Abstract
Free full text

Activation of mammalian Chk1 during DNA replication arrest
Abstract
Checkpoints maintain order and fidelity in the cell cycle by blocking late-occurring events when earlier events are improperly executed. Here we describe evidence for the participation of Chk1 in an intra-S phase checkpoint in mammalian cells. We show that both Chk1 and Chk2 are phosphorylated and activated in a caffeine-sensitive signaling pathway during S phase, but only in response to replication blocks, not during normal S phase progression. Replication block–induced activation of Chk1 and Chk2 occurs normally in ataxia telangiectasia (AT) cells, which are deficient in the S phase response to ionizing radiation (IR). Resumption of synthesis after removal of replication blocks correlates with the inactivation of Chk1 but not Chk2. Using a selective small molecule inhibitor, cells lacking Chk1 function show a progressive change in the global pattern of replication origin firing in the absence of any DNA replication. Thus, Chk1 is apparently necessary for an intra-S phase checkpoint, ensuring that activation of late replication origins is blocked and arrested replication fork integrity is maintained when DNA synthesis is inhibited.
Introduction
In eukaryotic cells, fidelity of chromosome transmission is maintained by checkpoints that prevent cell cycle progression if chromosomes are damaged, remain unreplicated, or are incorrectly segregated. Checkpoints responding to DNA damage or incomplete DNA replication elicit one cellular response by inhibiting cyclin-dependent kinases that drive cell cycle progression (Nurse, 1997; Weinert, 1998). In yeasts, a group of six checkpoint proteins monitors the presence of DNA damage or incomplete DNA replication and signals to components of the cell cycle machinery (Weinert, 1998). Signaling elements of this pathway include Rad3 (Bentley et al., 1996), a phosphatidylinositol 3-kinase–like kinase, as well as downstream protein kinases Chk1 (Walworth et al., 1993) and Cds1 (Murakami and Okayama, 1995), which are believed to directly interact with effectors of cell cycle progression (Furnari et al., 1997; Boddy et al., 1998; Zeng et al., 1998).
These gene products have structural and functional equivalents in mammalian cells. Upstream signaling elements include the phosphatidylinositol 3-kinase family members ataxia telangiectasia mutated (ATM)* (Savitsky et al., 1995) and ATM- and Rad3-related protein (ATR) (Bentley et al., 1996; Cimprich et al., 1996). Mammalian Chk1 is a homologue of yeast Chk1 (Sanchez et al., 1997), whereas Chk2/hCds1 (Matsuoka et al., 1998; Brown et al., 1999; Chaturvedi et al., 1999) is a homologue of Schizosaccharomyces pombe Cds1 (S. cerevisiae Rad53). Chk1 and Chk2 act downstream of ATM and ATR to elicit appropriate responses such as cell cycle arrest (Peng et al., 1997; Sanchez et al., 1997; Matsuoka et al., 1998; Liu et al., 2000).
In eukaryotes, DNA replication is initiated from many origins (Cairns, 1966; Huberman and Riggs, 1968) that fire in precise temporal sequence throughout S phase (Diffley, 1998). In budding yeast, when replication is blocked, late-firing origins are prevented from initiating replication (Santocanale and Diffley, 1998; Shirahige et al., 1998) and entry into mitosis is inhibited (Weinert et al., 1994). These cell cycle blocks are mediated by Rad53 and Mec1 (Weinert et al., 1994; Santocanale and Diffley, 1998; Shirahige et al., 1998; Santocanale et al., 1999).
In mammalian cells, several S phase checkpoint responses have been described. Fusion of S phase HeLa cells with late G2 cells results in a delay in appearance of mitotic forms, suggesting that ongoing DNA synthesis generates a checkpoint signal (Rao and Johnson, 1970). DNA damage incurred in S phase causes inhibition of replication initiation and chain elongation (Painter and Young, 1980). Such inhibition is dramatically reduced in cells from AT patients, indicating a role for the ATM kinase in mediating this response (Painter and Young, 1980). The roles of ATR, Chk1, and Chk2 in mammalian S phase checkpoints are much less clear, in part because ATR (Brown and Baltimore, 2000; de Klein et al., 2000) and Chk1 (Liu et al., 2000) are essential genes.
In mammalian cells, the timing of origin firing may be examined by monitoring the dynamics of groups of coordinately replicated chromosomal domains. The origin firing program operates independently of checkpoint pathways but is sensitive to them nonetheless (Dimitrova and Gilbert, 2000). Whenever cells are prevented from completing synthesis from early replicons, a caffeine-sensitive, ATM-independent, intra-S phase checkpoint stabilizes components of existing replicons and prevents initiation of replication from late-firing origins (Dimitrova and Gilbert, 2000).
Here, we have investigated the properties of Chk1 and Chk2 during S phase in mammalian cells. We present data to suggest that Chk1 responds to stalled replication forks as a necessary component for an intra-S phase checkpoint, ensuring that activation of late replication origins is blocked when synthesis from early origins is inhibited.
Results
Specific S phase activation of Chk1 in mammalian cells
Anti-Chk1 antibodies specifically recognized a single protein in HeLa (Fig. 1 A) and other mammalian cell lysates (unpublished data), which migrated with an apparent M r of ~58 kD, as expected (Sanchez et al., 1997). Anti-Chk1 immunoprecipitates (IPs) contained kinase activity that efficiently phosphorylated a peptide derived from Cdc25C (Fig. 1 B) and containing Ser216, which is phosphorylated in vivo and in vitro by Chk1 (Peng et al., 1997; Sanchez et al., 1997). IP kinase activity was completely inhibited by submicromolar concentrations of UCN-01 (Fig. 1 B), shown previously to be a selective small molecule inhibitor of Chk1 (Graves et al., 2000).
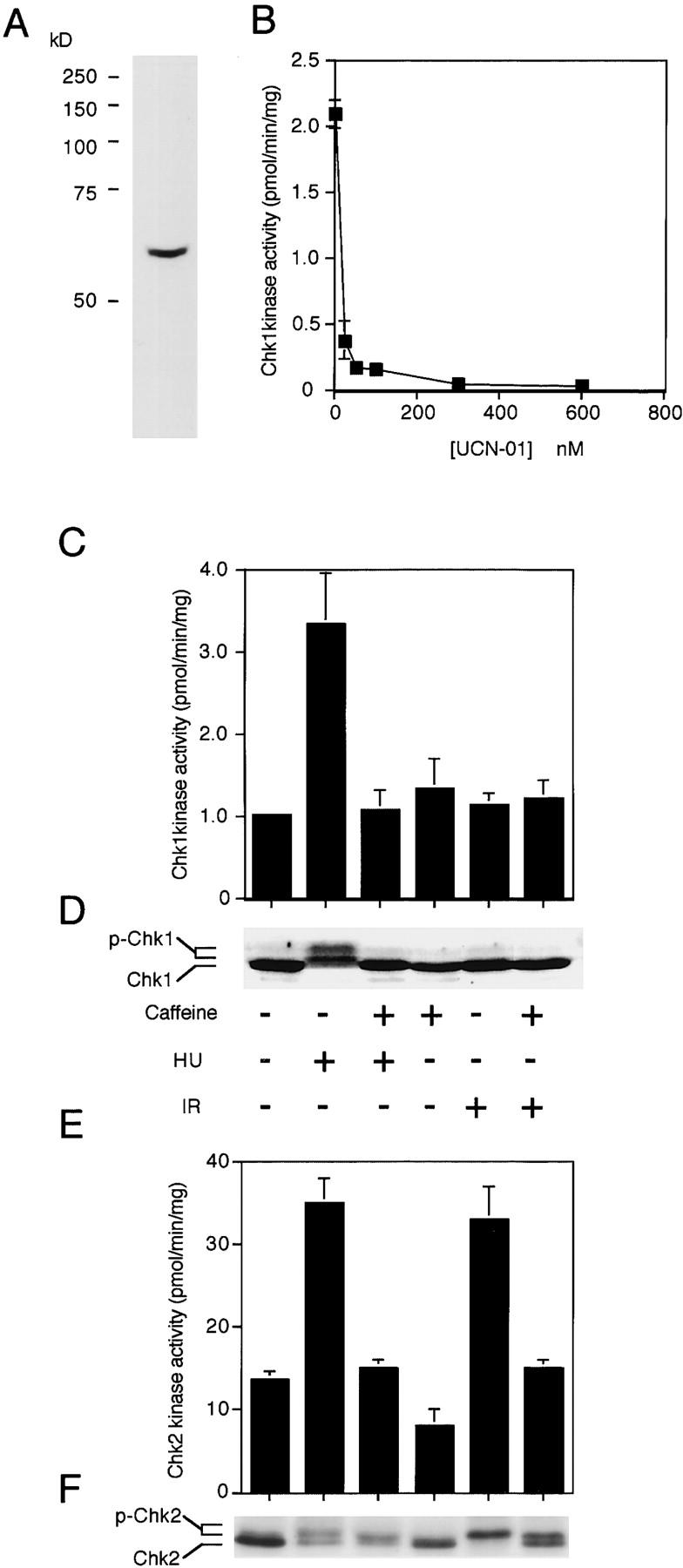
Chk1 and Chk2 activation in response to HU and IR. (A) HeLa cell lysate (50 μg) subjected to SDS-PAGE and immunoblotted with anti-Chk1 antibodies. (B) Kinase assays in the presence of indicated concentrations of UCN-01 performed on Chk1 IPs from HeLa cell lysates. (C) HeLa cells ± caffeine (5 mM), were treated with hydroxyurea (+HU, 2 mM) or irradiated (10 Gy, +IR) as indicated. Cells were harvested, lysed and Chk1 IP kinase assays performed. (D) Lysates from cells treated as in C were subjected to SDS-PAGE and immunoblotted for Chk1. (E) Kinase assays performed on Chk2 IPs from lysates obtained as in C. (F) Immunoblot analysis of Chk2 following treatments indicated in E.
Next, we compared kinase activity of Chk1 and Chk2 in IPs from lysates of cells treated either with hydroxyurea (HU), which arrests cells in or at the start of S phase, or with 10 Gy of ionizing radiation (IR), which arrests cells in G2. Chk1 was specifically activated 3.5–4-fold in cells arrested in S phase compared with asynchronous cell controls (Fig. 1 C). No significant activation was observed in response to IR. Activation was accompanied by conversion of Chk1 to a number of forms migrating with reduced mobility on SDS-PAGE (Fig. 1 D). Both activation and mobility shift of Chk1 were reversed by protein phosphatase 2A treatment (unpublished data), indicating that both were due to phosphorylation. The absence of Chk1 activation after IR was not due to an absence of a response in these cells to IR, because this treatment gave rise to robust phosphorylation and activation of Chk2 kinase (Fig. 1, E and F, and unpublished data), which was also activated by HU treatment as expected (Matsuoka et al., 1998; Chaturvedi et al., 1999).
Several checkpoints are sensitive to caffeine, resulting in inappropriate cell cycle progression (Busse et al., 1978; Schlegel and Pardee, 1986). Likely in vivo targets of caffeine are ATM (Blasina et al., 1999) and ATR (Hall-Jackson et al., 1999). Caffeine completely abrogated phosphorylation and activation of Chk1 induced by S phase arrest (Fig. 1, C and D), as well as activation of Chk2 in response to HU or IR (Fig. 1, E and F).
S phase activation of checkpoint proteins Chk1 and Chk2 does not require ATM
Cells derived from patients with AT are defective in some (Houldsworth and Lavin, 1980; Painter and Young, 1980), but not all (Dimitrova and Gilbert, 2000), S phase checkpoints. We utilized ATM-null cells (AT221JE-T) transfected either with empty vector or vector encoding wild-type ATM (Ziv et al., 1997) to determine whether S phase activation of Chk1, as well as Chk2, required ATM. Both Chk1 (Fig. 2, A and B) and Chk2 (Fig. 2, C and D) were phosphorylated and activated in response to HU, to levels very similar to those observed with other cell types. Reintroduction of wild-type ATM had little or no effect on the extent of activation in response to HU (unpublished data). As expected, treating ATM-null cells with 10 Gy of IR had no effect on either Chk1 or Chk2 activity (Matsuoka et al., 1998). Surprisingly, we also found that reintroduction of ATM had a very small effect on the phosphorylation and activation of Chk1 (Fig. 2, E and F) compared with the response to HU. Cells expressing ATM had slightly higher basal levels of Chk1 activity compared with ATM-null cells, and IR treatment in the presence of ATM led to hyperphosphorylation of a very small fraction of Chk1 in these cells (Fig. 2 F). This was not because these cells are refractory to IR under these conditions, as reintroduction of ATM reestablished the phosphorylation and activation of Chk2 after IR (Fig. 2, G and H).
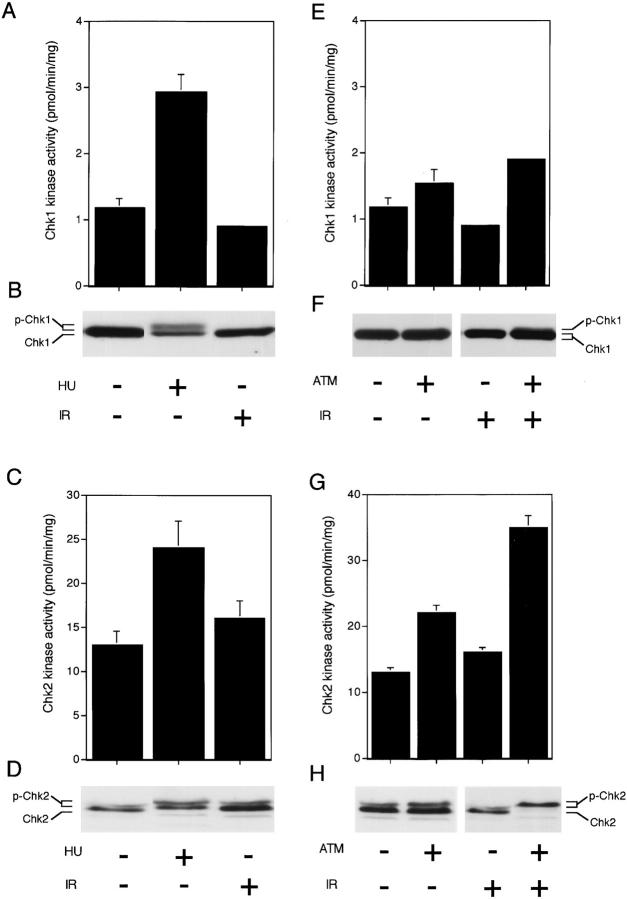
S phase activation of Chk1 and Chk2 is independent of ATM. (A–D) ATM-null (AT221JE-T) cells were untreated, treated with HU (+HU, 2 mM) or irradiated (10 Gy, +IR) as indicated. (A) Cells were lysed, and kinase assays performed on Chk1 IPs. (B) Lysates subjected to SDS-PAGE and immunoblotted for Chk1. (C) Kinase assays performed on Chk2 IPs. (D) Lysates subjected to SDS-PAGE and immunoblotted for Chk2. (E-H) AT221JE-T cells containing either vector alone (−ATM) or vector expressing ATM (+ATM) were irradiated (10 Gy, +IR) or not as indicated, and lysates prepared. (E) Kinase assays performed on immunoprecipitated Chk1. (F) Lysates subjected to SDS-PAGE and immunoblotted for Chk1. (G) Kinase assays were performed on immunoprecipitated Chk2. (H) Lysates subjected to SDS-PAGE and immunoblotted for Chk2.
These results indicate that both Chk1 and Chk2 are activated in S phase cells in a caffeine-sensitive manner that does not require ATM. They also show that although Chk1 has been implicated in the G2 checkpoint responding to IR, the fraction of Chk1 phosphorylated and activated is substantially less than that observed for these cells in S phase. This suggests that in addition to a role in G2 arrest in response to IR, Chk1 plays some role in S phase checkpoint responses in mammalian cells.
Arrest of DNA replication is required for S phase activation of Chk1 and Chk2
To test whether activation of Chk1 and Chk2 occurred during normal S phase progression, we synchronized HeLa cells in metaphase using nocodazole and mitotic shake-off. Mitotic cells were released into fresh medium in the presence or absence of HU. Cells were pulse labeled with BrdU 0.5 h before harvest, and then processed either for analysis by flow cytometry or for lysis and characterization of Chk1 and Chk2 proteins by IP kinase assay and immunoblot.
In the absence of HU, synchronized cells underwent normal progression through S phase over an ~10-h time period, with 24% cells emerging in G2 21 h after release from the nocodazole block (Fig. 3, A and B) . In contrast, cells in HU-containing medium entered S phase as judged by pulsed BrdU incorporation (unpublished data), but remained arrested with a G1 DNA content for the duration of the experiment (Fig. 3, C and D). Kinase activity together with the phosphorylation status of both Chk1 and Chk2 were analyzed every 2 h from 9 to 21 h after release from nocodazole block. No activation or phosphorylation of Chk1 was observed during normal S phase progression, despite the fact that 70–80% of the cells were in S phase 13–18 h after release (Fig. 3, E and F). In contrast, cells arrested in early S phase with HU had 2.5–5-fold higher levels of Chk1 kinase activity (black bars) compared with those undergoing normal S phase (white bars). Activation was accompanied by a mobility shift of Chk1 on analysis by SDS-PAGE and immunoblotting. A similar pattern was observed with Chk2, although Chk2 activation on S phase arrest was more modest than with Chk1 (Fig. 3, G and H).

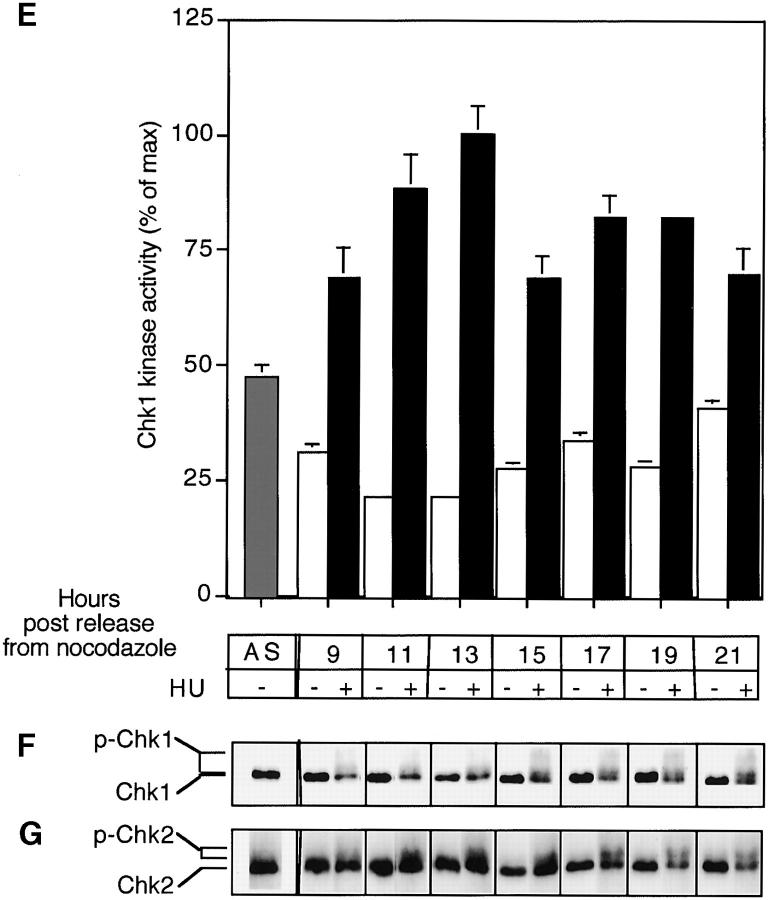
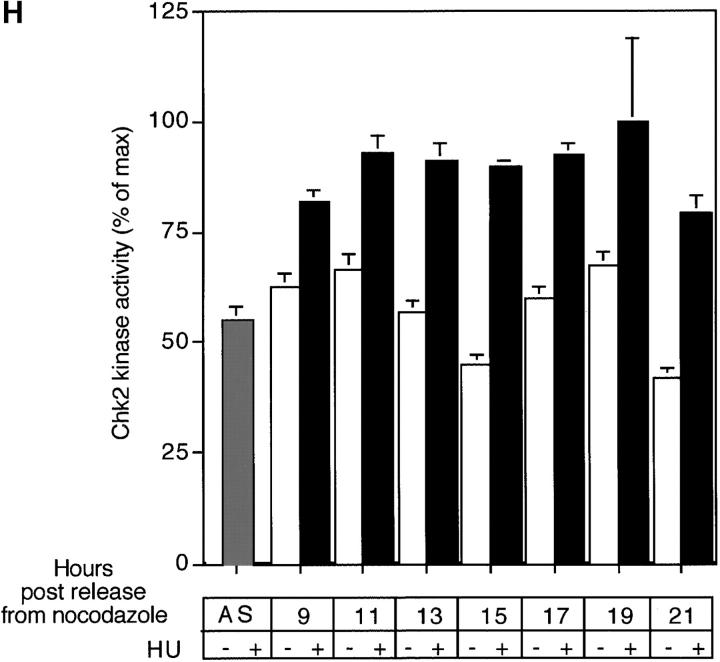
Activation of Chk1 and Chk2 requires DNA replication arrest. Metaphase HeLa cells collected by nocodazole treatment and mitotic shake-off were released into fresh medium +/−HU. 0.5 h before harvest, at indicated times after nocodazole release, cells were pulse labeled with BrdU, and aliquots removed for flow cytometry (A–D), lysis and IP kinase assays (E and H) and immunoblotting (F and G). (A–D) FACS® profiles and cell cycle position are shown for cells maintained in the absence (-HU, A and B) and presence (+HU, C and D) of HU (2 mM). (E) Chk1 IP kinase assays performed on lysates from asynchronous cycling cells (AS, grey bars), cells progressing through S phase (white bars) and arrested in early S phase (black bars). (F) Cell lysates as in E above subjected to SDS-PAGE and immunoblotted for Chk1. (G) Cell lysates as in E above subjected to SDS-PAGE and immunoblotted for Chk2. (H) Chk2 IP kinase assays performed on lysates from cells as in E.
Distinct checkpoint kinase responses following reversal of S phase arrest
Treating cells with aphidicolin, which stalls replication forks by directly inhibiting DNA polymerases, resulted in mobility shifts and activation of Chk1 and Chk2 identical to those observed in cells treated with HU (Fig. 4 A), which stalls replication forks by depleting dNTP pools. To confirm that activation of Chk1 and Chk2 occurs in response to stalled replication forks, we allowed HU-treated AT221JE-T cells to recover from replication arrest and measured Chk1 and Chk2 kinase activity as a function of S phase progression. As judged by BrdU incorporation, >70% of cells released from HU-containing medium resumed significant synthesis within 2 h (Fig. 4 B). Release from HU resulted in rapid (~0.5 h) inactivation of Chk1, as judged by decreased activity toward its peptide substrate and higher electrophoretic mobility (Fig. 4, C and D, upper panels). In contrast, the activity and mobility of Chk2 remained unchanged as cells initially arrested in HU resumed S phase progression (Fig. 4, C and D, lower panels). Identical results were also obtained using aphidicolin (unpublished data). In addition, sustained activity of Chk2 following recovery from replication block occurred in both ATM-null cells and AT cells retransfected with wild-type ATM. These results indicate that Chk1, but not Chk2, becomes inactivated, with kinetics indicative of a link between Chk1 activation and stabilization of stalled replication complexes together with prevention of new replicon initiation.
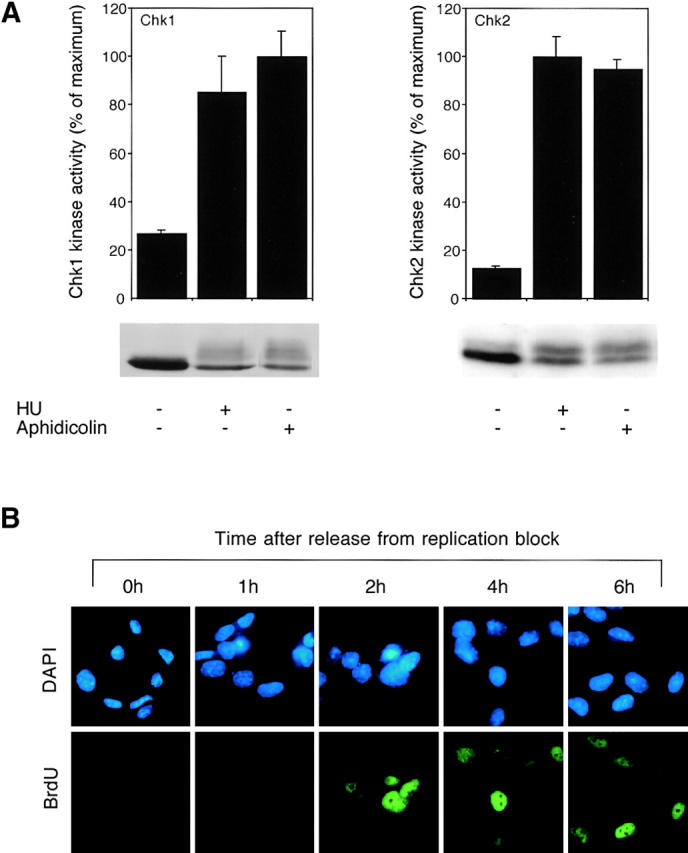
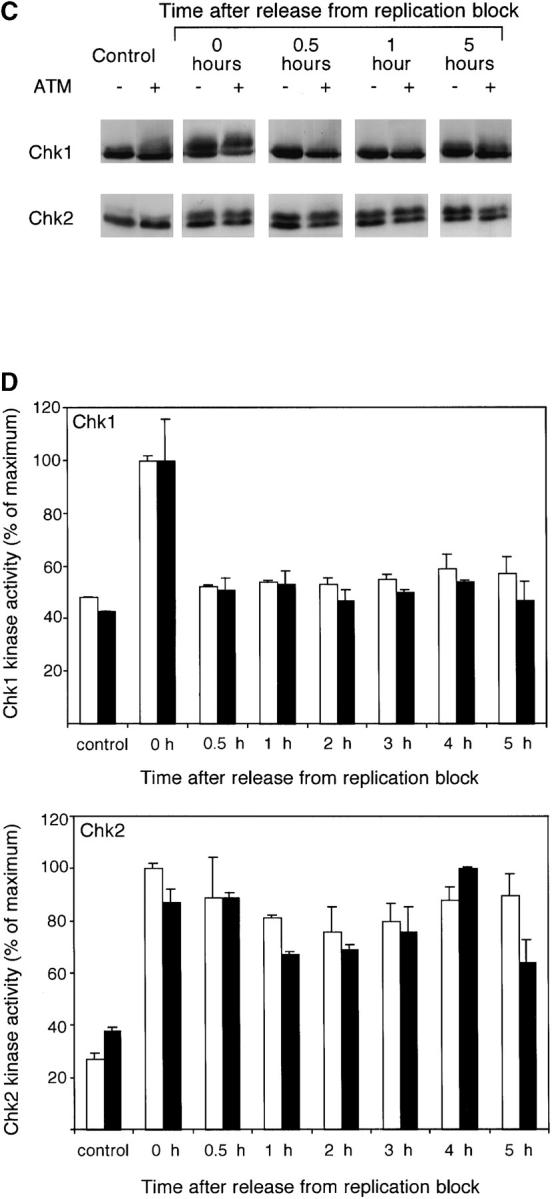
Differential inactivation of Chk1 and Chk2 following release from replication block. (A) Asynchronously growing HeLa cells were treated or not for 24 h with 2 mM HU or 5 μg/ml aphidicolin and lysates prepared. Kinase assays were performed on immunoprecipitated Chk1 and Chk2 (upper panels) as indicated. Lysates were subjected to SDS-PAGE and immunoblotted for Chk1 and Chk2 (lower panels). (B) Asynchronously growing AT221JE-T cells expressing ATM were treated with 2 mM HU for 24 h, released, and at times indicated pulse labeled with BrdU. Cells were fixed, stained with DAPI and anti-BrdU antibodies, and examined by indirect immunofluorescence microscopy. Identical results were observed with AT cells transfected with empty vector (not shown). (C and D) AT221JE-T cells containing either vector alone (−ATM) or vector expressing ATM (+ATM) were treated for 24 h with HU, and then released into fresh medium. (C) At the times indicated, lysates were prepared, subjected to SDS-PAGE, and immunoblotted for Chk1 and Chk2. (D) At times indicated, Chk1 (upper panels) and Chk2 (lower panels) IP kinase assays were performed on lysates from AT221JE-T cells containing vector alone (black bars) or vector expressing ATM (white bars) treated as above.
Selective abrogation of an S phase checkpoint signaling pathway
The response of Chk1 to the imposition and removal of replication blocks suggested that it might play a role in the intra-S phase checkpoint that stabilizes components of existing replicons and prevents initiation of replication from late-firing origins (Dimitrova and Gilbert, 2000). As Chk1 is an essential gene in mammals (Liu et al., 2000), we utilized UCN-01 which, at submicromolar levels, is highly selective for Chk1 over Chk2 (Graves et al., 2000), to determine the effect of abrogating Chk1 function. UCN-01 has been used to demonstrate a critical role for Chk1 in the IR-induced G2 checkpoint (Graves et al., 2000), as well as the UV-induced G1/S checkpoint (Mailand et al., 2000).
When active, mammalian Chk1 undergoes autophosphorylation at multiple sites (Chen et al., 2000; unpublished data). In yeast, Chk1 appears to undergo significant autophosphorylation after activation by Rad3 (Walworth and Bernards, 1996). UCN-01 blocked Chk1 autophosphorylation in vitro (Fig. 5 A) and substantially reduced but did not eliminate the S phase arrest–induced hyperphosphorylation of Chk1 in vivo (Fig. 5, B and E), indicating that UCN-01 is capable of interfering with the Chk1 signaling pathway. In contrast, a control protein kinase inhibitor, K-252c, had no effect on Chk1 hyperphosphorylation in vivo (unpublished data). These results suggested that, as in yeast, some of the hyperphosphorylation of Chk1 in mammalian cells in vivo is due to autophosphorylation, which may be blocked by treating cells with UCN-01. To show that UCN-01 was indeed acting on Chk1 and not by interfering with a known upstream activator, ATR (Liu et al., 2000) we investigated the effect of UCN-01 on ATR-mediated phosphorylation of Chk1 in vitro. UCN-01 had no effect on phosphorylation of Chk1 by ATR (Fig. 5 D). ATR is sensitive to inhibition by caffeine (Hall-Jackson et al., 1999). If UCN-01 does indeed block Chk1 autophosphorylation but not phosphorylation of Chk1 by its upstream activator, then lysates from HU-arrested cells treated with UCN-01 should contain forms of Chk1 with reduced mobility compared with HU-arrested cells treated with caffeine. We found that caffeine completely blocked the mobility shift of Chk1 in response to HU-induced replication block. In contrast, Chk1 from UCN-01–treated cells in HU had a reduced mobility, though not to the extent observed in cells treated with HU alone (Fig. 5 E). To determine whether UCN-01 had any effect on HU-induced Chk2 activation, lysates from cells treated with HU +/−UCN-01 were analyzed for Chk2. UCN-01 had no effect on either the phosphorylation or activation of Chk2 in response to HU treatment (Fig. 5 F).
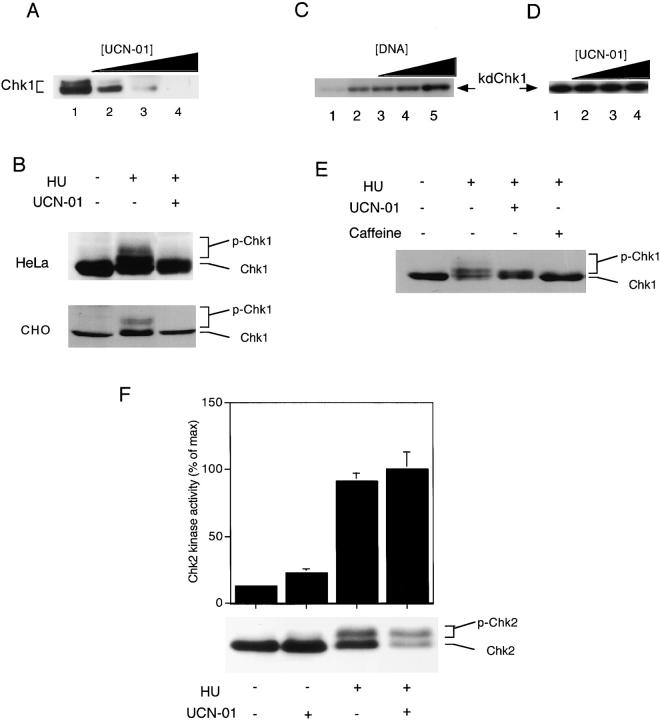
UCN-01 blocks Chk1 autophosphorylation in vitro and Chk1 hyperphosphorylation in vivo, with no effect on ATR kinase in vitro or S phase activation of Chk2 in vivo. (A) Chk1 autophosphorylation was measured by incorporation of 32P from labeled ATP in the presence of 0 (lane 1), 10 nM (lane 2) 50 nM (lane 3), and 300 nM UCN-01 (lane 4) followed by SDS-PAGE and autoradiography. (B) HeLa and CHO cells were treated +/− HU (2 mM, 15 h) in the presence or absence of 300 nM UCN-01 and lysates analyzed for Chk1 by SDS-PAGE and immunoblotting. Immunoblot is overexposed to show all reduced mobility forms of Chk1 on HU treatment. (C) SDS-PAGE and autoradiography of kinase-dead Chk1 following its phosphorylation by mock (lane 1), purified ATR in the presence of 0 (lane 2), 0.5 ng/μl (lane 3), 2 ng/μl (lane 4), and 4 ng/μl DNA (lane 5) and (D) DNA-dependent phosphorylation of kinase-dead Chk1 by purified ATR in the presence of 0 (lane 1), 10 nM (lane 2) 50 nM (lane 3), and 300 nM UCN-01 (lane 4). (E) HeLa cells treated +/−HU as above in the presence or absence of 300 nM UCN-01 or 5 mM caffeine, and lysates analyzed for Chk1 by SDS-PAGE and immunoblotting. Samples in lanes 1–3 are identical to upper panel of B but at lower exposure to resolve distinct forms of Chk1 after indicated treatments. (F) HeLa cells treated +/− HU as above in presence or absence of 300 nM UCN-01 as indicated, were lysed and Chk2 analysed by IP kinase assay (upper panel) or by SDS-PAGE and immunoblotting (lower panel).
Taken together, the data in Fig. 5 indicate that in mammalian cells, replication arrest activates a caffeine-sensitive pathway resulting in the phosphorylation and activation of Chk1 and Chk2. UCN-01 acts downstream of caffeine, blocking Chk1 function without interfering with the Chk2 pathway.
Inhibition of Chk1 function in aphidicolin-arrested cells results in initiation at late DNA replication origins
Eukaryotic chromosomes replicate from many origins programmed to initiate replication in a precise temporal sequence throughout S phase (Gilbert, 1986; Diffley, 1998; Ma et al., 1998). Whenever cells are prevented from completing synthesis from early replicons, late-firing origins are prevented from initiating replication (Hamlin, 1978; Santocanale and Diffley, 1998). DNA replication takes place at discrete sites that may be visualized by pulse-labeling cells with halogenated derivatives of deoxyuridine (dU) and staining with fluorescently labeled antibodies specific to each dU derivative. The spatial pattern of replication sites reveals their temporal position within S phase, allowing the dynamics of groups of coordinately replicated chromosomal domains to be monitored (Dimitrova and Gilbert, 2000).
To determine whether abrogation of Chk1 function in cells arrested in S phase would allow the initiation of replication at later-replicating sites, asynchronously growing cells were briefly pulse labeled with CldU and then treated with aphidicolin for 12 h. In the continued presence of aphidicolin, cells were incubated +/−UCN-01 for the indicated times. Cells were washed free of both aphidicolin and inhibitor, pulse labeled with IdU, and fixed and stained with anti-CldU (green) and anti-IdU (red) antibodies. Without UCN-01 addition, IdU labeling colocalized with CldU throughout the 12-h incubation period, indicating that there was no progression through S phase or initiation within later-replicating domains under these conditions (Fig. 6 A, +Aphidicolin). In contrast, when Chk1 function was abrogated during the aphidicolin block by the addition of UCN-01 for increasing lengths of time, the IdU label was incorporated into progressively later-replicating domains, and was accompanied by a lack of DNA synthesis from previously initiated sites. Thus, when UCN-01 was present for only 30 min during the aphidicolin block, the majority of signal for the two labels colocalized giving rise to mainly yellow foci (Fig. 6 A). However, with longer times in UCN-01 the two labels began to separate. Cells that were in early S phase at the time of the CldU pulse (identified by the presence of many sites of CldU incorporation distributed throughout euchromatic compartments of the nucleus) initiated replication at later-replicating euchromatic sites within 3 to 6 h and then progressed to middle-replicating sites by 6 to 9 h, and finally late-replicating heterochromatin by 12 h after the addition of UCN-01 (Fig. 6 A, upper panels). Cells that were in mid S phase during the CldU pulse (identified by peripheral and peri-nucleolar CldU staining) incorporated IdU into late-replicating heterochromatin within 6 to 9 h and ceased to incorporate IdU after 12 h (Fig. 6 A, middle panels). Cells that were in late S phase during the CldU pulse (identified by few very large sites of CldU incorporation) progressed into G2 phase within 3 to 6 h (Fig. 6 A, lower panels). In cells that entered G2 in the absence of DNA synthesis, nuclei underwent blebbing and fragmentation (unpublished data), possibly indicating an apoptotic response triggered by entry of cells into G2/M phase in the presence of an unreplicated genome, as reported previously (Dimitrova and Gilbert, 2000).
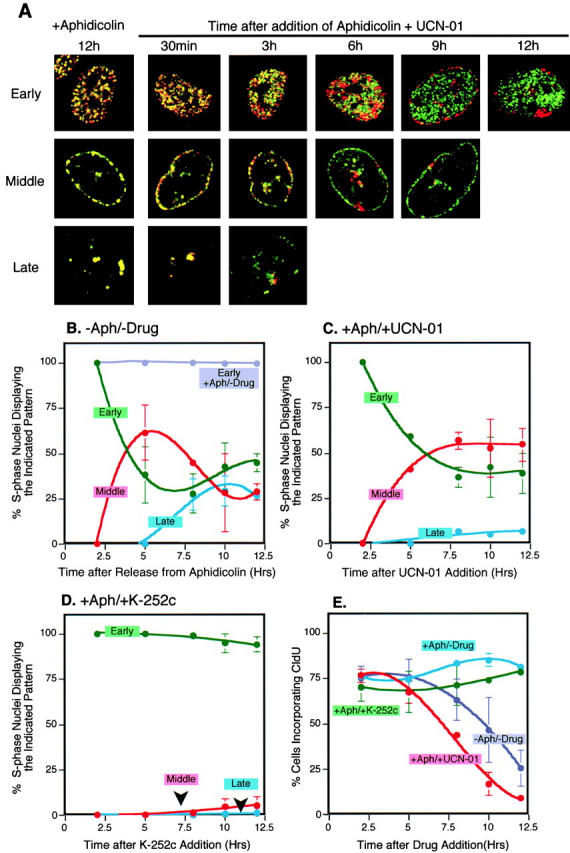
UCN-01 inhibits replication checkpoint controlling replicon firing. (A) Asynchronous CHOC cells were pulse labeled with CldU and then treated with aphidicolin for 12 h either in the absence (extreme left-hand panels, +Aphidicolin) or in the presence of 300 nM UCN-01. At time points after aphidicolin addition (0.5, 3, 6, 9, 12 h), aliquots of cells were washed free of drugs, pulse labeled with IdU and then fixed and stained with anti-CldU (green) and anti-IdU (red). Images show typical nuclei observed at indicated time points in cells that were prelabeled early, middle, or late in S phase. Blank panels indicate time points at which IdU incorporation into cells with the indicated CldU pattern were no longer detected, as these cells have progressed into G2-phase. (B–E) CHOC cells were synchronized at the G1/S boundary, and then divided into groups treated in parallel as follows. (B) Cells were either washed free of aphidicolin (colored circles) and allowed to proceed into S phase in the complete absence of drugs, or as controls, cells were maintained throughout the experiment in aphidicolin alone (B, grey circles). (C and D) Cells were maintained in the continuous presence of aphidicolin in combination with either (C) UCN-01 or (D) K-252c. At the indicated times, all cells were washed into fresh drug-free medium, pulse labeled with CldU, and then fixed and stained with anti-CldU. The percentages of S phase (CldU-positive) nuclei showing the indicated replication pattern were scored at each time point. In addition, percentages of cells in B–D that incorporated CldU were also scored (E).
To determine the time schedule of activation of progressively later-replicating foci in cells lacking Chk1 function, mitotic cells previously labeled at late-replicating sites with IdU were cultured in fresh medium containing aphidicolin to collect cells in early S phase. Then, in the continued presence of aphidicolin, UCN-01, K-252c, or no additional drug was added. Control cultures were incubated without any drug (i.e., aphidicolin removed). At indicated times thereafter, cells were washed free of all drugs, pulse labeled with CldU, and then fixed and stained with anti-CldU antibodies. The spatial pattern of anti-CldU staining (Fig. 6, B–D), as well as the percentage of cells stained with CldU (Fig. 6 E) was scored. Control cells released from aphidicolin proceeded through a normal S phase (Fig. 6 B). When cells were incubated in the continued presence of both aphidicolin and UCN-01, they proceeded through the various patterns of S phase in the absence of DNA synthesis (Fig. 6 C). In contrast, cells incubated in the continued presence of aphidicolin and the control K-252c (Fig. 6 D) or in aphidicolin alone (Fig. 6 B, +Aphidicolin/−Drug) remained poised in early S phase.
To see whether Chk1 regulates the replication timing program in cells transiting a normal S phase, we established the effect of UCN-01 on the timing of progressively later replicating foci in cells in the absence of aphidicolin. Asynchronous CHOC cell cultures were pulse labeled with CldU and then either treated or not treated with UCN-01. At indicated times after CldU labeling, cells were washed free of drugs, pulse labeled with IdU, and then fixed and stained for CldU and IdU. At each time point, the percentage of nuclei that proceeded from early CldU-labeled replication patterns to either early, middle, or late IdU replication patterns during the chase time was scored (Fig. 7) . Progression through the various patterns of S phase was virtually identical in cells treated with UCN-01 compared with those not exposed to the drug. Similarly, in cells synchronized at G1/S with aphidicolin and released, the presence of UCN-01 did not advance the timing of the appearance of late patterns (unpublished). Taking the data in Figs. 6 and and77 together, we conclude that, like 2-aminopurine (2-AP) and caffeine (Dimitrova and Gilbert, 2000), UCN-01, a selective inhibitor of Chk1, abrogates the intra-S phase checkpoint allowing cells to initiate further replication and progress through the cell cycle in the absence of DNA replication. In addition, on release from aphidicolin arrest, cells treated with UCN-01 are unable to elongate from previously arrested replication forks, indicating that this checkpoint also maintains the integrity of arrested replication forks as shown previously for caffeine and 2-AP (Dimitrova and Gilbert, 2000). However, abrogation of Chk1 does not affect the timing of initiation during normal S phase progression.
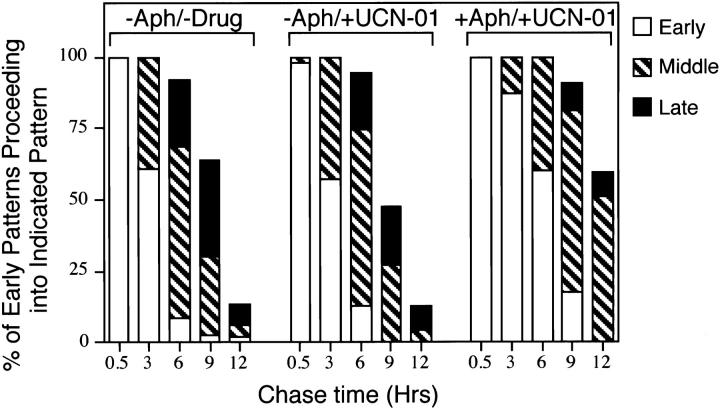
UCN-01 does not affect the timing of normal S phase progression. Three asynchronous CHOC cell cultures were pulse labeled for 10 min with CldU. Two of these were then chased +/−UCN-01. A third culture was incubated in parallel in the presence of aphidicolin and UCN-01. At indicated times after CldU labeling, aliquots of cells from each were washed free of drugs, pulse labeled with IdU for 20 min, and then fixed and stained for CldU and IdU. At each time point (minimum of 100 CldU-labeled nuclei counted), the percentage of nuclei that proceeded from early CldU-labeled replication patterns to either early, middle, or late IdU replication patterns during the chase time was scored. The percentage of cells that label with IdU decreases as progressively more CldU-labeled cells complete S phase and enter G2. Results are representative of two independent experiments.
Discussion
Here, we present evidence that, in mammalian cells, Chk1 protein kinase plays a role in an intra-S phase checkpoint controlling late origin firing, which is activated by stalled but not dynamic replication forks.
Significant phosphorylation and activation of Chk1 occurred when HeLa cells were treated with HU but not with IR, suggesting an important role for Chk1 specifically in signaling S phase events. In principle, it was conceivable that Chk1 (and Chk2) might be phosphorylated and activated in an unperturbed S phase and thus the hyperphosphorylation and activation following HU treatment would be a consequence of cells accumulating in S phase. However, we found that in synchronized cycling cells, neither Chk1 nor Chk2 was phosphorylated or activated during normal S phase progression, indicating that activation was not due to the accumulation of S phase cells, but rather a response to replicational stress.
Fusion of S phase cells to late G2 cells has been shown to delay the appearance of mitotic forms (Rao and Johnson, 1970), which led to the suggestion that ongoing DNA synthesis generates a continuous intrinsic checkpoint signal to prevent inappropriate entry into mitosis (Dasso and Newport, 1990). The data presented here indicate that phosphorylation and activation of the checkpoint proteins Chk1 and Chk2 are unlikely to be responsible for signaling such an intrinsic checkpoint. In addition, they strongly suggest that activation of these checkpoint elements in mammalian cells occurs in response to stalled or slowed, but not active, replication forks. We cannot exclude the possibility that some other aspect of checkpoint kinase function, not detected in these studies, participates in signaling an unperturbed S phase. For example, it remains possible that these kinases are activated at very specific times in S phase or that small populations are activated locally. One alternative explanation is that the presence of G2/M cell cytoplasm in an S phase cell (Rao and Johnson, 1970) causes replication fork slowing or stalling, which in turn generates a checkpoint signal.
Chk2 and Chk1 respond differently to DNA structures that are generated by replication arrest. After release from replication blocks, Chk1 became rapidly inactivated and inactivation preceded bulk resumption of DNA synthesis. This is consistent with the notion that Chk1 activation state is closely linked to the status of the bulk of cellular replication forks. However, this is not true for Chk2, which once activated by replication block, remained so for many hours in cells released into drug-free media, which resumed and executed S phase. One possibility is that Chk1 and Chk2 differ in their sensitivity to DNA structures generated by S phase inhibitors, and Chk2 activation reflects the cellular response to a fraction of forks which have not been, or cannot be, reactivated. Alternatively, Chk2 may respond to a subset of discrete, aberrant structures, that are insufficient to induce Chk1 activation. Whatever the nature of the structure(s) that activates Chk2, it does not significantly affect bulk DNA synthesis, and activation of Chk2 is not sufficient to block S phase progression. Prolonged activation of Chk2 after synchronization may also underlie the ability of synchronized S phase nuclei to delay M-phase onset (Rao and Johnson, 1970).
DNA damage incurred in replicating cells results in a checkpoint-mediated suppression of DNA synthesis. ATM-deficient cells fail to operate this intra-S phase checkpoint and carry out significant replication (radio-resistant DNA synthesis, RDS) even in the presence of DNA damage (Houldsworth and Lavin, 1980; Painter and Young, 1980). An additional intra-S phase response is concerned with replication fork arrest. This is modulated by a caffeine-sensitive, ATM-independent checkpoint that operates under conditions of replicational stress, but not during normal S phase progression (Dimitrova and Gilbert, 2000). The existence of two S phase checkpoint responses led us to determine whether the HU-induced activation of Chk1 and Chk2 was dependent on ATM. We found that Chk1 and Chk2 activation was retained in ATM-deficient cells treated with HU, and reintroduction of ATM had no significant effect on the HU-induced response (unpublished data). Overexpression of Chk1 in ATM-deficient cells restores the G2 checkpoint but has no effect on suppressing RDS (Chen et al., 1999). Taken together, these data indicate that HU-induced activation of Chk1 and Chk2 represents an intra-S phase checkpoint response distinct from that required to suppress RDS (Falck et al., 2001). The sensitivity of Chk1 and Chk2 activation to caffeine in conjunction with very recently published data suggests that the caffeine-sensitive kinase(s) involved in the HU-induced activation is ATR (Tibbetts et al., 2000; Zhao and Piwnica-Worms, 2001).
Previous work demonstrated that the replication timing program controlling origin firing in mammalian cells is regulated by an ATM-independent, caffeine-sensitive checkpoint activated by replication blocks (Dimitrova and Gilbert, 2000). Here, we found that treating S phase–arrested cells with UCN-01 disrupted the Chk1 response, without any effect on Chk2, and resulted in the initiation of replication at progressively later-replicating sites as well as in an inability to elongate from previously arrested replication forks. UCN-01 has also been reported to inhibit at least one other kinase involved in cell cycle progression, C-TAK1 (Busby et al., 2000). However, C-TAK1 activity is completely unaffected by treatments that induce or abrogate this S phase checkpoint (unpublished data) and is very unlikely to be part of this checkpoint machinery. It is conceivable that UCN-01 may have other targets that could be involved in the intra-S phase checkpoint. However, taking together observations that Chk1 activation occurs only in response to replication blocks in a caffeine-sensitive and ATM-independent manner, and that inactivation of Chk1, but not Chk2, correlates with resumption of the replication program, the data presented here provide the first formal link between abrogation of Chk1 function and loss of the intra-S phase checkpoint controlling both stalled replication fork integrity and late origin firing. We conclude that, like 2-aminopurine and caffeine (Dimitrova and Gilbert, 2000), UCN-01 abrogates this intra-S phase checkpoint, presumably by interfering with Chk1-mediated phosphorylation of replication components allowing cells to initiate further replication and progress through the cell cycle in the presence of HU. Further work will be required to identify such components.
Genetic analysis of this pathway in budding yeast indicates a requirement for the Chk2 homologue, Rad53. In mammalian cells, Chk2, like Chk1, is activated in an ATM-independent manner in S phase–arrested cells. It is conceivable that in mammalian cells, suppression of late origin firing in the presence of HU requires Chk2 in addition to Chk1. Both proteins are required for the G2 DNA damage checkpoint, perhaps acting synergistically to induce cycle arrest via the phosphorylation of Cdc25 on Ser216 (Peng et al., 1997). If Chk2 is involved in this intra-S phase checkpoint, then our data indicate that Chk2 activation is not sufficient for its establishment.
Finally, in eukaryotic cells, the basis for the timing of replication origin firing is unknown. In principle, an intra-S phase checkpoint might influence timing if replication forks assembled early in S phase generated an inhibitory signal to prevent the initiation at later-firing origins. We found that Chk1 (and Chk2) activation only occurred when replicational stress was imposed and no activation could be detected during normal S phase progression. In addition, blocking Chk1 function in cells either arrested in S phase or undergoing normal S phase resulted in the initiation of replication at progressively later replicating sites with a time course similar to that observed in untreated cells. Taken together, these observations indicate that the Chk1-mediated intra-S phase checkpoint functions to arrest a preestablished replication timing program under conditions of replicational stress but is unlikely to play a role in the timing of initiation during normal S phase progression.
Materials and methods
Reagents, cell lines
HeLa (Johnson et al., 1993), AT22IJE-T (Ziv et al., 1997), and CHOC 400 (Dimitrova and Gilbert, 2000) cells were cultured as described. Recombinant wild-type and kinase-dead (D130A) Chk1 proteins were expressed as GST-fusion proteins in Sf21 insect cells infected with appropriate baculovirus and purified as described (Paterson, 1999). Recombinant ATR was expressed in HEK293 cells and purified as described (Hall-Jackson et al., 1999).
Cell synchronization and treatments
In replication arrest experiments, unless otherwise stated, exponential HeLa cells were treated for 30 min ± 5 mM caffeine and then with 2 mM hydroxyurea (HU) for 24 h in the continued presence/absence of caffeine. In irradiation experiments, HeLa cells were treated for 30 min +/− 5 mM caffeine and then irradiated (10 Gy) using a 137Cs source (IBL 437C irradiator; CIS UK Ltd) at 2.9 Gy/min. Cells were then incubated for 1 h ± caffeine. For synchrony experiments, metaphase HeLa cells were obtained as described (Johnson et al., 1993) using 40 ng/ml nocodozole (Calbiochem) for 14 h. Cells were then washed and released into normal medium for indicated times.
In checkpoint inhibition experiments, two complementary schemes were used: (1) Exponential CHOC 400 cells grown on coverslips were pulse labeled for 10 min with 10 μg/ml CldU (Sigma-Aldrich) and then treated with 50 μg/ml aphidicolin (Calbiochem) for 12 h ± 300 nM UCN-01 or 5 μM K-252c. At times after aphidicolin addition, coverslips were washed with warm PBS, transferred to warm drug-free medium plus 10 μg/ml IdU (Sigma-Aldrich), and incubated for 20 min; and (2) CHOC 400 mitotic cells (95% metaphase) obtained as described (Wu et al., 1997) were released into drug-free medium for 2 h. Then aphidicolin (5–10 μg/ml) was added to block cells at G1/S boundary by incubation for another 12–16 h. The aphidicolin concentration was then raised to 50 μg/ml and cells were grown in the continued presence/absence of UCN-01 or K-252c. At times thereafter, up to 12 h, coverslips were transferred to warm drug-free medium containing 10 μg/ml CldU and incubated for 10 min.
Cell lysis
Cells were lysed in ice cold lysis buffer (LB, 20 mM Tris acetate, pH 7.5, 0.27 M sucrose, 1% Triton X-100, 1 mM EDTA, 1 mM EGTA, 10 mM sodium β-glycerophosphate, 5 mM sodium pyrophosphate, 1 mM sodium orthovanadate, 50 mM sodium fluoride, 1 μM microcystin, 0.1% 2-mercaptoethanol, Complete proteinase inhibitor cocktail (Roche). Lysates were cleared by centrifugation at 16,000 g for 5 min, frozen in liquid nitrogen, and stored at –80°C.
Flow cytometry
Cells were trypsinized, washed with PBS, 1 mM EDTA, 1% BSA, resuspended in PBS, 1 mM EDTA, and fixed by addition of 10 vol of cold (−20°C) 70% ethanol and stored at −20°C. Cells were processed and analyzed on a Becton Dickinson FACScan® flow cytometer as described (Ball et al., 1997).
Immunofluorescence microscopy
For BrdU detection, cells grown on coverslips were pulse labeled with 25 μM BrdU for 30 min and then washed with ice-cold PBS, fixed with 4% paraformaldehyde in PBS for 10 min at 20°C, and permeabilized with PBS containing 0.2% Triton X-100 for 5 min at 20°C. For CldU and IdU detection, cells grown on coverslips were washed with PBS, fixed with 4°C 70% ethanol, and stored at 4°C. The differential staining of DNA sites substituted with halogenated derivatives of dU was performed essentially as described (Dimitrova and Gilbert, 2000).
Antibodies
Sheep polyclonal antibodies against Chk1 were raised against full-length recombinant human GST-tagged Chk1, and passed over a GST affinity column before affinity purification on a GST-Chk1 column. Anti-Chk2 antibodies were raised against a COOH-terminal peptide of human Chk2 (Chaturvedi et al., 1999) coupled to keyhole limpet hemocyanin and affinity purified on an immobilized peptide column.
Immunoblotting
Lysates (50 μg) were subjected to SDS-PAGE, optimized to resolve modified forms of Chk1 and Chk2 (acrylamide bisacrylamide ratio of separating gel was 13.8%:0.4% and 10%:0.1% for Chk1 and Chk2, respectively), transferred to nitrocellulose, and detected with 1° antibodies using HRP-conjugated rabbit anti–sheep 2° antibody and enhanced chemiluminescence (ECL; Amersham Pharmacia Biotech).
Kinase assays
Chk1 and Chk2 were immunoprecipitated from 150 μg of cell lysates using protein G Sepharose (5 μl; Amersham Pharmacia Biotech) conjugated to 2 μg of appropriate antibody. Immunocomplexes were washed twice in lysis buffer containing 0.5 M NaCl, once in lysis buffer and once in wash buffer (50 mM Tris-HCl, pH 7.5, 0.1 mM EGTA, 0.1% 2-mercaptoethanol), and Chk1 and Chk2 activity measured by the ability to phosphorylate a human Cdc25C-derived peptide Checktide, KKKVSRSGLYRSPSMPENLNRPR (Cross and Smythe, 1998). In ATR kinase assays (Hall-Jackson et al., 1999), sheared salmon sperm DNA was included at 2 ng/μl unless otherwise indicated.
Acknowledgments
We thank Y. Shiloh (Tel Aviv University, Tel Aviv, Israel) for AT cells, S. Elledge (Baylor College of Medicine, Houston, Texas) for baculovirus constructs and colleagues at Dundee for useful discussions.
This work is supported by National Institutes of Health grant GM57233-01 (to D.M. Gilbert) and an Association for International Cancer Research grant (to C. Smythe).
Notes
C. Feijoo and C. Hall-Jackson contributed equally to this work.
Footnotes
*Abbreviations used in this paper: 2-AP, 2-aminopurine; AT, ataxia telangiectasia; ATM, AT mutated; ATR, ATM- and Rad3-related protein; dU, deoxyuridine; HU, hydroxyurea; IP, immunoprecipitate; IR, ionizing radiation.
References
- Ball, K.L., S. Lain, R. Fahraeus, C. Smythe, and D.P. Lane. 1997. Cell-cycle arrest and inhibition of Cdk4 activity by small peptides based on the carboxy-terminal domain of p21WAF1. Curr. Biol. 7:71–80. [Abstract] [Google Scholar]
- Bentley, N.J., D.A. Holtzman, G. Flaggs, K.S. Keegan, A. DeMaggio, J.C. Ford, M. Hoekstra, and A.M. Carr. 1996. The Schizosaccharomyces pombe rad3 checkpoint gene. EMBO J. 15:6641–6651. [Europe PMC free article] [Abstract] [Google Scholar]
- Blasina, A., B.D. Price, G.A. Turenne, and C.H. McGowan. 1999. Caffeine inhibits the checkpoint kinase ATM. Curr. Biol. 9:1135–1138. [Abstract] [Google Scholar]
- Boddy, M.N., B. Furnari, O. Mondesert, and P. Russell. 1998. Replication checkpoint enforced by kinases Cds1 and Chk1. Science. 280:909–912. [Abstract] [Google Scholar]
- Brown, E.J., and D. Baltimore. 2000. ATR disruption leads to chromosomal fragmentation and early embryonic lethality. Genes Dev. 14:397–402. [Europe PMC free article] [Abstract] [Google Scholar]
- Brown, A.L., C.H. Lee, J.K. Schwarz, N. Mitiku, H. Piwnica-Worms, and J.H. Chung. 1999. A human Cds1-related kinase that functions downstream of ATM protein in the cellular response to DNA damage. Proc. Natl. Acad. Sci. USA. 96:3745–3750. [Europe PMC free article] [Abstract] [Google Scholar]
- Busby, E.C., D.F. Leistritz, R.T. Abraham, L.M. Karnitz, and J.N. Sarkaria. 2000. The radiosensitizing agent 7-hydroxystaurosporine (UCN-01) inhibits the DNA damage checkpoint kinase hChk1. Cancer Res. 60:2108–2112. [Abstract] [Google Scholar]
- Busse, P.M., S.K. Bose, R.W. Jones, and L.J. Tolmach. 1978. The action of caffeine on X-irradiated HeLa cells. III. Enhancement of X-ray-induced killing during G2 arrest. Radiat. Res. 76:292-307. [Abstract] [Google Scholar]
- Cairns, J. 1966. Autoradiography of HeLa cell DNA. J. Mol. Biol. 15:372–373. [Abstract] [Google Scholar]
- Chaturvedi, P., W.K. Eng, Y. Zhu, M.R. Mattern, R. Mishra, M.R. Hurle, X. Zhang, R.S. Annan, Q. Lu, L.F. Faucette, G.F. Scott, X. Li, S.A. Carr, R.K. Johnson, J.D. Winkler, and B.B. Zhou. 1999. Mammalian Chk2 is a downstream effector of the ATM-dependent DNA damage checkpoint pathway. Oncogene. 18:4047–4054. [Abstract] [Google Scholar]
- Chen, P., M. Gatei, M.J. O'Connell, K.K. Khanna, S.J. Bugg, A. Hogg, S.P. Scott, K. Hobson, and M.F. Lavin. 1999. Chk1 complements the G2/M checkpoint defect and radiosensitivity of ataxia-telangiectasia cells. Oncogene. 18:249–256. [Abstract] [Google Scholar]
- Chen, P., C. Luo, Y. Deng, K. Ryan, J. Register, S. Margosiak, A. Tempczyk-Russell, B. Nguyen, P. Myers, K. Lundgren, C.C. Kan, and P.M. O'Connor. 2000. The 1.7 A crystal structure of human cell cycle checkpoint kinase Chk1: implications for Chk1 regulation. Cell. 100:681–692. [Abstract] [Google Scholar]
- Cimprich, K.A., T.B. Shin, C.T. Keith, and S.L. Schreiber. 1996. cDNA cloning and gene mapping of a candidate human cell cycle checkpoint protein. Proc. Natl. Acad. Sci. USA. 93:2850–2855. [Europe PMC free article] [Abstract] [Google Scholar]
- Cross, D.A., and C. Smythe. 1998. PD 98059 prevents establishment of the spindle assembly checkpoint and inhibits the G2-M transition in meiotic but not mitotic cell cycles in Xenopus. Exp. Cell Res. 241:12–22. [Abstract] [Google Scholar]
- Dasso, M., and J.W. Newport. 1990. Completion of DNA replication is monitored by a feedback system that controls the initiation of mitosis in vitro: studies in Xenopus. Cell. 61:811–823. [Abstract] [Google Scholar]
- de Klein, A., M. Muijtjens, R. van Os, Y. Verhoeven, B. Smit, A.M. Carr, A.R. Lehmann, and J.H. Hoeijmakers. 2000. Targeted disruption of the cell-cycle checkpoint gene ATR leads to early embryonic lethality in mice. Curr. Biol. 10:479–482. [Abstract] [Google Scholar]
- Diffley, J.F. 1998. Replication control: choreographing replication origins. Curr. Biol. 8:R771–R773. [Abstract] [Google Scholar]
- Dimitrova, D.S., and D.M. Gilbert. 2000. Temporally coordinated assembly and disassembly of replication factories in the absence of DNA synthesis. Nat. Cell Biol. 2:686–694. [Europe PMC free article] [Abstract] [Google Scholar]
- Falck, J., N. Mailand, R.G. Syljuasen, J. Bartek, and J. Lukas. 2001. The ATM-Chk2-Cdc25A checkpoint pathway guards against radioresistant DNA synthesis. Nature. 410:842–847. [Abstract] [Google Scholar]
- Furnari, B., N. Rhind, and P. Russell. 1997. Cdc25 mitotic inducer targeted by chk1 DNA damage checkpoint kinase. Science. 277:1495–1497. [Abstract] [Google Scholar]
- Gilbert, D.M. 1986. Temporal order of replication of Xenopus laevis 5S ribosomal RNA genes in somatic cells. Proc. Natl. Acad. Sci. USA. 83:2924–2928. [Europe PMC free article] [Abstract] [Google Scholar]
- Graves, P.R., L. Yu, J.K. Schwarz, J. Gales, E.A. Sausville, P.M. O'Connor, and H. Piwnica-Worms. 2000. The Chk1 protein kinase and the Cdc25C regulatory pathways are targets of the anticancer agent UCN-01. J. Biol. Chem. 275:5600–5605. [Abstract] [Google Scholar]
- Hall-Jackson, C.A., D.A. Cross, N. Morrice, and C. Smythe. 1999. ATR is a caffeine-sensitive, DNA-activated protein kinase with a substrate specificity distinct from DNA-PK. Oncogene. 18:6707–6713. [Abstract] [Google Scholar]
- Hamlin, J.L. 1978. Effect of damage to early, middle, and late-replicating DNA on progress through the S period in Chinese hamster ovary cells. Exp. Cell Res. 112:225–232. [Abstract] [Google Scholar]
- Houldsworth, J., and M.F. Lavin. 1980. Effect of ionizing radiation on DNA synthesis in ataxia telangiectasia cells. Nucleic Acids Res. 8:3709–3720. [Europe PMC free article] [Abstract] [Google Scholar]
- Huberman, J.A., and A.D. Riggs. 1968. On the mechanism of DNA replication in mammalian chromosomes. J. Mol. Biol. 32:327–341. [Abstract] [Google Scholar]
- Johnson, R.T., C.S. Downes, and R.E. Meyn. 1993. The synchronisation of mammalian cells. The Cell Cycle: A Practical Approach. P. Fantes and R. Brooks, editors. Oxford University Press, Oxford. 1–24.
- Liu, Q., S. Guntuku, X.S. Cui, S. Matsuoka, D. Cortez, K. Tamai, G. Luo, S. Carattini-Rivera, F. DeMayo, A. Bradley, L.A. Donehower, and S.J. Elledge. 2000. Chk1 is an essential kinase that is regulated by Atr and required for the G(2)/M DNA damage checkpoint. Genes Dev. 14:1448–1459. [Europe PMC free article] [Abstract] [Google Scholar]
- Ma, H., J. Samarabandu, R.S. Devdhar, R. Acharya, P.C. Cheng, C. Meng, and R. Berezney. 1998. Spatial and temporal dynamics of DNA replication sites in mammalian cells. J. Cell Biol. 143:1415–1425. [Europe PMC free article] [Abstract] [Google Scholar]
- Mailand, N., J. Falck, C. Lukas, R.G. Syljuasen, M. Welcker, J. Bartek, and J. Lukas. 2000. Rapid destruction of human Cdc25A in response to DNA damage. Science. 288:1425–1429. [Abstract] [Google Scholar]
- Matsuoka, S., M. Huang, and S.J. Elledge. 1998. Linkage of ATM to cell cycle regulation by the Chk2 protein kinase. Science. 282:1893–1897. [Abstract] [Google Scholar]
- Murakami, H., and H. Okayama. 1995. A kinase from fission yeast responsible for blocking mitosis in S phase. Nature. 374:817–819. [Abstract] [Google Scholar]
- Nurse, P. 1997. Checkpoint pathways come of age. Cell. 91:865–867. [Abstract] [Google Scholar]
- Painter, R.B., and B.R. Young. 1980. Radiosensitivity in ataxia-telangiectasia: a new explanation. Proc. Natl. Acad. Sci. USA. 77:7315–7317. [Europe PMC free article] [Abstract] [Google Scholar]
- Paterson, A. 1999. Application of the baculoviral expression system to signal transduction. Signal Transduction: A Practical Approach. G. Milligan, editor. Oxford University Press, Oxford. 139–169.
- Peng, C.-Y., P.R. Graves, R.S. Thoma, Z. Wu, A.S. Shaw, and H. Piwnica-Worms. 1997. Mitotic and G2 checkpoint control: regulation of 14-3-3 protein binding by phosphorylation of Cdc25C on serine-216. Science. 277:1501–1505. [Abstract] [Google Scholar]
- Rao, P.N., and R.T. Johnson. 1970. Mammalian cell fusion: studies on the regulation of DNA synthesis and mitosis. Nature. 225:159–164. [Abstract] [Google Scholar]
- Sanchez, Y., C. Wong, R.S. Thoma, R. Richman, Z. Wu, H. Piwnica-Worms, and S.J. Elledge. 1997. Conservation of the Chk1 checkpoint pathway in mammals: linkage of DNA damage to Cdk regulation through Cdc25. Science. 277:1497–1501. [Abstract] [Google Scholar]
- Santocanale, C., and J.F. Diffley. 1998. A Mec1- and Rad53-dependent checkpoint controls late-firing origins of DNA replication. Nature. 395:615–618. [Abstract] [Google Scholar]
- Santocanale, C., K. Sharma, and J.F. Diffley. 1999. Activation of dormant origins of DNA replication in budding yeast. Genes Dev. 13:2360–2364. [Europe PMC free article] [Abstract] [Google Scholar]
- Savitsky, K., S. Sfez, D.A. Tagle, Y. Ziv, A. Sartiel, F.S. Collins, Y. Shiloh, and G. Rotman. 1995. The complete sequence of the coding region of the ATM gene reveals similarity to cell cycle regulators in different species. Hum. Mol. Genet. 4:2025–2032. [Abstract] [Google Scholar]
- Schlegel, R., and A.B. Pardee. 1986. Caffeine-induced uncoupling of mitosis from the completion of DNA replication in mammalian cells. Science. 232:1264–1266. [Abstract] [Google Scholar]
- Shirahige, K., Y. Hori, K. Shiraishi, M. Yamashita, K. Takahashi, C. Obuse, T. Tsurimoto, and H. Yoshikawa. 1998. Regulation of DNA-replication origins during cell-cycle progression. Nature. 395:618–621. [Abstract] [Google Scholar]
- Tibbetts, R.S., D. Cortez, K.M. Brumbaugh, R. Scully, D. Livingston, S.J. Elledge, and R.T. Abraham. 2000. Functional interactions between BRCA1 and the checkpoint kinase ATR during genotoxic stress. Genes Dev. 14:2989–3002. [Europe PMC free article] [Abstract] [Google Scholar]
- Walworth, N.C., and R. Bernards. 1996. rad-dependent response of the chk1-encoded protein kinase at the DNA damage checkpoint. Science. 271:353–356. [Abstract] [Google Scholar]
- Walworth, N., S. Davey, and D. Beach. 1993. Fission yeast chk1 protein kinase links the rad checkpoint pathway to cdc2. Nature. 363:368–371. [Abstract] [Google Scholar]
- Weinert, T. 1998. DNA damage and checkpoint pathways: molecular anatomy and interactions with repair. Cell. 94:555–558. [Abstract] [Google Scholar]
- Weinert, T.A., G.L. Kiser, and L.H. Hartwell. 1994. Mitotic checkpoint genes in budding yeast and the dependence of mitosis on DNA replication and repair. Genes Dev. 8:652–665. [Abstract] [Google Scholar]
- Wu, J.R., G. Yu, and D.M. Gilbert. 1997. Origin-specific initiation of mammalian nuclear DNA replication in a Xenopus cell-free system. Methods. 13:313–324. [Abstract] [Google Scholar]
- Zeng, Y., K.C. Forbes, Z. Wu, S. Moreno, H. Piwnica-Worms, and T. Enoch. 1998. Replication checkpoint requires phosphorylation of the phosphatase Cdc25 by Cds1 or Chk1. Nature. 395:507–510. [Abstract] [Google Scholar]
- Zhao, H., and H. Piwnica-Worms. 2001. Atr-mediated checkpoint pathways regulate phosphorylation and activation of human chk1. Mol. Cell. Biol. 21:4129–4139. [Europe PMC free article] [Abstract] [Google Scholar]
- Ziv, Y., A. Bar-Shira, I. Pecker, P. Russell, T.J. Jorgensen, I. Tsarfati, and Y. Shiloh. 1997. Recombinant ATM protein complements the cellular A-T phenotype. Oncogene. 15:159–167. [Abstract] [Google Scholar]
Articles from The Journal of Cell Biology are provided here courtesy of The Rockefeller University Press
Full text links
Read article at publisher's site: https://doi.org/10.1083/jcb.200104099
Read article for free, from open access legal sources, via Unpaywall:
https://rupress.org/jcb/article-pdf/154/5/913/1299503/jcb1545913.pdf
Citations & impact
Impact metrics
Citations of article over time
Alternative metrics
Smart citations by scite.ai
Explore citation contexts and check if this article has been
supported or disputed.
https://scite.ai/reports/10.1083/jcb.200104099
Article citations
CHK1 inhibitor SRA737 is active in PARP inhibitor resistant and CCNE1 amplified ovarian cancer.
iScience, 27(7):109978, 15 May 2024
Cited by: 0 articles | PMID: 39021796 | PMCID: PMC11253285
Investigations of the novel checkpoint kinase 1 inhibitor SRA737 in non-small cell lung cancer and colorectal cancer cells of differing tumour protein 53 gene status.
Explor Target Antitumor Ther, 4(5):1210-1226, 21 Dec 2023
Cited by: 0 articles | PMID: 38214010 | PMCID: PMC10776598
BRD7 suppresses tumor chemosensitivity to CHK1 inhibitors by inhibiting USP1-mediated deubiquitination of CHK1.
Cell Death Discov, 9(1):313, 25 Aug 2023
Cited by: 1 article | PMID: 37626049 | PMCID: PMC10457387
Photodynamic therapy induced cell cycle arrest and cancer cell synchronization: review.
Front Oncol, 13:1225694, 12 Jul 2023
Cited by: 5 articles | PMID: 37503319 | PMCID: PMC10369002
Review Free full text in Europe PMC
Ring finger protein 126 promotes breast cancer metastasis and serves as a potential target to improve the therapeutic sensitivity of ATR inhibitors.
Breast Cancer Res, 24(1):92, 20 Dec 2022
Cited by: 3 articles | PMID: 36539893 | PMCID: PMC9764525
Go to all (213) article citations
Other citations
Wikipedia
Data
Similar Articles
To arrive at the top five similar articles we use a word-weighted algorithm to compare words from the Title and Abstract of each citation.
Carcinogen-induced S-phase arrest is Chk1 mediated and caffeine sensitive.
Cell Growth Differ, 13(2):77-86, 01 Feb 2002
Cited by: 46 articles | PMID: 11864911
Chk1 inhibits replication factory activation but allows dormant origin firing in existing factories.
J Cell Biol, 191(7):1285-1297, 20 Dec 2010
Cited by: 134 articles | PMID: 21173116 | PMCID: PMC3010067
Characterization of a novel ATR-dependent, Chk1-independent, intra-S-phase checkpoint that suppresses initiation of replication in Xenopus.
J Cell Sci, 117(pt 25):6019-6030, 09 Nov 2004
Cited by: 61 articles | PMID: 15536124 | PMCID: PMC2701543
The mammalian DNA replication elongation checkpoint: implication of Chk1 and relationship with origin firing as determined by single DNA molecule and single cell analyses.
Cell Cycle, 6(22):2760-2767, 22 Aug 2007
Cited by: 54 articles | PMID: 17986860
Review
Funding
Funders who supported this work.
NIGMS NIH HHS (2)
Grant ID: GM57233-01
Grant ID: R01 GM057233