0.16±1.01 |
![]() | 7.7 |
![]() | |
![]() ![]() | 37.2 |
![]() ![]() | 15.3 |
![]() ![]() | 8.4 |
![]() ![]() | 14.6 |
![]() ![]() | 11.3 |
![]() ![]() | 13.2 |
![]() | |
![]() ![]() | 9.1 |
![]() ![]() | 52.6 |
![]() ![]() | 38.3 |
Data are presented as %, median (range) or mean±sd. FEV1: forced expiratory volume in 1 s; FVC: forced vital capacity; ECRHS: European Community Respiratory Health Survey. #: both grandparents were reported to have only studied up to the minimum school-leaving age; ¶: less than or equal to the minimum school-leaving age in their country before the start of the ECRHS [28]; +: derived from the longest-held job during the follow-up period between ECRHS I and II, using the International Standard Classification of Occupations [29].
TABLE 2
Main characteristics of the offspring (n=383) and offspring-reported information on their mothers
Mother (generation G1) | |
![]() | |
![]() ![]() | 50.7 |
![]() ![]() | 25.3 |
![]() ![]() | 24.0 |
Offspring (generation G2) | |
![]() | 52.0 |
![]() | 28 (18–47) |
![]() | −0.38±0.75 |
![]() | −0.23±0.83 |
![]() | −0.26±0.91 |
![]() | 30.3 |
![]() | |
![]() ![]() | 37.9 |
![]() ![]() | 57.4 |
![]() ![]() | 4.7 |
Data are presented as %, median (range) or mean±sd. FEV1: forced expiratory volume in 1 s; FVC: forced vital capacity. #: offspring had only studied up to the minimum school-leaving age.
Fathers’ smoking initiation in prepuberty
Fathers’ smoking initiation in prepuberty (generation G1) had a negative direct effect on their own FEV1/FVC (Δz-score −0.36, 95% CI −0.68–−0.04) compared with fathers’ never smoking (table 3). This exposure had a negative direct effect on both offspring's FEV1 (−0.36, 95% CI −0.63–
−0.10) and FVC (−0.50, 95% CI −0.80–
−0.20) (generation G2), but we did not observe a statistically significant effect on offspring's FEV1/FVC. No mediated effect of fathers’ smoking in prepuberty on offspring's pulmonary values was identified (direct-only nonmediation) (table 4). Fathers’ smoking initiation at later ages had a negative direct effect on their own FEV1 (−0.27, 95% CI −0.51–
−0.02) and FEV1/FVC (−0.20, 95% CI −0.37–
−0.04), but we did not find an effect on offspring's lung function.
TABLE 3
Natural direct effects# on fathers’ and offspring's pre-bronchodilator forced expiratory volume in 1 s (FEV1) and forced vital capacity (FVC) (model 1), or FEV1/FVC (model 2) z-scores within the paternal line
Father (generation G1) | Offspring (generation G2) | |||||
ΔFEV1
(95% CI) |
ΔFVC
(95% CI) |
ΔFEV1/FVC
(95% CI) |
ΔFEV1
(95% CI) |
ΔFVC
(95% CI) |
ΔFEV1/FVC
(95% CI) | |
Grandparents (generation G0) | ||||||
![]() | ||||||
![]() ![]() | −0.33 (−0.89–0.23) | −0.05 (−0.54–0.44) | −0.57 (−1.09– ![]() | −0.03 (−0.35–0.28) | −0.03 (−0.39–0.34) | 0.01 (−0.09–0.12) |
![]() ![]() ![]() ![]() ![]() ![]() | −0.27 (−0.70–0.17) | −0.11 (−0.35–0.13) | −0.36 (−0.82–0.11) | 0.05 (−0.05–0.14) | 0.05 (−0.08–0.18) | 0.02 (−0.21–0.25) |
Father (generation G1) | ||||||
![]() ![]() | ||||||
![]() ![]() | −0.22 (−0.78–0.34) | −0.05 (−0.64–0.53) | −0.36 (−0.68– ![]() | −0.36 (−0.63– ![]() | −0.50 (−0.80– ![]() | 0.30 (−0.01–0.61) |
![]() ![]() | −0.27 (−0.51– ![]() | −0.11 (−0.32–0.11) | −0.20 (−0.37– ![]() | −0.01 (−0.18–0.16) | −0.04 (−0.23–0.16) | 0.03 (−0.13–0.20) |
![]() ![]() | 0.18 (0.05–0.31)* | |||||
![]() ![]() | 0.17 (0.07–0.27)* | |||||
![]() ![]() | 0.21 (0.07–0.34)* |
#: difference (Δ) in offspring's expected z-score for the change in exposure status, keeping fathers’ z-score at its expected value when the exposure is absent. Models 1 and 2 also include the potential confounders and adjusting variables of the mediators (grandparents’ education level, and fathers’ age, education level and occupational class) and of the outcomes (fathers’ education level and occupational class, mother's smoking before or after offspring's birth, and offspring's age, sex, education level and smoking). ¶: versus not smoking before father's birth nor during father's childhood. *: p<0.05 (statistically significant).
TABLE 4
Natural indirect effects# on offspring's pre-bronchodilator forced expiratory volume in 1 s (FEV1) and forced vital capacity (FVC) (model 1), or FEV1/FVC (model 2) z-scores within the paternal line
Offspring (generation G2) | |||
ΔFEV1
(95% CI) |
ΔFVC
(95% CI) |
ΔFEV1/FVC
(95% CI) | |
Grandparents (generation G0) | |||
![]() | |||
![]() ![]() | −0.06 (−0.17–0.05) | −0.01 (−0.09–0.08) | −0.12 (−0.21– ![]() |
![]() ![]() ![]() ![]() | −0.05 (−0.13–0.03) | −0.02 (−0.06–0.02) | −0.07 (−0.17–0.02) |
Father (generation G1) | |||
![]() | |||
![]() ![]() | −0.04 (−0.14–0.06) | −0.01 (−0.10–0.09) | −0.07 (−0.18–0.02) |
![]() ![]() | −0.05 (−0.10–0.004) | −0.02 (−0.06–0.02) | −0.04 (−0.09–0.01) |
#: difference (Δ) in offspring's expected z-score when the exposure is present, but fathers’ z-score changes from its expected value when the exposure is absent to its expected value when the exposure is present. Models 1 and 2 also include the potential confounders and adjusting variables of the mediators (grandparents’ education level, and fathers’ age, education level and occupational class) and of the outcomes (fathers’ education level and occupational class, mother's smoking before or after offspring's birth, and offspring's age, sex, education level and smoking). ¶: versus not smoking before father's birth nor during father's childhood. *: p<0.05 (statistically significant).
Grandmothers’ smoking in pregnancy
Grandmothers’ smoking when the father was in utero (generation G0) had a negative direct effect on fathers’ FEV1/FVC (generation G1) (−0.57, 95% CI −1.09–−0.05) compared with grandmothers’ not smoking before fathers’ birth nor during fathers’ childhood (table 3). Grandmothers’ smoking while pregnant with the father had no direct effect on grandchildren's lung function (generation G2). This exposure had a negative mediated effect, through unobserved biological mechanisms for which fathers’ FEV1/FVC in adulthood is an indicator, on grandchildren's FEV1/FVC (−0.12, 95% CI −0.21–
−0.03) (indirect-only mediation) (table 4).
Sensitivity analyses
Simulations suggested that unmeasured confounding could reasonably be assumed to have a low impact on the effects of fathers’ and grandmothers’ smoking (supplementary figures S4 and S5). When the effect (β regression coefficient) of each unmeasured confounder (U1 and U2) on the exposure, mediator and outcome was set ≤5, the proportion of simulations where the results matched (whether U1 and U2 were included or excluded from the models) was >72.8% and the average absolute difference of the average effects was <0.083. U1 and U2 changed the results for very strong effects only (i.e. for β regression coefficients >5).
The mediational E-value for the natural direct effect of father's smoking in prepuberty on offspring's FEV1 and FVC was 2.31 and 2.85, respectively, whereas the mediational E-value for the natural indirect effect of grandmother's smoking in pregnancy on offspring's FEV1/FVC was 1.50. Therefore, an unmeasured confounder must be associated with both the mediator and outcome with an approximate risk ratio at least equal to 2.31, 2.85 or 1.50 (when the outcome is FEV1, FVC or FEV1/FVC, respectively) to completely explain away the observed effects.
Exclusion of the offspring of fathers who had smoked after their birth provided stronger estimates of the direct effect of fathers’ smoking in prepuberty on offspring's FEV1 (−0.55, 95% CI −1.18–0.07) and FVC (−0.77, 95% CI −1.61–0.07) than that obtained in the main analysis. However, these estimates did not reach statistical significance because of data sparseness, as only eight offspring were born to seven fathers who had started smoking in prepuberty and had quit smoking before their birth.
When offspring's post-bronchodilator values were used, the direct effect of fathers’ smoking on offspring's FVC (−0.35, 95% CI −0.62–−0.07) and the indirect effect of grandmothers’ smoking on grandchildren's FEV1/FVC (−0.09, 95% CI −0.17–
−0.01) were reduced. However, the direct effect of fathers’ smoking on offspring's FEV1 did not reach statistical significance (−0.19, 95% CI −0.48–0.11).
Discussion
In the present study, we found that fathers’ and paternal grandmothers’ smoking in vulnerable time windows may negatively affect lung function in the next two generations. These results were obtained using a method of causal inference with observational data and were strengthened by sensitivity analyses accounting for unmeasured confounding. Our novel observation is that the offspring of fathers who had smoked during prepuberty may have lower FEV1 and FVC values (suggesting an effect on reducing overall lung volumes) compared with the offspring of fathers who had never smoked. Another key result is the negative indirect effect of grandmothers’ smoking when the father was in utero on grandchildren's FEV1/FVC. This finding suggests that smoking during pregnancy (generation G0) may not only increase the risk of airflow obstruction in generation G1, but it may also have a negative effect on generation G2 within the paternal line. Our results support the concept that lifestyle-related exposures during prepuberty in males and during pregnancy influence the health of future generations.
Fathers’ smoking initiation in prepuberty
In the present analyses, the effect of fathers’ prepubertal smoking on offspring's FEV1 and FVC was observed regardless of the effect of mothers’ smoking before or after offspring's birth, and it was even present after the exclusion of the offspring of fathers who had smoked after their birth. When offspring's post-bronchodilator values were used, the effect on FVC was reduced but it was still statistically significant. Moreover, fathers’ smoking initiation at later ages had no effect on their offspring's lung function.
Unmeasured genetic confounding unlikely explains away the direct effect of paternal early smoking on offspring's FEV1 and FVC, as the few risk loci for both nicotine dependence and lung function identified in genetic studies (such as rs16969968 in the cholinergic receptor nicotinic α5 subunit CHRNA5 gene) [43, 44] have weaker associations than the computed mediational E-values [39]. Therefore, we speculate that our findings could reflect epigenetic alterations (such as DNA methylation, histone modification and microRNAs) in developing germ cells [45–47], leading to reduced lung volumes in offspring. The heritable effects of smoking in young men seem to be biologically plausible, because male prepuberty represents a critical period for germ line development [19, 20], which might give higher susceptibility to tobacco-related effects on the epigenetic profile of gametes. In addition, the observed negative impact of fathers’ prepubertal smoking on offspring's FEV1 and FVC may reflect the direct toxicogenic effects of cigarette smoke on biological processes involved in metabolic health. Prepubertal start of father's smoking may contribute to obesity in adolescent [48] and adult sons [49], and obese adults show a spirometry pattern characterised by lower FEV1, FVC, total lung capacity and residual volume [50].
Our results are supported by findings from previous analyses of the same population, in which we observed that fathers’ smoking initiation in prepuberty was associated with an increased risk for nonallergic asthma in offspring [11, 12]. In addition, we found that fathers’ overweight onset before 15 years of age had a direct effect on nonallergic asthma in the next generation [13]. All these results strengthen the hypothesis that lifestyle and environmental exposures during male prepuberty may affect the respiratory health of future offspring. Our current findings may have considerable public health implications, particularly as tobacco smoking in 11- to 15-year-old boys has increased in different European regions over recent decades [51], as well as the use of moist oral tobacco and e-cigarettes among the very young. Nevertheless, we acknowledge that early smoking in men could also be a marker for other causative factors, as risky behaviours of several kinds can be associated with smoking in adolescents.
Grandmothers’ smoking in pregnancy
Our study highlights that grandmothers’ smoking in pregnancy may negatively affect FEV1/FVC in the next two generations within the paternal line. Accordingly, findings from the UK Biobank indicate an excess reduction in FEV1/FVC among sons due to maternal smoking around delivery, whereas the reduction in FVC is very modest [52]. The negative direct effect of this prenatal exposure on fathers’ lung function is supported by previous evidence in humans and animals [53], because nicotine can penetrate the placental barriers and adversely affect lung development [54]. In addition, maternal smoking during pregnancy is associated with low birthweight and preterm delivery [55, 56], which are linked to reduced lung function in adulthood [57, 58].
We speculate that the negative indirect effect of grandmothers’ smoking during pregnancy (through biological mechanisms for which fathers’ lung function in adulthood is an indicator) on grandchildren's FEV1/FVC could be explained by epigenetic changes [59, 60], resulting in airflow obstruction in offspring [60], and this effect is unlikely fully explained away by unmeasured risk loci, as suggested by the computed mediational E-value [39]. Tobacco smoking may cause heritable changes of the germ cell epigenome, particularly in the prenatal period [60, 61]. An animal model of multigeneration nicotine-induced asthma showed that epigenetic modifications can affect lung function in second-generation offspring [62]. Furthermore, a study on humans highlighted a link between prenatal exposure to tobacco smoke, DNA methylation changes and asthma-related lung function [63].
Strengths and weaknesses
Epidemiological studies on the early-life origins of diseases usually focus on mothers’ exposures immediately before conception and during pregnancy. By contrast, RHINESSA included the offspring of both male and female participants in ECRHS, and the ECRHS survey followed adult men and women through their reproductive age. Thus, the ECRHS/RHINESSA studies collected extensive data on exposures occurring well in advance before conception (together with objective measurements of lung function) in two generations within both the paternal and maternal lines. Another major strength of the present analyses is the statistical approach used for assessing causal associations to estimate causal effects across generations. We used mediation models [30], which have become increasingly relevant for causal inference in epidemiological studies, in order to investigate complex pathways among multiple exposures, multiple mediators and multiple outcomes (MEMMMO framework) [22]. Finally, our results were supported by: 1) probabilistic simulations on the impact of unmeasured confounding [38]; 2) mediational E-values [39] to quantify robustness to unmeasured confounding, e.g. due to risk loci for both nicotine dependence and lung function [43, 44]; 3) a subgroup analysis to strengthen the causal interpretation of the observed effects of fathers’ smoking in prepuberty on lung function in the next generation; and 4) a sensitivity analysis based on offspring's post-bronchodilator spirometry, which is required to identify irreversible airflow obstruction [2].
There are a number of limitations of our analyses. First, the main limitation is that parental information from the ECRHS study was available for one parent only. The information on mothers and paternal grandparents was offspring- and father-reported, respectively, rather than directly assessed, generating a possible information bias. However, validation studies from our cohorts on tobacco smoking [64] and asthma [65] reports across generations suggested that, although recall bias is present, it is likely to have a limited effect. Second, post-bronchodilator spirometry was not used in the main analyses because it was only available for a subset of the ECRHS fathers. Third, the number of exposed subjects was small. Due to this data sparseness, we could not assess the moderating effects of offspring's sex [66] and mothers’ smoking before or after offspring's birth [5] (i.e. specific patterns of effects where grandmothers’ and fathers’ risk factors differently affect offspring according to their sex or their exposure to mothers’ smoking). Lastly, it is possible that unmeasured confounders (such as genetic, other lifestyle and environmental factors in the three generations) may be important. In particular, we could not control for the potential confounding effect of genetic factors in our models because polymorphisms were available for a subset of the fathers only, whereas genotypes on grandmothers, grandfathers, mothers and offspring are also needed to separate the influence of genetic inheritance from the effect of lifestyle/environmental exposures [67]. However, we estimated that unmeasured confounding had a limited impact on the effects identified in our analyses.
Conclusions
Men who initiated smoking before 15 years of age may have offspring with lower lung function compared with men who had never smoked. Grandmothers’ smoking in pregnancy may have a negative impact on their sons’ lung function, an effect that could be carried over to their grandchildren. These results support the concept that lifestyle-related exposures in male prepuberty and in pregnancy influence the health of future generations. Preventing smoking in these susceptibility time windows might have potential benefits for several generations.
Supplementary material
Please note: supplementary material is not edited by the Editorial Office, and is uploaded as it has been supplied by the author.
Supplementary material ERJ-02791-2020.SUPPLEMENT
Shareable PDF
Acknowledgements
The present analyses are part of the Research Council of Norway FRIPRO project (led by C. Svanes) and the ALEC Study (www.alecstudy.org; led by D. Jarvis). The present manuscript contributes to ALEC Workpackage 2 (led by C. Svanes). The RHINESSA Principal Investigators (PIs)/vice-PIs and ECRHS PIs/team members are listed in the supplementary material.
Footnotes
This article has supplementary material available from erj.ersjournals.com
Author contributions: All authors conceived and designed the study. A. Johannessen, B. Benediktsdóttir, R.J. Bertelsen, L. Bråbäck, S.C. Dharmage, B. Forsberg, F. Gómez Real, M. Holm, C. Janson, N.O. Jõgi, R. Jõgi, A. Malinovschi, J. Martínez-Moratalla Rovira, J.L. Sánchez-Ramos, V. Schlünssen, K. Torén and C. Svanes collected the data. S. Accordini and L. Calciano performed the statistical analyses. S. Accordini, L. Calciano, D. Jarvis and C. Svanes wrote the first version of the manuscript. All authors commented on results and contributed to the manuscript.
Conflict of interest: S. Accordini has nothing to disclose.
Conflict of interest: L. Calciano has nothing to disclose.
Conflict of interest: A. Johannessen has nothing to disclose.
Conflict of interest: B. Benediktsdóttir has nothing to disclose.
Conflict of interest: R.J. Bertelsen has nothing to disclose.
Conflict of interest: L. Bråbäck has nothing to disclose.
Conflict of interest: S.C. Dharmage has nothing to disclose.
Conflict of interest: B. Forsberg has nothing to disclose.
Conflict of interest: F. Gómez Real has nothing to disclose.
Conflict of interest: J.W. Holloway reports grants from the European Union's Horizon 2020 research and innovation programme, and from the Research Council of Norway, during the conduct of the study.
Conflict of interest: M. Holm has nothing to disclose.
Conflict of interest: C. Janson has nothing to disclose.
Conflict of interest: N.O. Jõgi has nothing to disclose.
Conflict of interest: R. Jõgi reports grants from the Estonian Research Council (personal research grant number 562), during the conduct of the study; personal fees for consultancy and lectures from GSK, Boehringer and Novartis, personal fees for travel/accommodation/meeting expenses from GSK and Boehringer, outside the submitted work.
Conflict of interest: A. Malinovschi has nothing to disclose.
Conflict of interest: A. Marcon has nothing to disclose.
Conflict of interest: J. Martínez-Moratalla Rovira has nothing to disclose.
Conflict of interest: J.L. Sánchez-Ramos has nothing to disclose.
Conflict of interest: V. Schlünssen has nothing to disclose.
Conflict of interest: K. Torén has nothing to disclose.
Conflict of interest: D. Jarvis reports grants from the European Union, during the conduct of the study.
Conflict of interest: C. Svanes has nothing to disclose.
Support statement: The ALEC Study received funding from the European Union's Horizon 2020 research and innovation programme (grant 633212). RHINESSA received funding from the Research Council of Norway (grants 214123, 228174 and 274767), the Bergen Medical Research Foundation, the Western Norwegian Regional Health Authorities (grants 911631, 911892 and 912011), the Norwegian Labour Inspection, the Norwegian Asthma and Allergy Association, the Danish Wood Foundation (grant 444508795), the Danish Working Environment Authority (grant 20150067134), the Swedish Lung Foundation, the Swedish Asthma and Allergy Association, and the Estonian Research Council (grant PUT562). Both the ALEC Study and RHINESSA received funding from the Australian National Health and Medical Research Council. The coordination of ECRHS was supported by the European Commission (ECRHS I and II) and the Medical Research Council (ECRHS III). Local funding agencies for ECRHS are reported in the supplementary material. The funders had no role in the study design, data collection, analysis and interpretation or writing of the report. The corresponding author had full access to all data and had final responsibility for the decision to submit for publication. Funding information for this article has been deposited with the Crossref Funder Registry.
References
Full text links
Read article at publisher's site: https://doi.org/10.1183/13993003.02791-2020
Read article for free, from open access legal sources, via Unpaywall:
https://erj.ersjournals.com/content/erj/58/4/2002791.full.pdf
Citations & impact
Impact metrics
Citations of article over time
Alternative metrics

Discover the attention surrounding your research
https://www.altmetric.com/details/103183288
Article citations
Maternal exposure to smoking and wheezing phenotypes in children: a cohort study of the Japan Environment and Children's Study.
BMC Pediatr, 24(1):624, 01 Oct 2024
Cited by: 0 articles | PMID: 39354379 | PMCID: PMC11443675
Multifaceted paternal exposures before conception and their epigenetic impact on offspring.
J Assist Reprod Genet, 04 Sep 2024
Cited by: 0 articles | PMID: 39230664
Review
To burn or not to burn: similar effects of different types of prenatal tobacco exposure on infant lung function.
ERJ Open Res, 10(4):294-2024, 08 Jul 2024
Cited by: 0 articles | PMID: 38978549 | PMCID: PMC11228605
Unveiling the hidden risk: paternal smoking and alcohol exposure prior to conception as independent factors for allergic rhinitis in children.
Front Pediatr, 12:1394400, 30 May 2024
Cited by: 0 articles | PMID: 38873584
ERS International Congress 2023: highlights from the Respiratory Infections Assembly.
ERJ Open Res, 10(3):880-2023, 13 May 2024
Cited by: 1 article | PMID: 38746858 | PMCID: PMC11089386
Go to all (20) article citations
Data
Data behind the article
This data has been text mined from the article, or deposited into data resources.
SNPs
- (1 citation) dbSNP - rs16969968
Similar Articles
To arrive at the top five similar articles we use a word-weighted algorithm to compare words from the Title and Abstract of each citation.
Parental Prepuberty Overweight and Offspring Lung Function.
Nutrients, 14(7):1506, 04 Apr 2022
Cited by: 4 articles | PMID: 35406119 | PMCID: PMC9002985
A three-generation study on the association of tobacco smoking with asthma.
Int J Epidemiol, 47(4):1106-1117, 01 Aug 2018
Cited by: 57 articles | PMID: 29534228 | PMCID: PMC6124624
Relationship between birth weight, maternal smoking during pregnancy and childhood and adolescent lung function: A path analysis.
Respir Med, 121:13-20, 19 Oct 2016
Cited by: 22 articles | PMID: 27888986 | PMCID: PMC5131806
Exposures during the prepuberty period and future offspring's health: evidence from human cohort studies†.
Biol Reprod, 105(3):667-680, 01 Sep 2021
Cited by: 5 articles | PMID: 34416759 | PMCID: PMC8444705
Review Free full text in Europe PMC
Funding
Funders who supported this work.
Horizon 2020 Framework Programme (1)
Grant ID: 633212