Abstract
Free full text

Solution structure of p53 core domain: Structural basis for its instability
Associated Data
Abstract
The 25-kDa core domain of the tumor suppressor p53 is inherently unstable and melts at just above body temperature, which makes it susceptible to oncogenic mutations that inactivate it by lowering its stability. We determined its structure in solution using state-of-the-art isotopic labeling techniques and NMR spectroscopy to complement its crystal structure. The structure was very similar to that in the crystal but far more mobile than expected. Importantly, we were able to analyze by NMR the structural environment of several buried polar groups, which indicated structural reasons for the instability. NMR spectroscopy, with its ability to detect protons, located buried hydroxyl and sulfhydryl groups that form suboptimal hydrogen-bond networks. We mutated one such buried pair, Tyr-236 and Thr-253 to Phe-236 and Ile-253 (as found in the paralogs p63 and p73), and stabilized p53 by 1.6 kcal/mol. We also detected differences in the conformation of a mobile loop that might reflect the existence of physiologically relevant alternative conformations. The effects of temperature on the dynamics of aromatic residues indicated that the protein also experiences several dynamic processes that might be related to the presence of alternative hydrogen-bond patterns in the protein interior. p53 appears to have evolved to be dynamic and unstable.
The tumor suppressor p53 has an unusually large number of functions in the cell, binds to many other proteins and ligands, and has a complex architecture (1, 2). Its core domain (residues 94–312) is the location of nearly all of the mutations that inactivate p53 in some 50% of human cancers (3). The core domain is naturally unstable, with a melting temperature of ≈42–44°C (4), and many oncogenic mutations inactivate the core by either simply destabilizing or distorting it (5). Although the crystal structures of the wild-type (WT) human core domain (6) and several mutants (7, 8) have been solved, it is not clear why the protein is so unstable.
NMR spectroscopy can give important complementary information about local dynamics and does not suffer from constraints induced on mobile structures by crystal packing and so may provide clues about the stability and function of p53. However, the size of the domain (≈25 KDa) together with its low solubility (≈400 μM) and thermal and long-term stability render NMR experiments difficult. We solved the structure by using a combination of high-field magnets, ultrasensitive cryoprobes, and complex labeling patterns (15N,13C,2H and reverse protonation). We also determined amide proton exchange rates to study local and global unfolding at the level of individual residues (9, 10). Direct observation of buried protons and dynamic processes gave previously undescribed structural information that is inaccessible to x-ray crystallography and identified structural reasons for instability that were confirmed by protein engineering.
Results and Discussion
Solution Structure of WT p53 Core Domain.
Crucial to the solution of the structure was the use of perdeuteration of all of the side chains with reverse-labeling techniques of selective reintroduction of protonated side chains (11). Perdeuterated samples provide high-quality spectra but lose the structural information of the side chains. Therefore, we reintroduced proton nuclei in several side chains including those of Ile, Val and Leu (12), methyl groups, aromatic Tyr and Phe (13), and Met and Arg residues. The gain in sensitivity afforded high-quality 2D (Fig. 1) and 3D data (Fig. 2) that were combined with the analysis of partly deuterated samples to get many restraints (Table 1). The spectra indicated some conformational heterogeneity by the absence of several amide signals from the 1H-15N-heteronuclear single quantum correlation (HSQC) spectrum. Line broadening was still detectable in perdeuterated samples around Lys-120, whose signal was missing from the spectra, and for Met-246, which appeared to occupy two conformations.
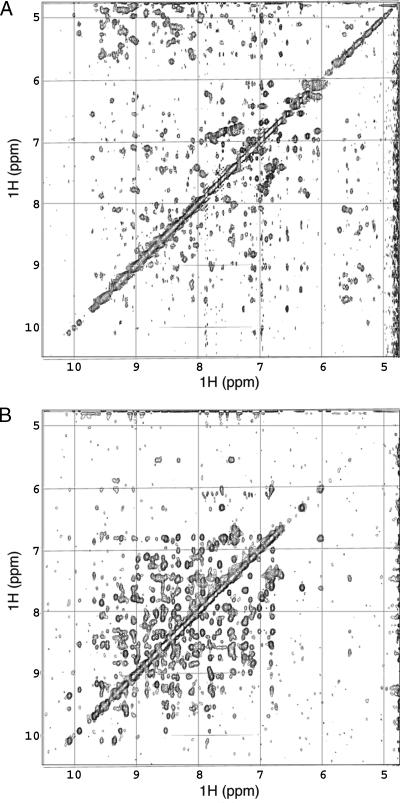
Comparison of the proton amide region of the p53 core domain in protonated (A) and perdeuterated (B) 2D NOESY spectra. The spectrum of the perdeuterated samples has far more amide–amide crosspeaks.

Example of a 13C-13C plane (corresponding to Val-157 Hγ2 in the third dimension) of the 13C-HSQC-NOESY-13C-HSQC spectrum. Off-diagonal peaks show different methyl–methyl NOEs (labeled) to Val-157 Hγ2 methyl group.
Table 1.
Structure calculation statistics for the NMR structure of p53 core domain (36 conformers)
Structural constraints | |
![]() ![]() ![]() ![]() | 722 |
![]() ![]() ![]() ![]() | 436 |
![]() ![]() ![]() ![]() | 175 |
![]() ![]() ![]() ![]() | 783 |
![]() ![]() ![]() ![]() | 263 |
![]() ![]() ![]() ![]() | 72 |
![]() ![]() ![]() ![]() | 9 |
![]() ![]() ![]() ![]() | 2,460 |
Statistics for accepted structures | |
![]() ![]() ![]() ![]() | |
![]() ![]() ![]() ![]() ![]() ![]() ![]() ![]() | 0.014 ± 0.009 |
![]() ![]() ![]() ![]() ![]() ![]() ![]() ![]() | 0.46 ± 0.05 |
![]() ![]() ![]() ![]() | |
![]() ![]() ![]() ![]() ![]() ![]() ![]() ![]() | 235 ± 19 |
![]() ![]() ![]() ![]() ![]() ![]() ![]() ![]() | 64 ± 6 |
![]() ![]() ![]() ![]() | |
![]() ![]() ![]() ![]() ![]() ![]() ![]() ![]() | 0.0018 ± 0.0001 |
![]() ![]() ![]() ![]() ![]() ![]() ![]() ![]() | 0.34 ± 0.01 |
![]() ![]() ![]() ![]() ![]() ![]() ![]() ![]() | 0.23 ± 0.02 |
![]() ![]() ![]() ![]() | |
![]() ![]() ![]() ![]() ![]() ![]() ![]() ![]() | 0.7 ± 0.1 |
![]() ![]() ![]() ![]() ![]() ![]() ![]() ![]() | 1.2 ± 0.1 |
![]() ![]() ![]() ![]() | |
![]() ![]() ![]() ![]() ![]() ![]() ![]() ![]() | 1.0 ± 0.1 |
![]() ![]() ![]() ![]() ![]() ![]() ![]() ![]() | 1.7 ± 0.1 |
A total of 36 structures out of 50 were accepted where no distance violation was >0.3 Å and no angle violations were >0.5°.
*Residues 100–116, 123–182, 188–208, 211–223, 228–288.
The overall NMR structure of p53 core domain is a β-sandwich, composed of two antiparallel β-sheets (Fig. 3) with a small β-hairpin (124–135) in contact with the second β-sheet, closing the access to the hydrophobic core. The C-terminal helix, which is part of the DNA-binding interface and packed against this hairpin, is more than one turn longer in the NMR structure (278–291) than in the crystal structure. There are two more helices in the middle of loop 2: one involved in Zn2+ coordination (177–181) and a small 310 helix (166–168).
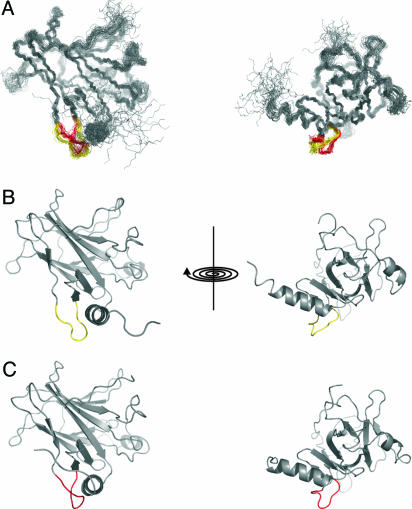
Solution structure of DNA-binding domain of p53. (A) Superposition of the 30 lowest-energy conformers representing the NMR structure of human p53 DNA-binding domain (Protein Data Bank ID code 2FEJ). Differences on the conformation of loop 1 have been found across the ensemble and are highlighted. (B) Open conformer in yellow. (C) Closed conformer in red.
The definition of the NMR structure was very high with rms deviation (rmsd) of 0.7 ± 0.1 Å for all of the secondary structure elements (Table 1), which are nearly identical to the p53–DNA complex (6) (rmsd 1.0 ± 0.1 Å; Table 1). The most notable difference was the orientation of loop 1. In the crystal structure, this loop folds on the C-terminal helix. In the solution structure, there were two alternative conformations compatible with the nuclear Overhauser effect (NOE) data: one (Fig. 3 in red) was similar to the crystal DNA-bound form (6), whereas the other (Fig. 3 in yellow) resembled the orientation of this loop in a mutant (A. Joerger and A.R.F., unpublished data). Although there were no violations of NOEs involving residues in loop 1 that could indicate that the NOE information, just like the chemical shift, is averaged, we cannot rule out that these alternative conformations arise from an insufficient number of distance restraints. However, the NMR data indicated conformational flexibility on the millisecond to microsecond time scale for this part of the protein and mobility in loop 1. Further, there are high B factors in crystal structures of p53 mutants in this region, peaking at Lys-120 (7, 8). The resonances of Lys-120 were missing in the spectra because of severe line broadening, which is a hallmark of slow conformational motions. Low concentration (200 μM) and high Mr compromise the measurement of high-quality 15N relaxation data, but qualitative inspection of T2 shows chemical exchange in the submillisecond time scale in this loop (data not shown). Further, H/D-exchange data showed that several amide protons (Leu-114, His-115, Gly-117, and Val-122) had disappeared within the first 12 min after solvent exchange, indicating the absence of a stable hydrogen bond (H-bond) network in this loop.
Protein Hydration and Stability.
Several hydrophilic side chains that are totally conserved in other vertebrate p53 genes or replaced by polar residues (see Fig. 8, which is published as supporting information on the PNAS web site) had very low solvent accessibility (Table 2). At least five of them (Thr-155, Ser-215, Thr-253, Thr-256, and Ser-269) are temperature-sensitive hot spots (14). Some of these residues had low protection factors in the H/D experiments (e.g., Ser-240, Tyr-163) indicating that their backbone accessibility is higher than their side-chain accessibility. However, most of them had high backbone protection factors (e.g., Cys-238, Thr-253, Thr-256, Tyr-234, and Tyr-236) (see Fig. 9, which is published as supporting information on the PNAS web site) with their corresponding HSQC peaks still present after several days in D2O.
Table 2.
Hydroxyl/sulfhydryl assignments of p53 WT DNA-binding domain
OH/SH | %ASA (NMR)* | δ,† ppm | NOE H2O‡ | H/B partner§ | Homology¶ |
---|---|---|---|---|---|
Ser-127 | 4.0 | 7.16 | Yes | Glu-286 Oε | Ser |
Ser-215 | 2.7 | 5.80 | Yes | Arg-158 sc (a) | Ser |
Ser-240 | 3.0 | 6.40 | Yes | Surf. H2O (a) | Ser |
Ser-269 | 5.0 | 5.90 | Yes | Surf. H2O (a) | Ser, Cys |
Thr-155 | 0.0 | 6.32 | No | Pro-152 O | Thr, Ser, Ala |
Thr-211 | 6.0 | 5.56 | Yes | Surf. H2O | Thr, Asn |
Thr-230 | 6.0 | 4.93 | a | Glu-221 O | Thr |
Thr-253 | 0.0 | 1.86 | No | Tyr-236 Oη | Thr |
Thr-256 | 0.5 | 6.74 | a | Surf. H2O | Thr |
Cys-124 | 5.1 | N/A | Met-133 Sδ | Cys, Ser, Trp | |
Cys-135 | 0.0 | 1.43 | Yes | (a) | Cys |
Cys-141 | 0.0 | 2.60 | Yes | (a) | Cys, Thr |
Cys-176 | 0.0 | N/A | Zn2+ | Cys | |
Cys-238 | 1.4 | N/A | Zn2+ | Cys | |
Tyr-163 | 1.2 | N/A | Surf./Bur. H2O | Tyr | |
Tyr-205 | 0.8 | 10.62 | Yes | Buried H2O | Tyr |
Tyr-220 | 1.0 | 9.40 | Yes | Buried H2O | Tyr |
Tyr-234 | 0.0 | 7.87 | No | Buried H2O | Tyr, Leu |
Tyr-236 | 0.0 | 6.97 | No | None | Tyr, Phe |
*Percentage of mean accessibility solvent surface (ASA) across the NMR ensemble.
†Chemical shifts (N/A, not assigned).
‡The presence of NOE (exchange peak) with the solvent. a, ambiguous.
§Putative H-bond partners in the previous crystal structure (6). (a), ambiguous.
¶The degree of conservation in other vertebrate p53 genes (see Fig. 8 for details).
NMR spectroscopy allows the direct observation of protons and therefore is a very powerful tool for studying protein hydration (15). Assignments of labile protons such as hydroxyl and sulfhydryl are underrepresented, however, in the chemical-shift database (16), because they can only be observed when they are in slow exchange with the solvent (buried). By using triple-labeled (15N/13C/2H) samples, labile resonances not directly bound to 15N or 13C could be readily assigned to hydroxyl or sulfydryl groups in the protein, which could be unambiguously assigned by their NOE interactions with other neighboring protons. We observed and assigned 13 hydroxyl and 2 sulfhydryl groups of low solvent accessibility (Table 2). The cysteines coordinating the Zn2+ ion were among the missing sulfhydryl protons: one of them (242) because it is exposed and in fast exchange with the solvent and the other two (176 and 238) because they are likely to be unprotonated ligands. The backbone amides of Cys-176 and 242 exchanged more rapidly than did Cys-238 (protection factor = 7.5 × 105). Interestingly, Cys-238 was buried but not involved in intramolecular H-bonds, whereas Cys-242 formed an interaction with carbonyl of Asn-239. H/D exchange gave additional information about the structure and stability around the metal coordination site.
The presence of a buried unpaired H-bonding group costs between 0.5 and 1.5 kcal·mol−1 (17). Frequently, buried water molecules can act as the H-bonding partners, but at enthalpic and entropic costs (18). Most of the buried hydroxyl or sulfhydryl protons showed strong NOEs (or exchange peaks) with water (Table 2 and Fig. 4), a good indication of the presence of water molecules in their vicinity. Most of the Ser and Thr hydroxyls are close to the surface, and the observed crosspeak is likely to arise from surface waters with residence times in the picosecond to nanosecond time scale (fast exchange with the bulk solvent).
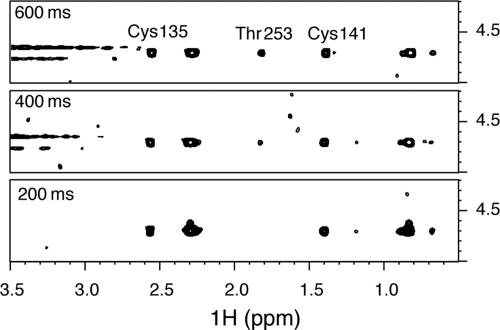
Detailed view of 2D NOESY spectra showing the NOEs (or exchange) peaks between protected hydroxyl/sulfhydryl protons and the water resonance. Three different spectra are shown at increasing mixing times (200, 400, and 600 ms). The crosspeak between Thr-253 Hγ2 and the water can only be observed at long mixing times, which indicates the absence of structural waters nearby this protected OH.
There were at least four hydroxyls (Thr-155 Hγ1, Tyr-234 Hη, Tyr-236 Hη, and Thr-253 Hγ1) without the water NOE crosspeak. Three of them are critical for the formation of the protein core (Fig. 5). The Thr residues were involved in intramolecular H-bonds: Thr-155 Hγ1··Pro 152 O; and Thr-253 Hγ1··Tyr-236 Oη. The highly conserved Tyr-234 (Fig. 8) is likely to be H-bonded to structural water molecules that have tetrahedral coordination to protein H-bond donors and acceptors and low B-factors in the x-ray structure (e.g., H2O 2367 in the structure with Protein Data Bank ID code 1TUP). The lack of the intermolecular NOE peak could be explained if this water molecule is in slow to intermediate exchange (<10−2 s−1) with the bulk solvent. Thus, the overwhelming proportion of solvent molecules will cause a severe line broadening of the structural water resonances that preclude its observation. H/D exchange data supported this hypothesis because all backbone amides neighboring this water are protected, so the water exchange can only occur upon global unfolding.

Structural detail of the H-bond networks among Thr-253, Tyr-236, and Tyr-234 in the hydrophobic core of p53 DNA-binding domain. These three residues show protected hydroxyl groups (see text) consistent with null accessibility in the structure. (A) Most of the conformers representing the NMR structure show a H-bond between Thr-253 Hγ2 and Tyr-236 Oη. (B) Only little population of conformers displayed a suboptimal H-bond between Tyr-236 Hη and Thr-253 Oγ2. Although protected, there is not an intramolecular H-bond acceptor for Tyr-234 Hη, and the structural analysis suggests the presence of a structural water molecule stabilizing this hydroxyl inside the hydrophobic core of the protein (see text).
Evidence from NMR and crystallographic structures and the lack of NOEs with water supports the absence of a H-bond partner for Tyr-236 Hη. Although the substitution of Tyr-236 by Phe in other p53 genes (Fig. 8) removes a hydroxyl group with one unpaired H-bond from the interior, it also removes the H-bond acceptor of the totally conserved Thr-253 hydroxyl (Fig. 5A). These compensating factors lead to the Tyr-236 → Phe mutant being only marginally more stable (0.27 kcal/mol) than WT (19). However, a double mutation (Tyr/Phe and Thr/Ile) as found in human p53 paralogs p63 and p73 (Fig. 8) could cause significant stabilization. Model building indicated that the p53 structure could accommodate the double mutation without steric clashes. We made the double mutant Tyr-236 → Phe/Thr-253 → Ile and found it to be 1.6 kcal/mol more stable than WT (Fig. 6).
It is worth summarizing the evidence for the assignment the hydroxyl and sulfydryl protons. Their resonances disappeared after D2O exchange. Therefore, they are labile protons. They are not 15N- or 13C-bound because they did not show active 1J coupling with either of these nuclei. The resonances of the hydroxyls in the amide–amide region (Tyr OHs mostly) did not correspond to any 15N HSQC peak: e.g., the OH of Tyr-205 was at >10 ppm, and the lowest field amide in the HSQC is at 9.5 ppm. We checked that these resonances did not come from other buffer components (i.e., use fully deuterated Tris·HCl) or contaminants in the expression/purification of the sample. The specific assignment of each of these groups was based on the NOEs that each of them had with other NHs in the structure. Because these groups are buried, it is possible to observe several NOEs with NHs (in the perdeuterated sample) and with aromatic or methyls (in the reverse-protonated samples). It is possible to assign without ambiguity nearly all of the OH and SH protons. There was only one that could not be assigned because the lack of NOEs with other protons (Table 2).
Structural Plasticity of the Hydrophobic Core.
The presence of buried polar groups in the core of the structure may perhaps affect its dynamics. We found from the temperature dependence of the NMR spectra for the Tyr residues in the Tyr reverse-protonated sample (Fig. 7) that several side chains did have complex dynamic behavior. The proton signals of the Tyr-236 ring evolved into intermediate exchange with decreasing temperature, indicating a conformational exchange process, such as unusually slow ring flipping or the formation of alternative H-bonds networks between the Tyr-236/Thr-253 pair. Oη acts as a H-bond acceptor for the Thr-253 hydroxyl in the NMR structure. However, a small rearrangement would allow Thr-253 to act as acceptor of Tyr-236 Hη. Both alternatives might be energetically similar but cannot happen at the same time because of the geometry of the H-bond interaction. There was evidence that the dynamic mode observed in Tyr-236 was a local rather than global feature: first, the backbone amides of Thr-253 and Tyr-236 were among the slowest exchanging in the protein (still protected after 20 d; Fig. 9), confirming the rigidity of the β-sandwich scaffold; second, Tyr-234, which is close to Tyr-236, did not have a detectable temperature variation and is more fixed and forms a very stable water-mediated H-bond network (see above).
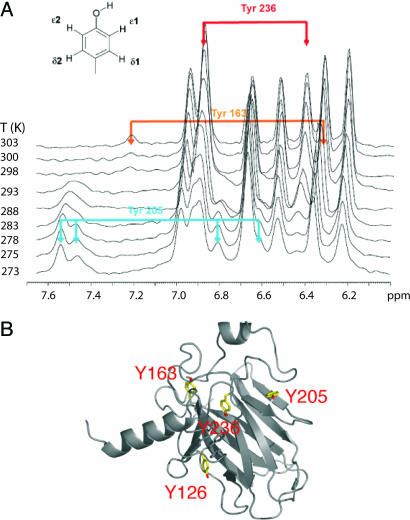
Temperature dependence of p53 core domain internal dynamics. (A) Temperature dependence of the aromatic spectrum of Tyr residues of p53 DNA binding domain. The spectra have been recorded with the deuterated Tyr reverse-protonated sample in 2H2O to remove the interference of other aromatic and amide signals. (B) Locations of some of the mobile Tyr residues in the structure of p53 DNA-binding domain.
Other Tyr residues had temperature-dependent dynamics (Fig. 7): Tyr-163 signals disappeared at low temperature as the spin system moved further into intermediate exchange; Tyr-205 spin system evolved from intermediate into slow exchange (four distinctive resonances); and Tyr-126 was undetectable over the whole temperature range. All this behavior indicates different motions on different time scales (probably ≈10−3 s) and that these side chains are sampling different local motions. For example, the motion of Tyr-126, which is stacked with Phe-113 in the x-ray structures, broadens the linewidths of Phe-113 but not the resonances of Phe-270, which stacks on the other face of Phe-113. It seems that the motion is quenched at the level of Phe-113.
All these “mobile” Tyr residues are at key positions in the structure. Tyr-126 forms a key contact between the β-sandwich and the helix–loop–hairpin motif, a crucial region that forms the interface with the DNA, and Tyr-163 and Tyr-205 are involved in contacts between the β-sandwich and loop 3, which very likely forms a protein–protein interface in the DNA bound form.
Irrespective of the details, we have found that several Tyr residues are involved in dynamic processes that are likely to occur on different time scales (i.e., are uncoupled). Their side chains are deep in the hydrophobic core and are at key junctions between the main structural scaffold, the β-sandwich, and other structural elements that are very important for p53 function. Our data demonstrate that, in reality, the structure is substantially more flexible than was apparent from crystal structures (6). Together with the structure, the presence of different dynamics is likely to be very important and perhaps a conserved feature in different p53 homologs.
In conclusion, it seems that the p53 core domain has evolved to be naturally unstable at temperatures close to physiological. A suboptimally designed protein core is an important factor in this instability. Future studies can now address whether and why this intrinsic instability is important for the function of the protein.
Materials and Methods
Molecular Cloning.
The DNA sequence 92–312 of WT human p53 was subcloned into a pRSET-derived vector (courtesy of M. D. Allen, Medical Research Council Centre) containing an N-terminal fusion of 6xHis/lipoamyl domain/thrombin cleavage sequence. The p53 Y236F/T253I double mutant was generated from the WT by using the QuikChange site-directed mutagenesis kit (Stratagene) and subcloned into the polylinker of vector pET-24a(+) (Novagen).
Protein Expression and Purification.
Isotopically labeled (15N,13C) samples were expressed in Escherichia coli C41(DE3) cells (20). Cultures were grown in minimal M9 medium supplemented with ampicillin (120 μg/ml), vitamin mix, and the appropriate nitrogen (1 g/liter of 15NH4) and carbon (4g/liter of 13C-Glucose) sources. After induction at 37°C, cultures were incubated overnight at 25°C and harvested and disrupted by sonication. After centrifugation, p53-containing fusion protein was purified by Ni2+ affinity [Ni-NTA (Qiagen, West Sussex, U.K.) or Ni Hi-trap (Amersham Pharmacia)], and the fusion protein was dialyzed and cleaved by thrombin digestion (overnight at room temperature in 150 mM NaCl/25 mM Tris·HCl, pH 7.0/5 mM DTT). The product was diluted twofold with 25 mM Tris·HCl (pH 7.0) plus 5 mM DTT, filtered, and purified by ion-exchange chromatography (Resource 15S, Amersham Pharmacia).
The production of perdeuterated and reverse-protonated samples followed a similar protocol using 2H2O-based M9 medium supplemented with 0.3–1% rich triple-labeled (13C/2H/15N) medium (BioExpress, Cambridge Isotope Laboratories, Cambridge, MA). Cells were grown directly in 2H2O without prior adaptation and at 25°C. Reverse-labeled samples were produced by adding the corresponding α-ketoacids (12) or amino acid (Tyr, Phe, Arg, or Met) to the culture 2–3 h before induction.
p53 constructs used in the urea denaturization (WT and double mutant) were expressed and purified according to published protocols (19).
NMR Spectroscopy.
The core domain of p53 aggregates at temperatures of ≈37°C (21). The transverse relaxation times of the amide protons indicated the presence of species of high Mr in fast exchange with the monomers at concentrations >400 μM (pH 7.2; 150 mM ionic strength). Excellent spectra were obtained at 20°C by using 200–400 μM samples. Triple resonance experiments were performed on a triple-labeled (13C,15N,2H) sample to confirm and complete our previous backbone assignments of p53 core domain (K. B. Wong, S.M., and V.F., unpublished data). HNCA, HNCO, HN(CO)CA, CBCA(CO)HN, HN(CO)CC (8 and 12 ms for carbon spin lock), and HNCACB experiments were modified from the standard (22) to include deuterium decoupling. Side-chain assignments were completed with various HCCH-COSY in double (13C,15N) or triple (13C,15N,2H 50%) labeled samples.
Distance constraints were obtained from a collection of NOESY spectra with mixing times ranging from 100 ms (protonated samples) to 200 ms (deuterated and reverse-labeled samples). The following series was used in the order (sample number, labeling regime, and spectra): 1, unlabeled, 2D NOESY water, and D2O (80 ms); 2, 2H (50%), 13C, 15N, 2D NOESY and various 3D NOESY-HSQC; 3, 2H (100%), 13C, 15N, 2D NOESY (100–600 ms mixing), 3D NOESY-HSQC; 4, as 3 plus reverse 13CH3 Ile,Leu,Val, 2D NOESY, 3D NOESY-13C-HSQC, 3D 13C-HSQC-NOESY-13C-HSQC; 5, as 4 plus reverse 12C/1H Tyr, 2D NOESY, 3D NOESY-13C-HSQC; 6, as 3 plus reverse 12C/1H Phe, Met, 2D NOESY (200 ms); and 7, as 3 plus reverse 12C/1H Phe, Arg, 2D NOESY (200 ms). For the perdeuterated sample, several 2D NOESY with 100, 200, 400, and 600 ms were recorded to check the absence of spin-diffusion. In general, all of the NOESY-like experiments were recorded for samples between 300 and 400 μM in the optimal buffer conditions (25 mM d11-Tris·HCl, pH 7.1/150 mM NaCl/10 mM d10-DTT) at 298 K in 600- (with cryoprobe) and 800-MHz spectrometers. For the Tyr reverse-labeled sample, an additional set of 2D NOESY experiments was recorded at various temperatures to analyze the changes in chemical exchange regime of the ring resonances as a function of temperature.
Amide Proton-Exchange Experiments.
15N-labeled p53 core domain was buffer-exchanged into D2O buffer (25 mM phosphate, pH 7.2/150 mM NaCl/5 mM DTT) using a NAP-column (Amersham Pharmacia) resulting in a final concentration of ≈280 μM and placed immediately into an NMR tube and into the magnetic field. A series of 1H-15N fast HSQC spectra (23) were acquired on a DRX600 spectrometer (Bruker, Karlsruhe, Germany) with a TXI cryoprobe over 4 d with further experiments after 10 and 20 d, respectively. The middle of the acquisition time for each spectrum was taken as the time base for the decay of the signals. The volume integrals for each crosspeak were integrated, and the decays were fitted to a single exponential decay. Protection factors (P) for backbone amide were extracted according to the formula P = kint/kexobs, where kint was obtained from elsewhere (24).
Structure Calculation.
Distance constraints were obtained from several 2D and 3D NOESY-type experiments in deuterated, reverse-protonated, and partially deuterated samples. The interproton distance constraints were classified into four categories corresponding to 1.8–2.8, 1.8–3.5, 1.8–4.5, and 1.8–5.5 Å, respectively. Crosspeaks from known secondary structures within the protein were used for calibration purposes. Backbone and ϕ angular constraints were determined from the 13C chemical shifts by using the program talos (25). The final ensemble of structures was calculated with the program cns (26). Thirty-six structures out of 50 were accepted where no distance violation was >0.3 Å and no angle violation was >0.5°. Structure calculation statistics are summarized in Table 1. The data are deposited in the Protein Data Bank (PDB ID code 2FEJ).
Equilibrium Denaturation.
Samples for urea denaturation experiments were prepared by using a Hamilton MicrolabM dispenser from stock solutions of urea, buffer, and protein to contain 1 μM protein in 25 mM sodium phosphate buffer (pH 7.2), 150 mM KCl, 5 mM DTT, and increasing concentrations of urea. Samples were incubated at 10°C for 14 h before measurements. The intrinsic fluorescence spectra of p53, excited at 280 nm, were recorded in the range of 300–400 nm on a PerkinElmer LS50B spectrofluorimeter equipped with a Waters 2700 sample manager and controlled by laboratory software. The data were analyzed by using the equation described in ref. 4. The value of urea for 50% denaturation was 3.06 ± 0.04 M for WT and 3.56 ± 0.02 M for the double mutant Y236F/T253I, with an average m-value of 3.3 kcal/mol·M−1 (Fig. 6).
Acknowledgments
We thank Drs. Mark Bycroft, Mark Allen, and Andreas Joerger for helpful discussions. This work was supported by Cancer Research UK, the Medical Research Council, and European Community FP6 funding. H.T. was supported by a Boehringer Ingelheim Ph.D. scholarship. This publication reflects the authors’ views and not necessarily those of the European Community. The Community is not liable for any use that may be made of the information.
Glossary
Abbreviations:
HSQC | heteronuclear single quantum correlation |
NOE | nuclear Overhauser effect |
rmsd | rms deviation. |
Footnotes
Conflict of interest statement: No conflicts declared.
Data deposition: The atomic coordinates have been deposited in the Protein Data Bank, www.pdb.org (PDB ID code 2FEJ).
References
Articles from Proceedings of the National Academy of Sciences of the United States of America are provided here courtesy of National Academy of Sciences
Full text links
Read article at publisher's site: https://doi.org/10.1073/pnas.0510941103
Read article for free, from open access legal sources, via Unpaywall:
https://europepmc.org/articles/pmc1413739?pdf=render
Citations & impact
Impact metrics
Citations of article over time
Alternative metrics
Article citations
Oncogenic p53 triggers amyloid aggregation of p63 and p73 liquid droplets.
Commun Chem, 7(1):207, 16 Sep 2024
Cited by: 0 articles | PMID: 39284933 | PMCID: PMC11405828
Structural basis for the dynamic chaperoning of disordered clients by Hsp90.
Nat Struct Mol Biol, 31(10):1482-1491, 18 Jun 2024
Cited by: 0 articles | PMID: 38890550
Computational Methods to Predict Intrinsically Disordered Regions and Functional Regions in Them.
Methods Mol Biol, 2627:231-245, 01 Jan 2023
Cited by: 0 articles | PMID: 36959451
Review
Therapeutic Strategies to Activate p53.
Pharmaceuticals (Basel), 16(1):24, 24 Dec 2022
Cited by: 9 articles | PMID: 36678521 | PMCID: PMC9866379
Review Free full text in Europe PMC
Conformational Heterogeneity and Frustration of the Tumor Suppressor p53 as Tuned by Punctual Mutations.
Int J Mol Sci, 23(20):12636, 20 Oct 2022
Cited by: 1 article | PMID: 36293489 | PMCID: PMC9604312
Go to all (105) article citations
Data
Data behind the article
This data has been text mined from the article, or deposited into data resources.
BioStudies: supplemental material and supporting data
Protein structures in PDBe (3)
-
(3 citations)
PDBe - 2FEJView structure
-
(1 citation)
PDBe - 1TRSView structure
-
(1 citation)
PDBe - 1TUPView structure
Similar Articles
To arrive at the top five similar articles we use a word-weighted algorithm to compare words from the Title and Abstract of each citation.
Stability of the core domain of p53: insights from computer simulations.
BMC Bioinformatics, 9 Suppl 1:S17, 01 Jan 2008
Cited by: 10 articles | PMID: 18315848 | PMCID: PMC2259418
Stabilising the DNA-binding domain of p53 by rational design of its hydrophobic core.
Protein Eng Des Sel, 22(7):421-430, 10 Jun 2009
Cited by: 17 articles | PMID: 19515728 | PMCID: PMC2699268
Structures of oncogenic, suppressor and rescued p53 core-domain variants: mechanisms of mutant p53 rescue.
Acta Crystallogr D Biol Crystallogr, 69(pt 10):2146-2156, 20 Sep 2013
Cited by: 7 articles | PMID: 24100332 | PMCID: PMC3792646
Crystal structure of a superstable mutant of human p53 core domain. Insights into the mechanism of rescuing oncogenic mutations.
J Biol Chem, 279(2):1291-1296, 08 Oct 2003
Cited by: 111 articles | PMID: 14534297
Funding
Funders who supported this work.
Medical Research Council (1)
Cancer and Mutational Diseases
Professor Sir Alan Fersht, Mrc Centre For Protein Engineering
Grant ID: MC_U105474168