Abstract
Free full text

Programmed necrosis induced by asbestos in human mesothelial cells causes high-mobility group box 1 protein release and resultant inflammation
Abstract
Asbestos carcinogenesis has been linked to the release of cytokines and mutagenic reactive oxygen species (ROS) from inflammatory cells. Asbestos is cytotoxic to human mesothelial cells (HM), which appears counterintuitive for a carcinogen. We show that asbestos-induced HM cell death is a regulated form of necrosis that links to carcinogenesis. Asbestos-exposed HM activate poly(ADP-ribose) polymerase, secrete H2O2, deplete ATP, and translocate high-mobility group box 1 protein (HMGB1) from the nucleus to the cytoplasm, and into the extracellular space. The release of HMGB1 induces macrophages to secrete TNF-α, which protects HM from asbestos-induced cell death and triggers a chronic inflammatory response; both favor HM transformation. In both mice and hamsters injected with asbestos, HMGB1 was specifically detected in the nuclei, cytoplasm, and extracellular space of mesothelial and inflammatory cells around asbestos deposits. TNF-α was coexpressed in the same areas. HMGB1 levels in asbestos-exposed individuals were significantly higher than in nonexposed controls (P < 0.0001). Our findings identify the release of HMGB1 as a critical initial step in the pathogenesis of asbestos-related disease, and provide mechanistic links between asbestos-induced cell death, chronic inflammation, and carcinogenesis. Chemopreventive approaches aimed at inhibiting the chronic inflammatory response, and especially blocking HMGB1, may decrease the risk of malignant mesothelioma among asbestos-exposed cohorts.
In the United States, asbestos causes ~2,000–3,000 malignant mesothelioma (MM) deaths per year and contributes to an even larger number of lung carcinomas because asbestos has a synergistic carcinogenic effect with cigarette smoke. The latency of 30–50 y from the time of exposure to tumor development could potentially allow time for intervention to block the presently unclear mechanism(s) that trigger asbestos-induced carcinogenesis (1, 2).
Asbestos refers to a family of mineral fibers that includes crocidolite, often considered the most oncogenic type. Because asbestos does not induce malignant transformation of primary human mesothelial cells (HM) directly, indirect mechanisms of carcinogenesis have been investigated. Inhaled asbestos fibers become entrapped in the lung, and some migrate through the lymphatics to the pleura. The deposition of asbestos in the lung and pleura causes chronic inflammation that may lead to lung fibrosis and reactive mesothelial hyperplasia; it is around these areas that MM and lung cancer develop (1). Indeed, cancer often arises in the setting of chronic inflammation (3, 4), and it has been suggested that asbestos-induced inflammation might be somehow linked to asbestos carcinogenesis (5, 6).
The mechanisms that trigger the chronic inflammatory response seen in the lungs of asbestos-exposed individuals and in many MM patients are unknown. Macrophages play an important role in this process by releasing mutagenic reactive oxygen species (ROS) and cytokines that support inflammation (5–9). Among these cytokines, TNF-α has been identified as a critical mediator of the pathogenesis of asbestos-related disease. TNF-α has been linked to tumor promotion (10, 11), fibrosis and asbestosis (10, 12, 13), asbestos carcinogenesis (14), and MM (10).
Asbestos is cytotoxic. Most HM exposed to asbestos die within 24–48 h (14, 15). The mechanisms of asbestos-induced HM cell death, and the possible link between cytotoxicity and carcinogenesis, are unclear. It is generally assumed that asbestos causes apoptosis in rat, rabbit, and human mesothelial cells (16–17). These reports predate recent discoveries that allow us to distinguish more precisely between apoptosis and necrosis, the two major distinct forms of cell death. The apoptotic and necrotic pathways ensure that cells with irreparable damage are eliminated. Apoptosis is an energy-dependent process and does not cause inflammation. The hallmarks of apoptotic cell death are internucleosomal DNA fragmentation and caspase activation, which leads to poly(ADP ribose) polymerase (PARP) cleavage. PARP is a nuclear enzyme with DNA nick sensor function. When activated by DNA strand breaks, PARP cleaves NAD+ into nicotinamide and ADP ribose, and catalyzes the formation of poly-ADP ribose (PAR) polymers. Initially, PARP was considered a marker of DNA repair and apoptosis. Subsequently, it was found that cells exposed to DNA-damaging agents could enter one of the following three pathways: (i) when PARP is activated by mild genotoxic stimuli, it facilitates DNA repair; (ii) more severe DNA damage induces apoptosis, during which caspase inactivates PARP by cleaving it into two fragments (p89 and p24); and (iii) extensive DNA breakage triggered by a massive degree of oxidative or nitrosative stress causes PARP overactivation, which depletes ATP, promoting cell necrosis (18, 19).
Necrosis, unlike apoptosis, causes inflammation (20–23). Necrosis was initially viewed as an uncontrolled form of cell death caused by overwhelming extrinsic injury. It was then shown that necrosis can occur as a consequence of acute ATP depletion (24–26). Recent findings indicate that necrosis can also be a regulated event, which involves the activation of PARP, depletion of intracellular ATP, and release of high-mobility group box 1 protein (HMGB1), a factor that starts and promotes inflammation (20–23, 25–28).
In healthy cells, HMGB1 is found in the nucleus, where it plays multiple roles in DNA transcription, replication, and recombination (29). In apoptotic cells, HMGB1 is selectively retained in the nucleus by the condensed chromatin. Because HMGB1 is not released, apoptosis does not induce inflammation. In contrast, during programmed necrosis, overactivation of PARP promotes the translocation of HMGB1 from the nucleus to the cytosol, and then to the extracellular space, where HMGB1 triggers the inflammatory response that characterizes this type of cell death (30, 31). Macrophages participate in this inflammatory response and can actively secrete HMGB1 in the extracellular space, promoting chronic inflammation (32). HMGB1 is the archetypal damage associated molecular pattern (DAMP): molecules that originate from within the cell, and are exposed only as a signal of “danger” or “alarm.” As expected for a DAMP, extracellular HMGB1 (i) conveys the message of danger to other cells, (ii) triggers inflammation and innate immunity, (iii) plays a role in establishing immunological memory of the adverse event, and (iv) helps orchestrate tissue repair and healing (26).
In this study, we investigated the mechanism of asbestos-caused HM cell death and discovered a critical link between asbestos cytotoxicity, HMGB1 release, and carcinogenesis.
Results
Asbestos-Induced HM Cell Death Is Caspase Independent.
HM were exposed to increasing amounts of crocidolite asbestos (0-10 μg/cm2) or to actinomycin D (0.1 μM) for 24 h. We found a dose-dependent effect of asbestos in inducing HM death, ranging from ~10% (0.5 μg/cm2) to 20–40% (1–2.5 μg/cm2) to 50% (5.0 μg/cm2) to more than 50% (10 μg/cm2) within 24 h after asbestos exposure (14, 15). We analyzed caspase-3 activation and PARP cleavage, two hallmarks of apoptosis, in HM exposed to increasing doses of asbestos for 24 h by Western blot. The results showed that asbestos did not induce caspase-3 activation (Fig. S1A) or PARP cleavage (Fig. 1A).
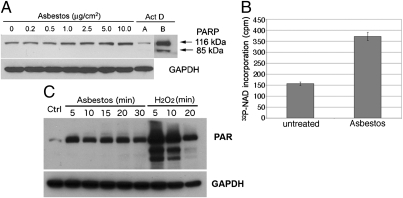
Asbestos induces PARP activation in HM. (A) Increasing amounts of asbestos induced a parallel increase in PARP levels, without inducing PARP cleavage. HM were exposed to asbestos at 0–10 μg/cm2 or to actinomycin D (0.1 μM) for 24 h. Whole-cell extracts (30 μg) were analyzed by Western blot with anti-PARP monoclonal antibody. GAPDH was used as loading control. Actinomycin D (Act D, control for apoptosis) induces PARP cleavage. Act D, lane B: Film was overexposed to show cleavage of PARP more clearly. No PARP cleavage was seen after overexposure of the film in any of the asbestos lanes. (B) PARP activity is induced by asbestos. Representative results of three separate experiments. HM were exposed to 5.0 μg/cm2 crocidolite for 24 h. PARP activity was measured by incorporation of radiolabeled NAD using a PARP assay kit. PARP activity was induced ~2.4-fold following asbestos exposure. (C) Amounts of PAR were increased following asbestos exposure, confirming that PARP activity is induced by asbestos. PARP activity was detected measuring PAR by Western blot. HM exposed to crocidolite (5 μg/cm2) or to 1 mM H2O2 (positive control for necrosis and PARP activation) for the indicated times.
To verify these findings, we inhibited the caspase pathway using the pan-caspase inhibitor z-VAD-fmk. We used lactate dehydrogenase (LDH) released from the damaged cells into the supernatant as a measure of cytotoxicity. We found that z-VAD-fmk did not reduce asbestos-induced cytotoxicity, although it did inhibit apoptotic cell death induced by actinomycin D (Fig. S1B), which suggested that asbestos-induced cell death was caspase independent. Together these results indicate that apoptosis is not the major pathway in asbestos-induced cell death.
Asbestos-Induced HM Cell Death Is PARP Dependent.
PARP was not cleaved in HM undergoing cell death following asbestos exposure; instead the levels of full-length PARP were markedly increased with increasing amounts of asbestos exposure (Fig. 1A). Real-time PCR experiments indicated that PARP mRNA was also induced by asbestos (Fig. S2). PARP activity, measured as conversion of radiolabeled NAD substrate, or production of poly-ADP ribose (PAR), was induced by asbestos treatment (Fig. 1 B and C).
To verify that asbestos-caused HM cell death was PARP dependent, we pretreated HM with the PARP inhibitor 3-aminobenzamide (3-ABA) for 1 h before asbestos exposure. 3-ABA protected HM from asbestos-induced cell death. Viability of HM was significantly increased by pretreatment with 3-ABA before asbestos exposure (Fig. 2A).
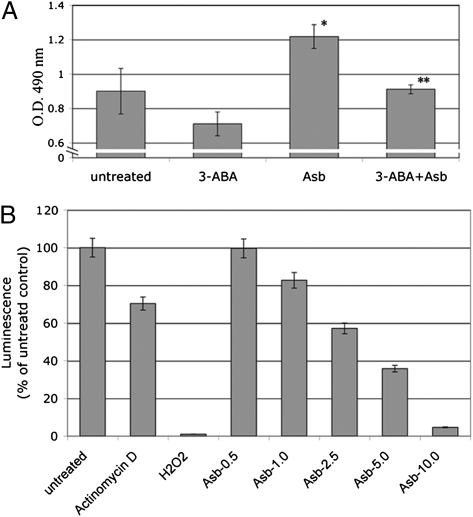
Asbestos-induced HM death is PARP dependent and causes ATP depletion. (A) PARP inhibitor 3-ABA decreases asbestos cytotoxicity. HM were incubated with or without 3-ABA (0.5 mM) for 1 h before asbestos exposure (5 μg/cm2). Representative results of three separate experiments are shown. Cytotoxicity was detected using the LDH assay. 3-ABA decreased asbestos cytotoxicity and protected HM from asbestos-induced cell death. *Significantly different compared with HM without asbestos exposure. **Significantly different compared with HM exposed to asbestos without 3-ABA pretreatment (P < 0.05). (B) Asbestos induces ATP depletion in HM. HM were treated with actinomycin D (0.1 μM, positive control for apoptosis), H2O2 (200 μM, positive control for necrosis) or with asbestos (0.5–10 μg/cm2) for 24 h. Cellular ATP was measured by a bioluminescence assay. Increasing amounts of asbestos significantly induced ATP depletion. Each column represents the average of three separate experiments.
We determined the levels of ATP in HM exposed to increasing doses of asbestos by bioluminescence assay. We found significant ATP depletion in HM exposed to asbestos and to H2O2 (positive control for necrosis, Fig. 2B). In contrast, ATP levels remained high in HM following actinomycin D treatment, which triggers apoptosis (Fig. 2B). These findings indicate that asbestos induces and activates PARP, which in turn promotes ATP depletion and cell necrosis.
Asbestos-Induced HM Death Causes HMGB1 Release and Is Proinflammatory.
To further test the hypothesis that asbestos-induced HM cell death was a regulated form of necrosis, we evaluated HMGB1 localization by immunofluorescence staining. Six hours after asbestos exposure, HMGB1 translocated from the nucleus into the cytosol, as observed in HM exposed to H2O2. Conversely, in actinomycin D–exposed HM cells, HMGB1 remained within the nucleus (Fig. 3A). To verify the results, whole-cell extracts and concentrated culture medium were analyzed by Western blot. After 24 h of asbestos exposure, HMGB1 was released into the media in concert with cell death (Fig. 3 B and C). Release of HMGB1 into the extracellular space was paralleled by an increase in HMGB1 transcripts (Fig. S3).
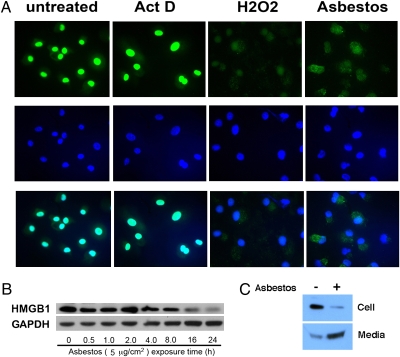
HMGB1 is released from HM into the extracellular space after asbestos exposure. (A) HMGB1 translocates from the nucleus to the cytosol upon asbestos exposure. Top row: HMGB1 staining (FITC conjugated antibody, green). Middle row: DAPI staining (blue). Bottom row: Overlay of HMGB1 (green) and DAPI staining (blue). HM were exposed to crocidolite asbestos (5 μg/cm2), H2O2 (100 μM) or actinomycin D (0.1 μM) for 6 h before immunofluoresence staining. Nuclei were visualized by DAPI staining. HMGB1 is localized in the nuclei in controls and actinomycin D–treated cells. In HM exposed to crocidolite or treated with H2O2, HMGB1 translocates to the cytosol and is seen mainly outside the nuclei (“overlay” panels, bottom row, where HMGB1 and DAPI stains are overlayed to identify location of HMGB1). (B) HMGB1 is released from HM after asbestos exposure. HM were exposed to 5 μg/cm2 asbestos for 0.5–24 h. Cell extracts were analyzed by Western blot. The amount of HMGB1 decreases 16 h after asbestos exposure when cell death becomes detectable. (C) HMGB1 is released from HM into the extracellular medium during asbestos exposure. HM were exposed to asbestos (5 μg/cm2) for 24 h. (Upper) cell extract. (Lower) Cell culture medium was collected, concentrated, and tested by Western blot with a HMGB1-specific antibody.
Next, we tested whether the release of HMGB1 was linked to an inflammatory response. The cell medium was collected, concentrated, and added to macrophages in tissue culture. Macrophage activation was measured by the secretion of the proinflammatory cytokine TNF-α, which promotes asbestos carcinogenesis (14). We found that the cell culture medium from asbestos-exposed HM promoted macrophage secretion of TNF-α. Similar results were obtained using the culture medium from HM in which necrosis was induced by H2O2. The culture medium from HM exposed to actinomycin D (positive control for apoptosis) did not induce TNF-α secretion (Fig. 4). To confirm that the induction of TNF-α secretion by macrophages was mediated through the HMGB1 released by necrotic HM, we incubated the culture medium of HM exposed to asbestos with Box A, a fragment of HMGB1 with antagonist activity (33, 34). Box A significantly reduced TNF-α secretion by macrophages treated with culture medium from asbestos-exposed HM (P < 0.05) (Fig. 4). These findings indicate that the release of HMGB1 from asbestos-exposed HM triggers the release of TNF-α and the inflammatory response associated with asbestos carcinogenesis.
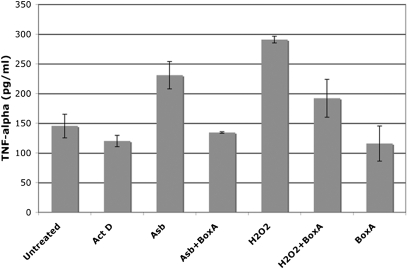
Asbestos-induced cell death and the release of HMGB1 promote TNF-α secretion by macrophages. HM were exposed to asbestos (5 μg/cm2), treated with H2O2 (1 mM) or actinomycin D (0.5 μM) for 1 h. Drugs were washed away and cells were fed with fresh media. After 24 h, cell culture media were collected, concentrated by ultrafiltration, and added to 105 macrophages in tissue culture with or without preincubation with Box A. The amount of TNF-α released by macrophages was measured 24 h later. TNF-α secretion is induced by culture medium from asbestos-exposed HM and from H2O2-treated HM but not by the medium from actinomycin D–treated HM. TNF-α secretion is significantly inhibited by preincubation of medium with HMGB1 antagonist Box A (100 ng/mL) (P < 0.05).
HMGB1 Is Linked to Pathogenesis of Asbestos-Related Disease.
We investigated the possible involvement of HMGB1 in the pathogenesis of asbestos-related disease in our mouse and hamster asbestos experimental models (35). Six hamsters and 10 BALB/c mice were injected with crocidolite asbestos intraperitoneally (i.p.). Chronic inflammation and mesothelial hyperplasia were detected in the peritoneum and tunica vaginalis of all of the injected hamsters and mice around areas of asbestos deposits. Histology showed that in these areas, the asbestos fibers were associated with cells with morphology consistent with macrophages, some of these cells multinucleated (foreign body–type histiocytic cells). Several of these cells contained asbestos fibers or part of these fibers in their cytoplasm (phagocytosis). We observed the same phagocytic response when HM or macrophages were grown in tissue culture in the presence of asbestos. Unlike other cell types, such as fibroblasts, we observed that both HM and macrophages phagocytized asbestos. Immunohistochemistry (performed on mouse tissues) showed that most of the mononuclear and the multinucleated cells found around asbestos deposits were positive for the macrophage marker, F4/80. In addition, there were scattered lymphocytes (CD4+ and CD8−), plasma cells and HM (pan-cytokeratin+) (Fig. S4). The inflammatory reaction formed irregular nodules around asbestos deposits. These nodules were situated in the loose connective tissue below the mesothelial covering (Fig. 5 and Fig. S4A). The mesothelial cells above these nodules showed focal hyperplasia. HMGB1 and TNF-α staining were specifically localized in these areas. Representative staining of mice specimens are shown in Fig. 5 and S4. HMGB1 was localized in both the nuclei and in the cytoplasm of reactive mesothelial and inflammatory cells and in the nearby extracellular space, consistent with HMGB1 release from cells undergoing necrosis. Away from areas of asbestos deposits, no chronic inflammation or HMGB1 cytoplasmic staining was detected (Fig. 5 A and B, Middle). These findings were very specific and consistently observed in all specimens from mice and hamsters (Fig. S5). TNF-α expression was detected in the same areas (Fig. 5 A and B, Right), consistent with our in vitro data showing that HMGB1 release leads to TNF-α secretion by inflammatory cells (Fig. 4).
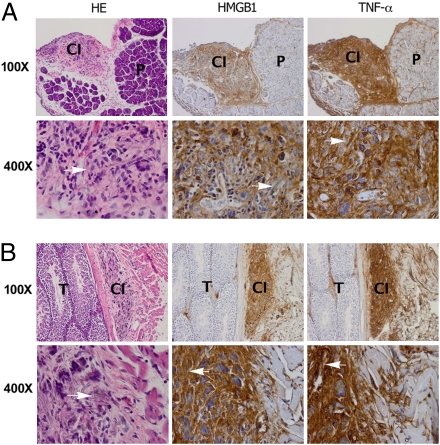
Immunohistochemical analyses show strong HMGB1 and TNF-α staining around areas of asbestos deposits in two representative murine specimens. (A) (Left) H&E staining shows nodular area of chronic inflammation (CI) around asbestos deposits under peritoneum, top left quadrant. P, pancreas. HMGB1 (Center) and TNF-α (Right) are present in area with chronic inflammation and not in pancreas (some staining is seen also along connective tissue that divides pancreatic lobes that contains lymphatic vessels). (Original magnification: top row, 100×; lower row, 400×.) (B) Top row. (Left) H&E staining. Testis (T) is shown on left half of figure. Tunical albuginea separate testis from tunical vaginalis (i.e., peritoneum), where chronic inflammation (CI) has formed around areas containing asbestos fibers. HMGB1 (Center) and TNF-α (Right) stain area with chronic inflammation (some staining can be seen also along the connective tissue, right portion of the panel, which contains lymphatic vessels with inflammatory cells). (Original magnification: top row, 100×; lower row, 400×.). H&E staining: Arrows point to asbestos fibers surrounded by inflammatory cells, macrophages, giant cells, and lymphocytes. HMGB1 and TNF-α are detected in cytoplasm and extracellular space.
Serological Analyses.
To begin the investigation into the relevance of these results to the pathogenesis of asbestos-related disease in humans, we checked the serum levels of HMGB1 in 20 individuals with a documented history of asbestos exposure, compared with 20 heavy smokers with lung inflammation and bronchoscopic evidence of dysplasia but without any history of asbestos exposure and also with 20 nonsmokers, non–asbestos-exposed healthy control individuals (information on assessment of exposure of these individuals can be found in SI Text, Table S1, and ref. 38). The serum level of HMGB1 (mean ± SE) in asbestos-exposed individuals was 80.2 ± 12.4 ng/mL, which was more than four times higher than the levels observed in nonexposed controls (16.9 ± 3.6 ng/mL, P < 0.0001) and approximately three times higher than the levels found in heavy smokers (26.1 ± 4.1 ng/mL, P < 0.001; Fig. 6). These results were separately reproduced in an additional 15 asbestos-exposed individuals compared with nonsmoker healthy controls (Fig. S6). These results suggest that the observations that we made in vitro and in vivo may be relevant to the pathogenesis of asbestos-related disease in humans, and also suggest that HMGB1 should be investigated as a possible biomarker to identify individuals/cohorts exposed to asbestos.
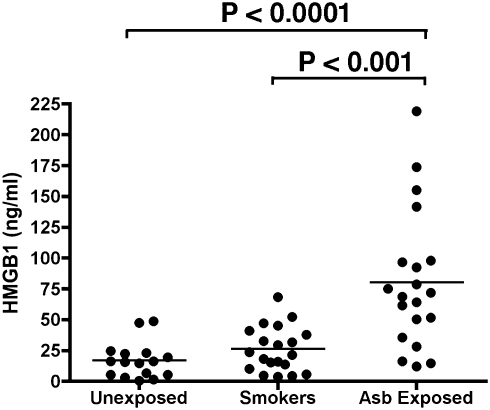
HMGB1 levels in serum from individuals exposed to asbestos, in heavy smokers, and in nonsmoker,non‐asbestos-exposed controls. Bars show mean of HMGB1 levels. Mean serum HMGB1 level in asbestos-exposed individuals was significantly higher than in unexposed controls (P < 0.0001) and also significantly higher than in heavy smokers with lung inflammation (P < 0.001). Twenty individuals were studied in each group. ELISAs shown were performed in parallel and blindly. Results were reproduced in an additional 15 asbestos-exposed individuals from the same cohorts (Fig. S6).
Discussion
Our results are consistent with the following scenario: asbestos causes mesothelial necrotic cell death and the release of HMGB1, thereby promoting an inflammatory response. Macrophages and mesothelial cells release ROS, such as H2O2 (Fig. S7), and secrete TNF-α (Fig. 4); both amplify the inflammatory process. Moreover, ROS cause DNA damage and aneuploidy (7, 8). TNF-α activates NF-κB, a survival pathway that allows some mesothelial cells that have undergone asbestos-induced DNA damage to survive rather than die, thereby creating a pool of aneuploid mesothelial cells with the potential to develop into cancer cells (14). The chronic release of HMGB1 around areas of asbestos deposits (Fig. 5 and Figs. S4 and S5) sustains the inflammatory process. At the same time, TNF-α and other cytokines released by the inflammatory cells may further promote the division of mutation-bearing HM, ultimately leading to the emergence of malignant cell clones.
Previous reports indicated that a fraction of HM exposed to asbestos undergo apoptosis. This fraction was estimated to vary between ~8–18% at concentrations of 5–10 μg/cm2, respectively, 24 h from exposure (16, 17). However, at these concentrations of asbestos, considerably more than 50% of HM are dead or are dying within 24 h (14, 15). Thus, what are the majority of HM exposed to asbestos dying of? Autophagy does not appear to play a role (Fig. S8). Instead, our results show that the vast majority of HM exposed to asbestos die of programmed necrosis. It is possible that the absence of clear markers of necrosis prevented previous studies to identify the extent of this phenomenon. In fact, PARP activation may have led to the interpretation that asbestos was causing apoptosis, because initially PARP activation was considered a marker of apoptosis. Only recently has necrosis been associated with the hyperactivation of PARP that leads to ATP depletion and HMGB1 release (21, 23), allowing us to identify cells undergoing necrosis. Our finding that asbestos promotes necrotic cell death is in agreement with the histopathological observation that asbestos causes chronic inflammatory reaction in both humans and rodents (1, 6, 36). In contrast, apoptotic cell death is not associated with the release of HMGB1 from cells and with the consequent inflammation (22, 27, 30). Thus, apoptosis may not be nearly as relevant to the pathogenesis of asbestos-related disease as necrosis. It is possible that apoptosis, by removing mesothelial cells damaged by asbestos without causing an inflammatory process, may have a protective role and decrease the risk of malignancy.
Our in vitro data were supported by our findings in hamsters and mice injected with asbestos. In these animals, histology showed areas of chronic inflammation with macrophage accumulation and mesothelial hyperplasia in and around areas of asbestos fiber deposition. These areas were scattered around the peritoneum and tunica vaginalis. HMGB1 and TNF-α staining were specifically detected in these areas. HMGB1 was detected in the cytoplasm and in the extracellular space only in areas of asbestos deposition, consistent with our in vitro data. This finding was strikingly reproducible in every animal and in every area of asbestos deposition. Moreover, we found that HMGB1 serum levels were approximately four times higher in individuals with a history of asbestos exposure compared with unexposed healthy controls (P < 0.0001) (Fig. 6). Furthermore, to rule out the possibility that the high levels of HMGB1 detected in the asbestos-exposed cohort were simply a result of inflammation and were not specifically linked to the pathogenic process caused by asbestos, we checked HMGB1 serum levels in a cohort of heavy smokers with brochoscopic evidence of dysplasia and we observed that the asbestos-exposed cohort had significantly higher level of HMGB1 than the smoker cohort (P < 0.001) (Fig. 6).
In summary, we report that crocidolite asbestos induces HM death primarily through programmed necrosis, involving PARP activation, H2O2 secretion, ATP depletion, and HMGB1 release. Our results provide a mechanistic rationale that links asbestos-induced mesothelial cell death to the chronic inflammatory reaction that is associated with asbestos carcinogenesis. By identifying necrosis and HMGB1 release as the triggers of the pathogenesis of asbestos-related disease, we provide potential targets for the prevention of MM and other asbestos-related cancers. Future studies should investigate whether the inhibition of the release of HMGB1 or the neutralization of its activity may be able to prevent or reduce asbestos carcinogenesis by decreasing chronic inflammation.
Materials and Methods
Cell Cultures.
Primary HM from three different donors were characterized as described (14, 15) and used between passages 4–7 in triplicate experiments. THP-1 human monocytes (ATCC) were differentiated into macrophages by phorbol 12-myristate 13-acetate (TPA), as described elsewhere (37).
Materials.
Actinomycin D was obtained from Calbiochem, z-VAD-fmk from R&D Systems, and H2O2, and TPA and 3-ABA from Sigma. Box A was provided by HMGBiotech. Crocidolite asbestos was obtained from the Union Internationale Contre le Cancer (UICC) and processed as previously described (14). Fiber concentration was 5 μg/cm2 or as indicated.
Western Blotting.
Western blot assays were performed as previously described (14) with anti-HMGB1 and anti-PAR (BD Bioscience Pharmingen), anti-PARP, anti-caspase-3 (Santa Cruz), and anti-GAPDH (Chemicon International). To assess HMGB1 release by HM, media were concentrated with Amicon Centrifugal Filter (Millipore).
Cytotoxicity.
HM cytotoxicity was analyzed 24 h after exposure to crocidolite, with or without 0.5 mM of the PARP inhibitor 3-ABA, by the LDH-cytotoxicity detection kit (Roche) according to the manufacturer's instructions.
PARP Activity.
PARP activity was determined in HM exposed to crocidolite, by using 32P-NAD (Amersham) and Poly (ADP-ribose) Polymerase Assay Kit (Trevigen). 32P incorporation in ribosylated proteins was evaluated with a scintillation counter.
Determination of ATP.
ATP levels were determined by the bioluminescence somatic cell assay kit according to the instructions of the manufacturer (Sigma) in HM treated with actinomycin D (0.1 μM), H2O2 (200 μM), or different doses of crocidolite (0.5, 1.0, 2.5, 5.0, or 10 μg/cm2) for 24 h.
Immunofluorescence.
HM were exposed to crocidolite, H2O2 (100 μM) or actinomycin D (0.1 μM) for 6 h. After fixation and permeabilization, cells were incubated with HMGB1 antibody (BD Bioscience Pharmingen) and a fluorescein-conjugated secondary antibody (Chemicon, Temecula). Nuclei were stained with DAPI.
TNF-α Secretion.
HM were exposed to crocidolite or treated with H2O2 (1 mM) or actinomycin D (0.5 μM) for 1 h. After 24 h, culture media were concentrated by ultrafiltration, and TNF-α production by macrophages was measured by the Quantikine human TNF-α ELISA kit (R&D Systems). HMGB1 was antagonized by adding Box A at 100 ng/mL.
Animal Experiments.
Animal experiments were performed as described (35). Briefly, six female Syrian hamsters and 10 BALB/c mice that were 21-d-old (to test for interspecies variability) were injected intraperitoneally with 0.4 mg crocidolite in PBS every 2 wk for 10 wk, for a total amount of 4 mg. Control groups (six hamsters and 10 mice) were injected with PBS. Animals were killed after 4 mo. All of the major organs were evaluated and studied histologically.
Immunohistochemistry.
Immunohistochemical analyses of hamster and mouse tissues were performed according to standard procedures (SI Materials and Methods).
ELISA for HMGB1.
The human HMGB1 ELISA kit (Shino-Test Corporation) was used to measure the level of serum HMGB1. Specimens were tested in duplicate.
Serum.
Serum samples were collected from 20 individuals with a documented history of asbestos exposure (38) and from unexposed controls (SI Materials and Methods). Participants provided informed consent, and procedures and protocols were approved by the institutional review board (IRB).
Statistical Analysis.
Statistical differences were evaluated by unpaired Student's t test and considered significant at P < 0.05.
Acknowledgments
We thank Francesco De Marchis for quality control of Box A. We thank Dr. Giovanni Gaudino for the critical review. This work was supported by National Cancer Institute (NCI) Grants R01 and P01 (to M.C.); the Mesothelioma Applied Research Foundation (MARF), the Hawaii Community Foundation’s Leahi fund, the Riviera United-4 a CURE (to H.Y.); Grant NCI R01 (to G.F.); the Associazione Italiana Ricerca sul Cancro (M.E.B.); and the Early Detection Research Network (EDRN)-NCI (H.I.P.).
Footnotes
Conflict of interest statement: M.E.B. is founder and part owner of HMGBiotech, a company that provides goods and services related to HMGB proteins.
This article contains supporting information online at www.pnas.org/lookup/suppl/10.1073/pnas.1006542107/-/DCSupplemental.
References
Articles from Proceedings of the National Academy of Sciences of the United States of America are provided here courtesy of National Academy of Sciences
Full text links
Read article at publisher's site: https://doi.org/10.1073/pnas.1006542107
Read article for free, from open access legal sources, via Unpaywall:
https://europepmc.org/articles/pmc2906549?pdf=render
Free after 6 months at www.pnas.org
http://www.pnas.org/cgi/content/abstract/107/28/12611
Free after 6 months at www.pnas.org
http://www.pnas.org/cgi/content/full/107/28/12611
Free after 6 months at www.pnas.org
http://www.pnas.org/cgi/reprint/107/28/12611.pdf
Citations & impact
Impact metrics
Article citations
Diagnosis of Pleural Mesothelioma: Is Everything Solved at the Present Time?
Curr Oncol, 31(9):4968-4983, 27 Aug 2024
Cited by: 0 articles | PMID: 39329996 | PMCID: PMC11430569
Review Free full text in Europe PMC
Adjuvant Novel Nanocarrier-Based Targeted Therapy for Lung Cancer.
Molecules, 29(5):1076, 29 Feb 2024
Cited by: 4 articles | PMID: 38474590 | PMCID: PMC10934468
Review Free full text in Europe PMC
Immune modulation in malignant pleural effusion: from microenvironment to therapeutic implications.
Cancer Cell Int, 24(1):105, 12 Mar 2024
Cited by: 0 articles | PMID: 38475858 | PMCID: PMC10936107
Review Free full text in Europe PMC
IGF2BP3 prevent HMGB1 mRNA decay in bladder cancer and development.
Cell Mol Biol Lett, 29(1):39, 19 Mar 2024
Cited by: 3 articles | PMID: 38504159 | PMCID: PMC10949762
Pleural Mesothelioma: Treatable Traits of a Heterogeneous Disease.
Cancers (Basel), 15(24):5731, 06 Dec 2023
Cited by: 0 articles | PMID: 38136277 | PMCID: PMC10741585
Review Free full text in Europe PMC
Go to all (165) article citations
Data
Data behind the article
This data has been text mined from the article, or deposited into data resources.
BioStudies: supplemental material and supporting data
Similar Articles
To arrive at the top five similar articles we use a word-weighted algorithm to compare words from the Title and Abstract of each citation.
Asbestos induces mesothelial cell transformation via HMGB1-driven autophagy.
Proc Natl Acad Sci U S A, 117(41):25543-25552, 30 Sep 2020
Cited by: 42 articles | PMID: 32999071 | PMCID: PMC7568322
HMGB1 released by mesothelial cells drives the development of asbestos-induced mesothelioma.
Proc Natl Acad Sci U S A, 120(39):e2307999120, 20 Sep 2023
Cited by: 0 articles | PMID: 37729199 | PMCID: PMC10523480
Crocidolite asbestos induces apoptosis of pleural mesothelial cells: role of reactive oxygen species and poly(ADP-ribosyl) polymerase.
Environ Health Perspect, 105 Suppl 5:1147-1152, 01 Sep 1997
Cited by: 11 articles | PMID: 9400715 | PMCID: PMC1470163
Inflammatory Alteration of Human T Cells Exposed Continuously to Asbestos.
Int J Mol Sci, 19(2):E504, 08 Feb 2018
Cited by: 6 articles | PMID: 29419731 | PMCID: PMC5855726
Review Free full text in Europe PMC