Abstract
Free full text

Recent insights into the biological roles of mucin-type O-glycosylation
Abstract
In this special issue of the Glycoconjugate Journal focusing on glycosciences and development, we summarize recent advances in our understanding of the role of mucin-type O-glycans in development and disease. The presence of this widespread protein modification has been known for decades, yet identification of its biological functions has been hampered by the redundancy and complexity of the enzyme family controlling the initiation of O-glycosylation, as well as the diversity of extensions of the core sugar. Recent studies in organisms as diverse as mammals and Drosophila have yielded insights into the function of this highly abundant and evolutionarily-conserved protein modification. Gaining an understanding of mucin-type O-glycans in these diverse systems will elucidate crucial conserved processes underlying many aspects of development and homeostasis.
Introduction
Proteins are decorated with a variety of carbohydrate side chains that are responsible for mediating many diverse cellular and developmental events (reviewed in [1]). The two major forms of protein glycosylation found in eukaryotes are N-linked and O-linked, designated as such by virtue of their glycosidic linkage to the corresponding amino acid. While many types of O-glycosylation are known to occur, the most abundant is mucin-type O-glycosylation, which involves the addition of an N-acetylgalactosamine residue (GalNAc) to the hydroxyl group of either serine or threonine to form the Tn antigen (Tn Ag) (GalNAcα1-S/T) (Fig. 1). This type of glycosylation is evolutionarily conserved, being found in mammals down to certain types of fungi [2, 3]. Here, we describe the enzymes responsible for the synthesis of the most common mucin-type O-glycans and focus on recent advances in our understanding of their diverse biological roles.
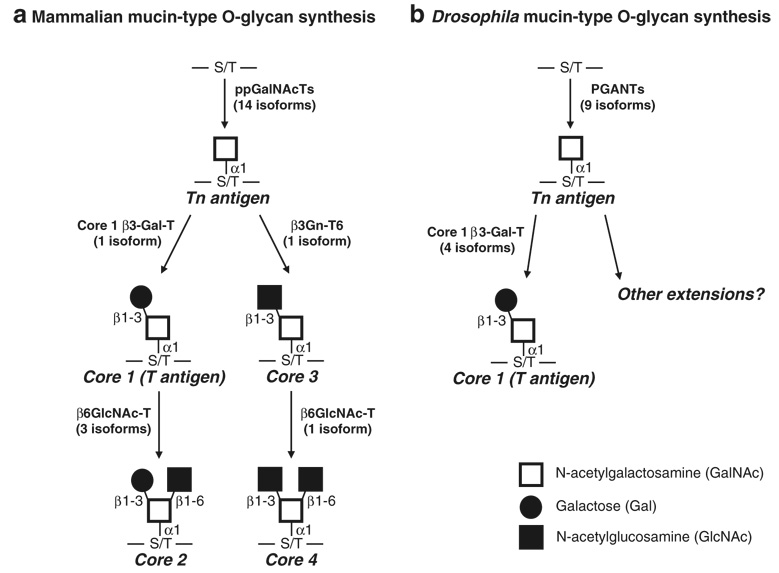
Biosynthesis of the most common mucin-type O-glycans in mammals (a) and Drosophila melanogaster (b). The initiation of mucin-type O-glycosylation is catalyzed by the addition of GalNAc onto the hydroxyl group of either serine or threonine in protein substrates destined to be membrane-bound or secreted, forming the Tn antigen (Tn Ag). Enzymes responsible for the synthesis of core 1 (T antigen), core 2, core 3 and core 4 structures are shown. Additional extensions of mammalian O-glycans and modifications with sialic acid or fucose are not shown. There is currently no evidence for sialylated O-glycans in Drosophila. ppGalNAcTs or PGANTs, UDP-N-acetylgalactosamine: polypeptide N-acetylgalactosaminyltransferases; Core 1 β3-Gal-T, core 1 β1-3-galactosyltransferase; β3Gn-T6, β1-3 N-acetylglucosaminyltransferase; β6GlcNAc-Ts, β1-6 N-acetylglucosaminyltransferase
Enzymes responsible for the synthesis of mucin-type O-glycans
O-glycosylation is initiated by a family of enzymes
Mucin-type O-linked glycosylation is initiated by a family of enzymes known as the UDP-N-acetylgalactosamine: polypeptide N-acetylgalactosaminyltransferases (ppGal-NAcTs or ppGaNTases in mammals and PGANTs in Drosophila; EC 2.4.1.41) (reviewed in [2, 3]) which are responsible for catalyzing the transfer of GalNAc from the nucleotide sugar UDP-GalNAc to the hydroxyl group of either serine or threonine in protein substrates destined to be membrane-bound or secreted (Fig. 1). There are 15 reports of distinct mammalian ppGalNAcTs in the literature, 14 of which have been functionally characterized (reviewed in [2, 3]) (Table 1). There is now evidence for three additional active isoforms, with homology searches revealing the potential for a total of 20 isoforms in human and 18 isoforms in mouse (J. Raman, T. Fritz and L. A. Tabak, personal communication). Fewer family members are found in the Drosophila and C. elegans; thus far, the fly has nine functional transferases (derived from nine distinct genes), with as many as 12 genes total [4–6]; the worm has five cDNAs that encode functional transferases (derived from four genes), with as many as nine transferase-encoding genes total [7, 8]. All isoforms are type II transmembrane proteins with a short N-terminal cytoplasmic region, a hydrophobic transmembrane region, a variable length stem region and a conserved catalytic region. Biochemical analyses have revealed unique as well as overlapping substrate preferences amongst isoforms [4, 5, 9–14] (reviewed in [2]). Additionally, there is a hierarchy of action within this family, with certain members acting as “initiating” transferases, transferring GalNAc to unmodified substrates, while other members act only on previously glycosylated substrates (“glycopeptide transferases”) [15–18].
Table 1
Published reports of mammalian ppGalNAcTs
ppGalNAcT isoform | Species | Activity demonstrated? | Reference |
---|---|---|---|
ppGalNAc-T1 | Bovine | Yes | Homa et al. [83], Hagen et al. [84] |
ppGalNAc-T1 | Rattus norvegicus | Yes | Hagen et al. [85] |
ppGalNAc-T1 | Homo sapiens | Yes | White et al. [86] |
ppGalNAc-T1 | Mus musculus | Yes | Hagen et al. [87] |
ppGalNAc-T1 | Porcine | Yes | Yoshida et al. [88] |
ppGalNAc-T2 | Homo sapiens | Yes | White et al. [86] |
ppGalNAc-T3 | Homo sapiens | Yes | Bennett et al. [89] |
ppGalNAc-T3 | Mus musculus | Yes | Zara et al. [9] |
ppGalNAc-T4 | Mus musculus | Yes | Hagen et al. [87] |
ppGalNAc-T4 | Homo sapiens | Yes | Bennett et al. [90] |
ppGalNAc-T5 | Rattus norvegicus | Yes | Ten Hagen et al. [13] |
ppGalNAc-T6 | Homo sapiens | Yes | Bennett et al. [10] |
ppGalNAc-T7a | Rattus norvegicus | Yes | Ten Hagen et al. [15] |
ppGalNAc-T7 | Homo sapiens | Yes | Bennett et al. [16] |
ppGalNAc-T8b | Homo sapiens | No | White et al. [91] |
ppGalNAc-T9 | Homo sapiens | Yes | Toba et al. [92], Zhang et al. [93] |
ppGalNAc-T10c | Rattus norvegicus | Yes | Ten Hagen et al. [17] |
ppGalNAc-T10 | Homo sapiens | Yes | Cheng et al. [94] |
ppGalNAc-T11 | Homo sapiens | Yes | Schwientek et al. [5] |
ppGalNAc-T12 | Homo sapiens | Yes | Guo et al. [95] |
ppGalNAc-T13d | Mus musculus | Yes | Hennet et al. [96], Zhang et al. [93] |
ppGalNAc-T13 | Homo sapiens | Yes | Zhang et al. [93] |
ppGalNAc-T14 | Homo sapiens | Yes | Wang et al. [97] |
ppGalNAc-T15 | Homo sapiens | Yes | Cheng et al. [98] |
(there is evidence for five additional human isoforms, three of which have confirmed transferase activity; J. Raman, T. Fritz and L. A. Tabak, personal communication)
Phylogenetic analysis reveals a high degree of sequence conservation among ppGalNAcTs across species, defining orthologous groups or pairs [4, 5]. These orthologues display not only sequence conservation but functional conservation as well. In vitro studies revealed that orthologues share similar substrate preferences [4, 5] and preferred sites of GalNAc addition within those substrates [4]. This functional conservation suggests unique biological roles for members of this family that have been maintained over the course of evolution.
Studies examining the expression of each family member revealed unique spatial and temporal gene expression patterns during both mammalian and fly development [6, 19]. Some members are expressed widely in many tissues during many stages of development while others are expressed in a very restricted subset of tissues at specific times [6, 19]. Collectively, the unique spatial and temporal expression patterns, along with conserved substrate preferences and hierarchy of action within this family suggest a complex and highly regulated process governing the acquisition of mucin-type O-glycans.
Extension of the GalNAcα1-S/T
The most abundant modification of the core GalNAcα1-S/T is known as the core 1 or T antigen structure (Galβ1-3GalNAcα1-S/T) (Fig. 1). There is one core 1 β1-3 galactosyltransferase (core 1 β3-Gal-T) in mammals responsible for the addition of a galactose in a β1-3 linkage to GalNAc [20, 21]; the gene encoding this enzyme is widely expressed in mammals, especially in the liver, kidney, heart and placenta [20, 21]. This gene is evolutionarily-conserved, with one core 1 β3-Gal-T being functionally described in C. elegans [22] and at least four functional core 1 β3-Gal-Ts described in Drosophila [23]. Like its mammalian counter-part, the C. elegans core 1 β3-Gal-T gene is expressed throughout all developmental stages and in all cells, suggesting a crucial requirement for this modification [22]. The complete developmental expression pattern of the multiple Drosophila core 1 β3-Gal-T genes is not currently known, although two are expressed in the developing salivary glands and one is expressed in the amnioserosa, a tissue involved in cell migration and adhesion during development [23]. Interestingly, the activity of the mammalian core 1 β3-Gal-T enzyme requires the coexpression of a molecular chaperone, Cosmc [24]. Cosmc aids in folding and stability of the core 1 β3-Gal-T enzyme, ensuring proper localization to the Golgi apparatus [24, 25]. Mutations in Cosmc result in the loss of core 1 β3-Gal-T from the Golgi apparatus and loss of core 1 activity [24, 25]. In contrast to the mammalian enzyme, the C. elegans and Drosophila core 1 β3-Gal-Ts do not appear to require a chaperone for activity [22, 23].
The core 3 modification (GlcNAcβ1-3GalNAcα-S/T) is catalyzed by the β1-3 N-acetylglucosaminyltransferase (β3Gn-T6) (Fig. 1). There is one β3Gn-T6 in mammals that is expressed primarily in stomach, small intestine and [26, 27]. Orthologues responsible for synthesizing the core 3 structure have not been identified in C. elegans or Drosophila as yet.
The core 2 and core 4 O-glycan branching (Fig. 1) of the core 1 and core 3 structures, respectively, is catalyzed by the β1-6 N-acetylglucosaminyltranferases (β6GlcNAc-Ts). There are three core 2 transferases identified to date in mammals [28–32] (reviewed in [33]), two of which catalyze the formation of core 2 structures and one that is responsible for forming both core 2 and core 4 branched structures (Fig. 1). The expression pattern of each gene is distinct, suggesting unique biological roles for each enzyme. The T-1 isoform is expressed ubiquitously [28], while T-2 (which is responsible for core 2 and core 4 synthesis) is expressed in the kidney, pancreas, stomach, intestines and colon [30, 31]; T-3 is expressed in the thymus and T cells [32], Less is known about the existence of β6GlcNAc-Ts responsible for this branched structure in C. elegans or Drosophila. Thus far no functional β6GlcNAc-Ts have been identified in Drosophila but a gene homologous to β6GlcNAc-T has been identified in C. elegans (gly-1) [34]. It is of note that nomucin-type O-glycans carrying the core 2, core 3 or core 4 structures or sialic acid were detected in a recent mass spectroscopy analysis of Drosophila O-glycans [35]. However, evidence for the presence of glucuronic acid (GlcA) on the core 1 O-glycans of Drosophila (M. Tiemeyer, personal communication) and C. elegans has been reported [36]. Additionally, novel O-linked structures containing β-Glc are also observed in C. elegans [36].
Each of the above mentioned branches can be further extended by the addition of Gal and GlcNAc and further modified by fucosylation and sialylation. The enzymes responsible for these additional modifications and other core structures will not be discussed in this review.
O-glycan function
O-glycosylation during development
A crucial role for mucin-type O-glycans during development was first demonstrated in Drosophila. Mutations in member of the pgant family (pgant35A) were shown to abrogate enzymatic activity and result in recessive lethality, with death occurring throughout development [5, 37]. This was the first example that mucin-type O-glycans were required for viability in any organism.
Studies of mice deficient for the core 1 β3-Gal-T gene (T-syn−/−), provided more detailed information regarding the role of O-glycans in specific organ systems during development [38, 39]. T-syn−/− mice displayed defective angiogenesis and died from fatal brain hemorrhages by embryonic day 14 (E14) [38]. Specifically, vasculature formation was irregular in these mice, displaying distorted capillary lumens, and detachment of endothelial cells from periocytes and the extracellular matrix. These studies revealed a role for O-glycans in vascular system/tubular system development, possibly via influencing cell–cell and/or cell–ECM interactions required for proper tube/diffusion barrier formation.
Studies examining hypomorphic mutations in the core 1 β3-Gal-T gene provided additional evidence for the role of O-glycans in tubular architecture and function [39]. In this study, most core 1 β3-Gal-T hypomorphic mice died within 200 days due to compromised renal function. The kidneys of these mice displayed distorted glomeruli and proximal tubules. The authors propose that the improper glycosylation of podocalyxin (podx1), a glycoprotein normally present on the surface of the renal podocyte foot process is responsible for the disruption in kidney function. podx1−/− mice die embryonically, with defective kidney formation [40], lending support for the role of this glycoprotein in the defects seen in the core 1 β3-Gal-T hypomorph. The molecular nature of how the O-glycans on podx1 are mediating these biological effects remains to be determined but it is suggested to be due to cell adhesion defects [39].
The core 1 β3-Gal-T hypomorphic mice also displayed thrombocytopenia [39]. The authors propose that abnormal glycosylation of GpIbα, a protein present on platelets and involved in platelet adhesion and function, is responsible for the thrombocytopenia observed. It is also possible that loss of the core 1 structure may result in self-reactivity and clearance of those cells now displaying the Tn Ag (see Tn syndrome below).
Additional evidence for the specific role for O-glycans in tubulogenesis was further supported by recent work in Drosophila. Examination of O-glycans present during Drosophila development revealed an abundance along the apical and luminal regions of developing tubular organs (Fig. 2) [41]. O-glycans detected by lectins and antibodies were present along the apical surfaces of the developing hindgut, foregut, salivary glands, malpighian tubules (the functional equivalent of the kidney) and the tracheal system [41]. Mutations in one member of the pgant family in Drosophila (pgant35A) resulted in abnormal tracheal tube formation. Specifically, tracheal tubes of mutants showed loss of apical and luminal O-glycans and were irregular in diameter and shape (Fig. 3) [42]. Further examination revealed a loss of apicobasal polarity and diffusion barrier formation in the pgant35A mutants. An increase in cytoplasmic vesicular staining of proteins destined for the apical and luminal surfaces was also seen in the mutants [42]. Based on these results, it was proposed that O-glycosylation is responsible for proper apical/luminal composition, apico-basal polarity and diffusion barrier formation in the tracheal system by influencing the apical delivery of proteins/glycoproteins [42]. Roles for O-glycans in trafficking, delivery and/or maintenance of proteins at the cell surface has also been suggested previously in a number of cell culture studies [43–49]. Taken together, these studies suggest a conserved role for O-glycans in the molecular events governing tubulogenesis across diverse organ systems and species, possibly by influencing trafficking and stability of crucial proteins involved in tube architecture and function.
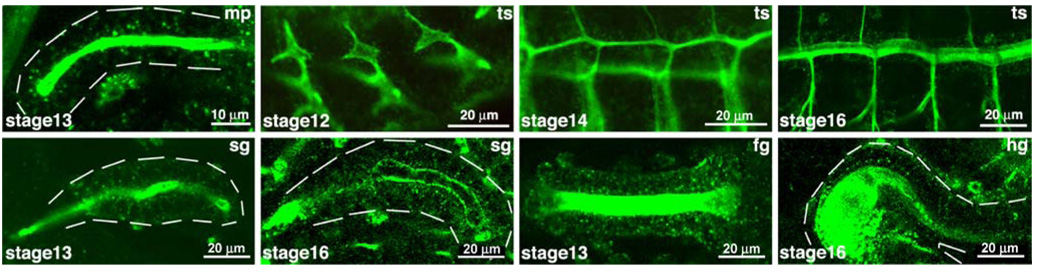
Tn antigen (GalNAcα-S/T) expression in developing tubular tissues during Drosophila embryogenesis. The dashed white lines outline the outer boundaries of each organ shown. Note the intense apical and luminal presence of the O-glycans in each organ. Stages are shown in the lower left corner of each panel. fg foregut, hg hindgut, mp malpighian tubules, sg salivary gland, ts tracheal system. Adapted from Glycobiology, 17, 820–827 (2007) by copyright permission of the Oxford University Press
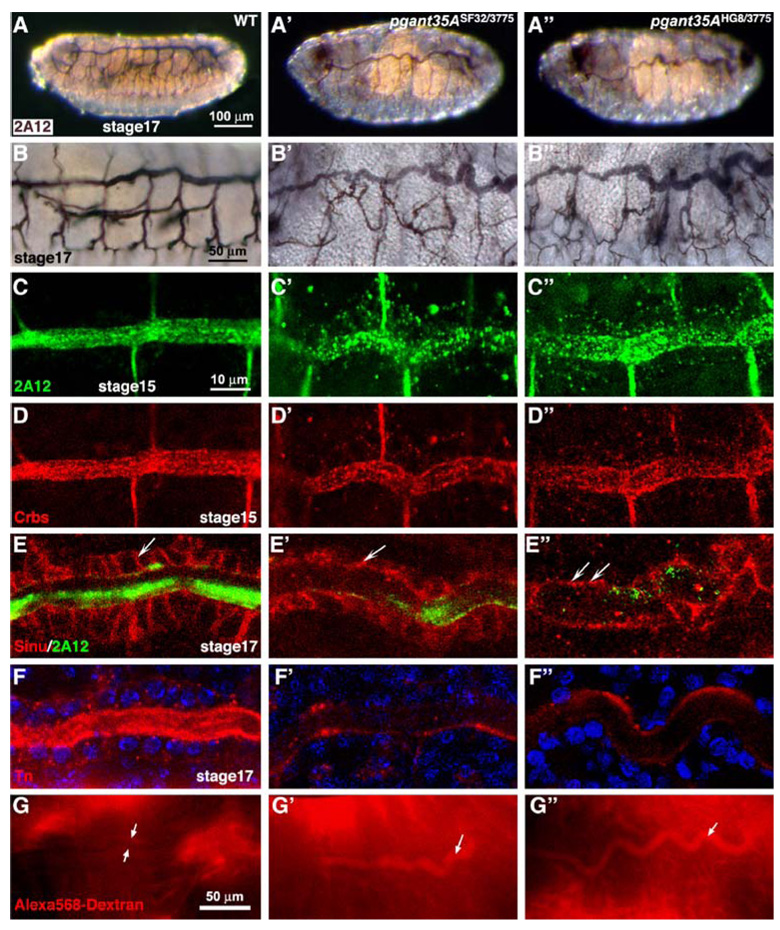
A PGANT O-glycosyltransferase is required for proper tracheal development and diffusion barrier formation during Drosophila embryogenesis. Wild-type (A–G), pgant35ASF32/3775 (A′–G′), pgant35AHG8/3775 (A″–G″). (A) Tracheal luminal marker 2A12 staining shows the normal development of the tracheal system in wild type (WT) embryos at stage 17 and abnormal tracheal tube formation at stage 17 for pgant35ASF32/3775 (A′) and pgant35AHG8/3775 (A″) homozygous maternal/zygotic (m/z) mutants. Magnified views (×40) are shown for wild type (B), pgant35ASF32/3775 (B′) and pgant35AHG8/3775 (B″) m/z mutants. Tracheal staining with the luminal marker 2A12 (green) at stage 15 in pgant35ASF32/3775 (C′) and pgant35AHG8/3775 (C″) m/z mutants reveals decreased luminal staining and increased cytoplasmic staining relative to wild type (WT) (C). Similar results are seen with Crbs staining at stage 15 in WT (D), pgant35ASF32/3775 (D′) and pgant35AHG8/3775 (D″) m/z mutants. At stage 17, the septate junction protein, Sinuous (Sinu), is mislocalized in the pgant35A m/z mutant trachea (E′ and E″) relative to wild type (E), as the mutants show reduced lateral localization and increased apical distribution (arrows in panels E–E″). Tn Ab staining in the apical and luminal regions of the trachea of stage 17 embryos (F–F″) is lost in pgant35ASF32/3775 (F′) and pgant35AHG8/3775 (F″) m/z mutants relative to wild type (F). Stage 16– 17 embryos were injected with 10 kD dextran dye and visualized after 30 min. Wild type embryos showed no dye leakage into the tracheal system (between arrows in G), whereas pgant35A homozygous m/z mutants had significant dye present in the tracheal tubes (arrows in G′ and G″), indicating loss of paracellular diffusion barrier formation. Scale bar: 100 µm for A–A″; 50 µm for B–B″; 10 µm for C–F″; 50 µm for G–G″. Embryos are oriented such that anterior is to the left and dorsal is up. Adapted from The Journal of Biological Chemistry, 282, 606–614 (2007) by copyright permission of The American Society for Biochemistry and Molecular Biology, Inc
Most recently, studies in Xenopus have implicated mucin-type O-glycosylation in TGF-β signaling during development [50]. This study demonstrated that over-expression of a putative ppGalNAcT caused inhibition of both Activin and BMP signaling during Xenopus embryogenesis. The authors propose that putative O-glycans on the ActR-IIB receptor may be responsible for the effects seen, by influencing the formation of heteromeric receptor complexes. However, direct demonstration of transferase activity, as well as the receptors, ligands or other proteins that are normally O-glycosylated in vivo remains to be determined.
O-glycosylation and disease
Recently studies have highlighted the involvement of mucin-type O-glycosylation in a number of diseases. One such example is familial tumoral calcinosis, a recessively inherited condition characterized by hyperphosphatemia, increased bone density and the development of ectopic calcified masses [51]. Studies of affected families revealed that this disease is the result of mutations in the glycosyltransferase ppGalNAc-T3 [51–53]. In other affected groups, mutations in the phosphate-regulating hormone FGF23 cause the same condition [54], suggesting a link between FGF23 and O-glycosylation. Patients with mutations in ppGalNAc-T3 have increased levels of cleaved, inactive FGF23 and a paucity of intact, active FGF23 in their blood [52, 55]. In vitro studies have demonstrated that ppGalNAc-T3 can glycosylate FGF23 at a serine near a protease cleavage site, leading to the hypothesis that O-glycans normally present on FGF23 confer protection from proteolysis and aid in the proper secretion of intact FGF23 [56]. In the case of ppGalNAc-T3 mutations, it is proposed that under-glycosylated FGF23 is prematurely cleaved and inactivated, leading to improper regulation of phosphate levels and the resultant phenotypic consequences in patients. These data suggest a role for O-glycans in regulating proteolytic events necessary for proper phosphate regulation and homeostasis in vivo.
Additional evidence for the role of O-glycans in disease comes from examining the molecular basis of Tn syndrome, a rare autoimmune disorder that results in thrombocytopenia and hemolytic anemia [57]. Mutations in Cosmc (the chaperone for the core 1 β3-Gal-T) were shown to be responsible for Tn syndrome in a subset of hematopoetic cells [24]. Somatic mutations in Cosmc result in the loss of the core 1 structure on a subpopulation of hematopoetic cells, exposing the immunoreactive Tn Ag on these cells and thus leading to hemolysis.
IgA nephropathy (IgAN) is also thought to have its root in aberrant O-glycosylation. This disease is characterized by abnormal deposition of IgA1 in the glomerular mesangium, resulting in glomerulonephritis (reviewed in [58]). IgA1 is normally heavily O-glycosylated in the hinge region with sialylated core 1 structures [59–62]. However, mesangial IgA1 in IgAN patients is aberrantly O-glycosylated, showing a loss of the core 1 structure [60, 61, 63–66]. It is thought that the truncated O-glycans present on IgA1 can cause aggregation of these molecules, forming IgA immune complexes that would promote glomerular inflammation. Current studies are focused on identifying the molecular basis for the changes in IgA O-glycosylation. While decreased Cosmc gene expression in IgAN patients has been reported by one group [67], another study failed to find a significant difference in core 1 β3-Gal-T or Cosmc enzymatic activity or gene expression [68]. Thus far, mutations in the genes responsible for core 1 formation have not been found in patient populations, suggesting that the primary defect may reside in another component of the O-glycosylation machinery.
Recent genome-wide association studies cataloguing loci that influence plasma lipid levels in humans have identified a ppGalNAcT (ppGalNAc-T2 or GALNT2) as one of a number of genes associated with variations in high density lipoprotein (HDL) levels [69, 70]. ppGalNAc-T2 variants may contribute to the heritable component of lipid profiles by glycosylating proteins involved in lipid metabolism, potentially influencing their stability or function. If verified, future therapeutics to regulate lipid levels and treat cardiovascular disease may include those directed at O-linked glycosylation.
Finally, the association between altered glycosylation patterns and tumor formation is well documented [71–74]. Recent studies have begun to directly interrogate this relationship by altering the activity of the glycosyltransferase responsible for the synthesis of the core 3 structure. In one study where β3Gn-T6 activity was found to be down-regulated in colon carcinomas, overexpression of β3Gn-T6 in these cells decreased their metastatic ability [75]. In another study, mice deficient in the β3Gn-T6 gene showed an increase in intestinal barrier permeability and became highly susceptible to induced colitis and colorectal tumors [76]. This study not only further supports the role of O-glycans in proper diffusion barrier function, but suggests that a causal role exists between loss of normal O-glycosylation patterns and tumor formation/progression.
O-glycosylation and the immune system
Many studies in mice have highlighted the importance of diverse types of glycans in immune system function. Here, we highlight recent work involving the deletion of specific glycosyltransferases involved in mucin-type O-glycan biosynthesis. Mice deficient in one of the core 2 transferases (Core2GlcNAcT-1) displayed altered inflammatory responses combined with decreased neutrophil infusion [77, 78]. Leukocytes from these mice displayed decreased rolling on P-, E- and l-selectin [78], highlighting a role for core 2 O-glycans in selectin adhesion as it relates to immune cell function. Likewise, deletion of a glycosyltransferase that extends the core 1 structure (Core1-β3GlcNAcT) as well as double mutants of Core1-β3GlcNAcT and Core2GlcNAcT-1 displayed reduced lymphocyte homing, although compensation was provided by selectin ligands present on N-glycans as well [79]. Work by a number of groups has also demonstrated the importance of fucosylation [80] and sialylation [81], two common modifications found on mucin-type O-glycans, in lymphocyte homing and homeostasis, respectively.
Recent work examining mice deficient in a member of the initiating ppGalNAcT family (ppGalNAc-T1) has demonstrated a role for O-glycosylation in blood clotting and immune system function [82]. Mutant mice had reduced plasma clotting factors and a concomitant increase in clotting time. Additionally, animals displayed decreased B cell homing to lymph nodes, the result of significant reductions in l-selectin ligands on high endothelial venules. The decreased neutrophil recruitment observed was the result of decreases in E- and P-selectin ligands on these cells, resulting in an alteration in their adhesive properties. Alterations in germinal center formation via increased apoptosis of B cells normally present there resulted in decreased IgG production, indicating a crucial role for ppGalNAc-T1 in the regulation of B cell maturation and antibody production. While the exact molecular mechanisms by which O-glycans mediate these effects remain to be determined, these studies highlight the importance of O-linked glycans in many tissues and cells involved in proper vascular function and humoral immunity.
Conclusion
Recent advances in deciphering the machinery controlling O-glycosylation in many experimental systems described herein have aided our understanding of the in vivo role of mucin-type O-glycans. Continued efforts to elucidate the molecular role of O-glycosylation in both development and disease in many diverse organisms will highlight conserved processes in which glycosylation plays key regulatory roles, with the eventual goal of designing better strategies to improve human health.
Acknowledgments
We would like to thank the many members of the community who have contributed to the work mentioned herein. We would like to thank Dr. Lawrence Tabak for carefully reading this manuscript. Our laboratory is supported by the Intramural Research Program of the NIDCR, NIH.
Abbreviations
ppGaNTase or | UDP-GalNAc:polypeptide |
ppGalNAcT or pgant | N-acetylgalactosaminyltransferase |
GalNAc | N-acetylgalactosamine |
Gal | galatose |
GlcNAc | N-acetylglucosamine |
GlcA | glucuronic acid |
Footnotes
Open Access This article is distributed under the terms of the Creative Commons Attribution Noncommercial License which permits any noncommercial use, distribution, and reproduction in any medium, provided the original author(s) and source are credited.
References
Full text links
Read article at publisher's site: https://doi.org/10.1007/s10719-008-9162-4
Read article for free, from open access legal sources, via Unpaywall:
https://link.springer.com/content/pdf/10.1007/s10719-008-9162-4.pdf
Citations & impact
Impact metrics
Citations of article over time
Alternative metrics

Discover the attention surrounding your research
https://www.altmetric.com/details/110386966
Article citations
Substrate O-glycosylation actively regulates extracellular proteolysis.
Protein Sci, 33(8):e5128, 01 Aug 2024
Cited by: 0 articles | PMID: 39074261 | PMCID: PMC11285871
Polypeptide N-acetylgalactosaminyltransferase (GalNAc-T) isozyme surface charge governs charge substrate preferences to modulate mucin type O-glycosylation.
Glycobiology, 33(10):817-836, 01 Oct 2023
Cited by: 4 articles | PMID: 37555669 | PMCID: PMC10629720
Identification of the glycopeptide epitope recognized by a protective Cryptosporidium monoclonal antibody.
Infect Immun, 91(10):e0027523, 19 Sep 2023
Cited by: 0 articles | PMID: 37725059 | PMCID: PMC10580954
Metabolic Glycoengineering: A Promising Strategy to Remodel Microenvironments for Regenerative Therapy.
Stem Cells Int, 2023:1655750, 13 Feb 2023
Cited by: 1 article | PMID: 36814525 | PMCID: PMC9940976
Review Free full text in Europe PMC
Glycoconjugates: Synthesis, Functional Studies, and Therapeutic Developments.
Chem Rev, 122(20):15603-15671, 29 Sep 2022
Cited by: 16 articles | PMID: 36174107 | PMCID: PMC9674437
Review Free full text in Europe PMC
Go to all (117) article citations
Data
Similar Articles
To arrive at the top five similar articles we use a word-weighted algorithm to compare words from the Title and Abstract of each citation.
Mucin-type O-glycosylation during development.
J Biol Chem, 288(10):6921-6929, 17 Jan 2013
Cited by: 159 articles | PMID: 23329828 | PMCID: PMC3591602
Review Free full text in Europe PMC
The chemistry and biology of mucin-type O-linked glycosylation.
Bioorg Med Chem, 13(17):5021-5034, 01 Sep 2005
Cited by: 152 articles | PMID: 16005634
Review
O-glycoprotein biosynthesis: site localization by Edman degradation and site prediction based on random peptide substrates.
Methods Mol Biol, 842:81-108, 01 Jan 2012
Cited by: 2 articles | PMID: 22259131
Dissecting the biological role of mucin-type O-glycosylation using RNA interference in Drosophila cell culture.
J Biol Chem, 285(45):34477-34484, 31 Aug 2010
Cited by: 17 articles | PMID: 20807760 | PMCID: PMC2966062
Funding
Funders who supported this work.
Intramural NIH HHS (1)
Grant ID: Z01 DE000713-03