Abstract
Free full text

RIPPED TO DEATH
Abstract
An old puzzle in the field of cell death was recently solved: the mysterious embryonic lethality of animals deficient either in caspase-8 or FADD, proteins involved in a pathway of apoptosis. This lethality is caused by a failure to develop the yolk sac vasculature rather than a lack of apoptosis. Remarkably, development is rescued by ablation of either of two Receptor Interacting Protein Kinases (RIPKs). Despite being well-known cell killers, caspase-8 and FADD act together to block RIPK-mediated necrosis. To manifest this newly elucidated pro-survival function, FADD and caspase-8 depend on FLIPLong, a catalytically inactive caspase-8 homolog. In this review, the mechanism by which RIPK necrotic death is inhibited by this trio is discussed, as well as how RIPKs might themselves mediate cell death.
Apoptosis and Necrosis as active processes
Cell death, while unavoidable, is also a critical part of animal development and homeostasis. It is widely accepted that most physiological cell death occurs via the process of apoptosis, involving the engagement of molecular pathways that culminate in the activation of cysteine proteases, known as caspases. Caspases cleave roughly a thousand substrates in the cell, some of which function to “package” the corpse for efficient removal without invoking inflammation [1]. This contrasts with necrotic cell death, generally regarded as the result of irreparable damage under pathological conditions, and which, unlike apoptosis, triggers inflammation.
This view of “active” (apoptotic) versus “accidental” (necrotic) cell death was challenged by early studies showing that inhibition of caspases often fails to block cell death, per se, but instead converts it from apoptosis to necrosis [2,3]. A partial resolution came with the realization that a step in the apoptotic process, the disruption of the mitochondrial outer membrane (mitochondrial outer membrane permeabilization, MOMP), can occur independently of caspases and results in a mitochondrial catastrophe from which the cell often does not recover [4].
This explanation, however, fails to account for a related set of findings in cells where ligation of death receptors (a subset of the Tumor Necrosis Factor Receptor [TNFR] superfamily, including TNFR1, CD95, and the TRAIL receptors) triggers caspase activation and apoptosis. While in some cells treated with ligands for these receptors, the inhibition of caspases preserves cell survival [5,6], in others it does not; rather, they die by active necrosis [3,6,7].
Mouse knockout studies made this quandary more urgent. In general, knockouts of proteins involved in apoptosis produce phenotypes consistent with decreased cell death (Table 1). For example, targeted deletion of the primary executioner caspase, caspase-3, produced a phenotype involving accumulation of extra neurons [8]. Similarly, deletion of caspase-9, an initiator caspase, which cleaves and activates caspase-3 in the mitochondrial pathway of apoptosis, produced a similar phenotype [9,10]. Deletion of caspase-8, the initiator caspase of the death receptor pathway of apoptosis, however, produced an unexpected phenotype: all embryos died around embryonic day 10.5 due to a failure to properly develop yolk sac vasculature. This lethal effect could not be attributed to a lack of apoptosis [11,12], suggesting instead a survival role for caspase-8. To resolve this puzzle, we will revisit the mechanism of caspase-8 lethality and unveil how one of its proteolytically inactive homologs, FLIPLong (hereafter referred to as FLIP), transforms caspase-8 into a protective form that inhibits a type of necrotic death regulated by RIPK kinases.
Table 1
Phenotypes of knockout mice in apoptotic and necrotic pathways.
Gene | Age Lethal | Phenotype | References |
---|---|---|---|
Caspase-3 | E18.5 to perinatal | Abnormal growth of the forebrain, midbrain, and hindbrain; exencephaly | [8] |
Caspase-9 | E16.5 to perinatal | Forebrain and hindbrain exencephaly | [9,10] |
APAF-1 | E16.5 to perinatal | Forebrain and hindbrain exencephaly | [89,90] |
Caspase-8 | E10.5-12.5 | Defective myocardial development and accumulation of erythrocytes | [20, 54] |
FLIP | E10.5-12.5 | Defective myocardial development, blood pooling in head and abdominal regions | [56] |
FADD | E10.5-12.5 | Defective myocardial development and abdominal hemorrhage | [35,91] |
TRADD | Viable | No gross phenotype | [17,21,22] |
RIPK1 | P3-5 | Edema, failure to gain weight, immune and adipose defects | [92] |
RIPK3 | Viable | No gross phenotype | [93] |
CD95 | Viable | Lymphoproliferation and liver hyperplasia in adults | [94,95] |
CD95L | Viable | Lymphoproliferation | [96] |
TNFR1 | Viable | No gross phenotype | [97] |
How caspase-8 kills cells
Unlike executioner caspases (such as caspase-3), which are expressed as inactive dimers and are activated by cleavage [13], initiator caspases (such as caspase-8) are expressed as inactive monomers and are activated by proximity-induced dimerization [14,15]. Caspase-8 dimerization triggers catalytic activity and autocleavage, which stabilizes the active dimer [16]. The adapter molecule that binds and activates caspase-8 is FADD. Once activated, caspase-8 triggers apoptosis by cleaving and thus activating caspase-3 and caspase-7 (another executioner caspase), or by cleaving the BCL-2 family protein, BID, causing MOMP which further facilitates the apoptotic process in many cells (Figure 1).
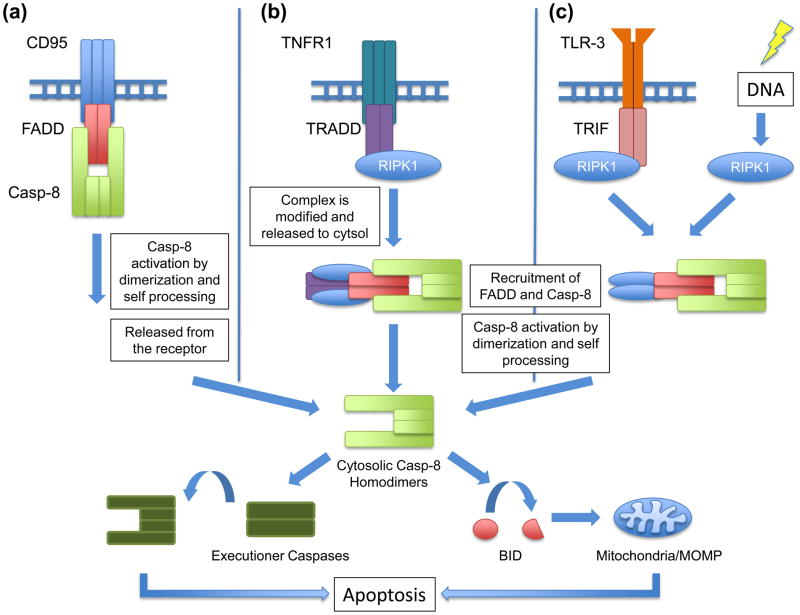
(a) Engagement of a death receptor such as CD95 by its ligand recruits FADD, which in turn recruits caspase-8. The close proximity of the inactive caspase-8 monomers forces their dimerization, triggering catalytic activity and autocleavage, which further stabilizes caspase-8 in its active form. Upon release into the cytosol, caspase-8 can either cleave and activate effector caspases or cleave BID, which induces mitochondrial outer membrane permeabilization (MOMP). (b) The activation of caspase-8 can also be achieved through ligation of TNFR1 by TNF, which recruits TRADD and RIPK1. Before being able to recruit FADD, and subsequently caspase-8, this complex is modified by several ubiquitination and deubiquitination events, resulting in its release from the TNF receptor. (c) Toll-Like Receptors (TLRs) which signal through TRIF, namely TLR3 and TLR4, can also engage caspase-8. This occurs through a complex that contains TRIF and depends on RIPK1 and FADD. Additionally, genotoxic stress can activate caspase-8 via RIPK1-FADD complexes.
Upon ligation, some death receptors, such as CD95, recruit FADD, which in turn, binds and activates caspase-8 (Figure 1a). The engagement of the death receptor TNFR1 leads to the recruitment of a different adapter molecule, TRADD, which does not directly bind and activate caspase-8 (Figure 1b) [17,18]. Instead, a number of additional signaling molecules associate with both the receptor and TRADD (e.g. Receptor-Interacting Serine-Threonine Kinase-1 [RIPK1]), and after several modifications (involving ubiquitination and deubiquitination events) a complex that includes TRADD is released to the cytosol, where it binds FADD and activates caspase-8 [19].
Germ line deletion of FADD is lethal around embryonic day 10.5, manifesting the same phenotypic effects seen in caspase-8 knockouts [20] (Table 1). In contrast, deletion of TRADD is not developmentally lethal even though TRADD is required for TNF-induced caspase-8 activation and apoptosis [17,21,22].
In response to DNA damage [23,24] or ligation of Toll-Like Receptor-3 (TLR-3) [25,26], caspase-8 is activated through another type of FADD-containing complex (Figure 1c). In these complexes, formed by self-assembly or by TLR-3/TRIF platforms, RIPK1 is activated to recruit FADD, which then binds and activates caspase-8 (see Box 1). While this process requires the kinase activity of RIPK1, it is not yet clear how phosphorylation of one or more of its substrates is involved. As FADD is known to be phosphorylated in some settings [27,28], it is possible that phosphorylation of FADD by RIPK1 may play a role in assembly of this complex; however, at present, this is merely speculation.
Caspase-8 activation through FADD binding has been described in other settings, including the ectopic expression of proteins with extended poly-glutamine tracks (a model for Huntington’s disease) [29] and the persistence of the ATG5-12 complex in autophagy [30]. How these modalities relate to the story we develop here remains obscure, however.
The FLIP side: How caspase-8 prevents death
As previously discussed, inhibition of caspases sensitizes some cells to necrotic death induced by ligation of death receptors (see above). A similar phenomenon occurs in T lymphocytes whereby inhibition of caspases induces necrosis [31,32] and thus prevents the proliferation of naïve lymphocytes [33,34]. These effects are also seen when caspase-8 or FADD are specifically deleted, knocked down, or inhibited in cell lines or primary T cells [20, 28,35–40].
Survival of such cells depends on the catalytic activity of caspase-8, and therefore caspase-8 must be activated to have this protective effect (also supported by the role of FADD). But now we have a paradox—how can activated caspase-8 prevent necrosis without inducing apoptosis?
The answer revolves around the actions of FLIP, which while similar to caspase-8, lacks the catalytic site. Upon recruitment to FADD, FLIP effectively blocks caspase-8-mediated apoptosis [41]. For this reason, FLIP was thought of as a “dominant negative” caspase-8. This view was challenged, however, with the realization that caspase-8-FLIP heterodimers are proteolytically active [42,43]; FLIP promotes folding of caspase-8 to form the catalytic site on the latter [43]. This heterodimer assembles with higher affinity and/or stability than that of the caspase-8 homodimer, and appears to be the preferred form when FLIP is present [42]. Of note, the alternatively spliced FLIP isoform, FLIPshort (FLIPS), does not create proteolytically active heterodimers with caspase-8; rather, it blocks caspase-8 processing into its active form (44).
Upon ligation of TNFR, signaling events dependent on TRADD (but not FADD) induce the activation of NF-κB [17,45]. One transcriptional target of NF-κB is FLIP, whose expression is essential for blocking TNF-induced, caspase-8-mediated apoptosis [46]. If NF-κB is blocked or the pathway is disrupted, apoptosis ensues [47,48]. Animals lacking components of the NF-κB pathway die around embryonic day 15 due to hepatic injury [49], and the development of such animals is rescued by deletion of TNFR1 [50]. While this demonstrates that TNF-induced NF-κB is required to protect mouse development, it also suggests a different mechanism of lethality than that caused by knockout of caspase-8 or FADD.
Five lines of evidence point to the idea that caspase-8-FLIP is the catalytically active moiety that protects wild type mice from lethality at embryonic day 10.5 and cells from necrotic death without triggering apoptosis. The first involves the viral serpin, CrmA. While CrmA potently blocks the enzymatic activity of caspase-8 homodimers, it is strikingly less effective in blocking the activity of caspase-8-FLIP heterodimers [51] (Figure 2a). Expression of CrmA in T cells [52] or fibroblasts [51] can block caspase-8-mediated apoptosis without sensitizing cells for necrosis induced by T- cell activation or TNF. This suggests that the caspase-8-FLIP heterodimer remains active to prevent necrosis while the activity of the apoptotic caspase-8 homodimer is blocked. It is unlikely that CrmA somehow also directly blocks necrosis, as higher expression of CrmA can ultimately sensitize cells to necrotic death, probably by blocking both the caspase-8 homodimers and caspase-8-FLIP heterodimers [3].

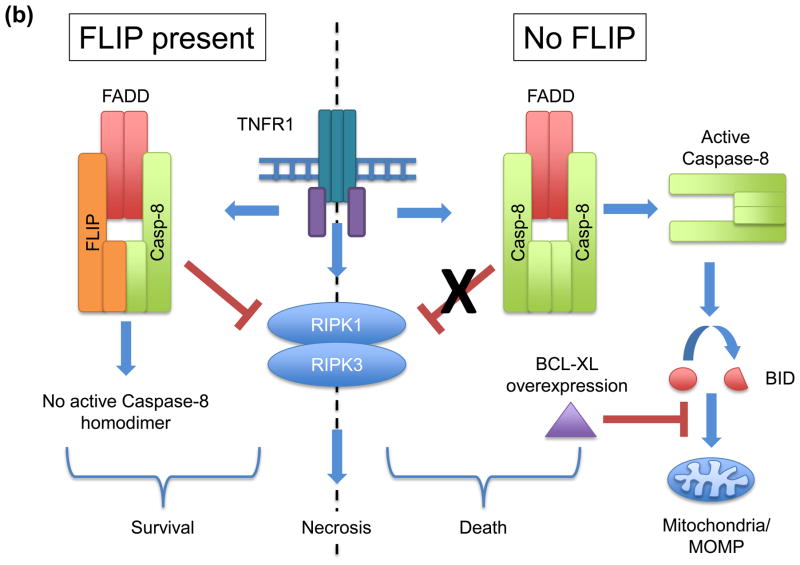
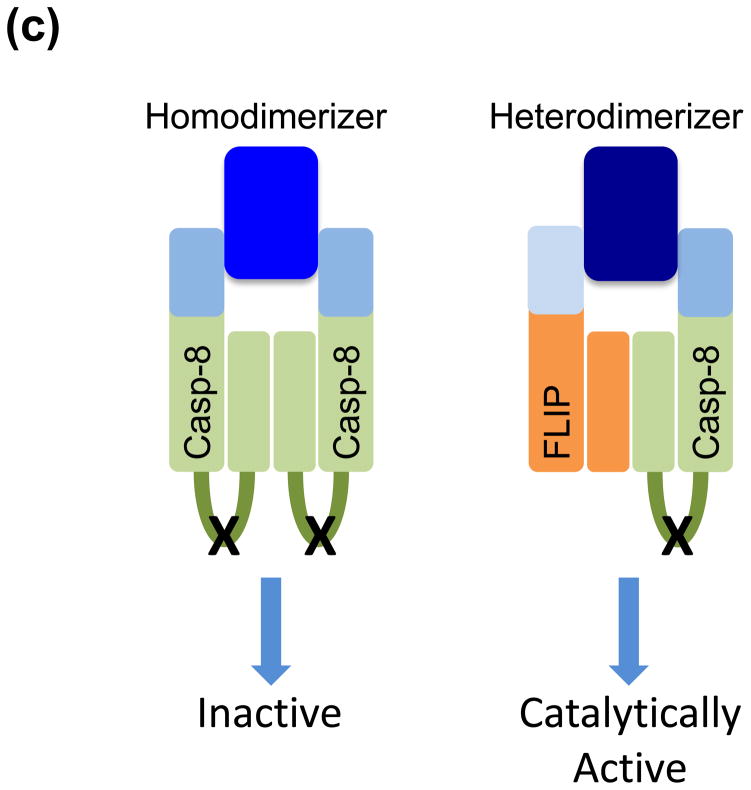
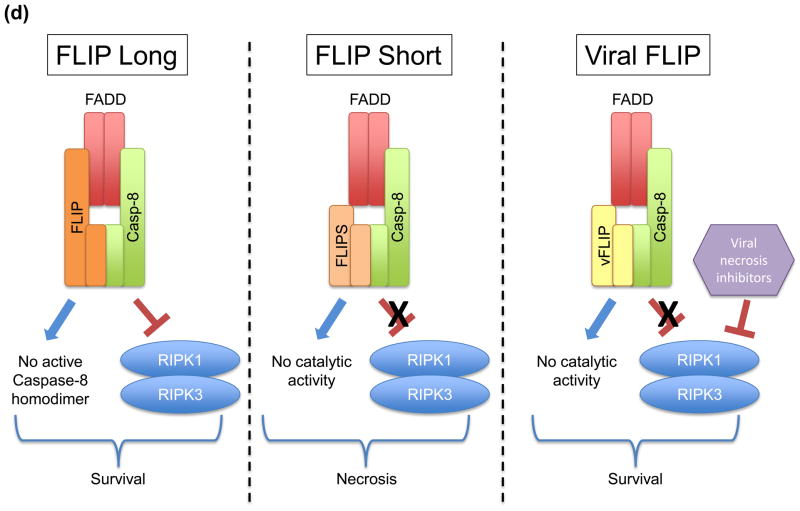
(a) Expression of the viral serpin, CrmA, blocks the apoptosis-inducing activity of caspase-8 homodimers, while not inhibiting the caspase-8-FLIP heterodimer from suppressing necrosis. (b) Cells lacking FLIP fail to suppress RIPK-necrosis in response to TNF, despite being able to activate caspase-8 homodimers in the presence of overexpressed BCL-XL, which blocks apoptosis. In the presence of FLIP, necrosis is suppressed and caspase-8 homodimer is not effectively formed, leading to survival of cells. (c) Enforced dimerization of noncleavable caspase-8 does not yield a catalytically active homodimer. However, heterodimerization of noncleavable caspase-8 and FLIP does produce catalytically active molecules. While transgenic expression of noncleavable caspase-8 fails to rescue sensitivity to death receptor mediated apoptosis, it nevertheless preserves development in mice. (d) Differential roles of FLIP isoforms in caspase-8 function. FLIPLong inhibits the apoptotic caspase-8 homodimers and forms a proteolytically active heterodimer, which is capable of blocking RIPK-mediated necrosis, promoting cell survival. In contrast, FLIPshort and viral FLIP also block caspase-8 homodimers, but heterodimers between these molecules and caspase-8 are not catalytically active and therefore incapable of blocking RIPK-necrosis. Some viruses encode inhibitors of caspase-8 and RIPKs, thereby promoting cell survival.
Another line of evidence comes from the examination of the effects of activating caspase-8 in the absence of FLIP in cells that are protected from apoptosis downstream of caspase-8 activation. In many cells, caspase-8 must trigger MOMP for apoptosis to occur (mentioned above), and this can be blocked by anti-apoptotic BCL-2 proteins, such as BCL-XL [53]. When BCL-XL is overexpressed, knockdown of FLIP sensitizes cells to caspase-8 activation (without apoptosis) but does not prevent TNF-triggered necrosis [51] (Figure 2b). Thus, while caspase-8-FLIP heterodimers protect cells from both apoptosis and necrosis, caspase-8 homodimers do not effectively block necrosis.
Providing the third line of evidence are experiments showing that transgenic expression of caspase-8 that lacks the stabilizing cleavage site rescues the development of caspase-8-deficient mice, but not their apoptotic response to death receptor ligation [54]. Biochemical studies showed that enforced dimerization of non-cleavable caspase-8 does not engage caspase activity in vitro, and does not trigger apoptosis in cells [15] (Figure 2c). However, hetero-dimerization of non-cleavable caspase-8 with FLIP results in full catalytic activity [55], providing further support that the caspase-8-FLIP heterodimer functions to preserve embryonic development.
The fourth line of evidence is circumstantial. Deletion of FLIP causes the same embryonic day 10.5 lethality seen in caspase-8 and FADD-deficient mice [56]. Thus, FADD, caspase-8, and FLIP all function to preserve development at the same stage.
The fifth line of evidence involves some speculation. It derives from the fact that while the long isoform of FLIP protects cells from CD95-induced necrosis in the absence of cIAPs, FLIPS, which does not confer proteolytic activity to caspases-8, does not [57] (Figure 2d). The increased sensitivity to death ligands in FLIPS-overexpressing cells correlates with an inability to inactivate RIPK1 molecules recruited to the DISC. T-cell specific transgenic expression of viral FLIP, which shares homology with FLIPS and blocks caspase-8 activity [58,59], prevents both thymocyte and T cell apoptosis; however, the survival of activated T cells and the generation of T cell memory is severely impaired [60], an effect that is probably due to induction of necrosis [31,32]. It is therefore likely that FLIP isoforms that do not form proteolytically active dimers with caspase-8 do not provide protection against necrosis.
While together these lines of evidence provide insight into how caspase-8, in the form of the caspase-8-FLIP heterodimer engaged by FADD, blocks necrosis, it does not tell us how. To probe this, we must examine this form of regulated necrosis, and the mechanisms whereby it is engaged.
RIPK-necrosis: Ripping the cell apart
RIPK1 does not only promote death via activation of caspase-8 (see above), it is also involved in promoting necrosis. An early study of TNF-induced necrosis (under conditions of caspase inhibition) presciently identified RIPK1 as required for this death pathway [7]. An inhibitor of TNF-induced necrosis, necrostatin-1 (nec-1), was later identified and found to inhibit the kinase activity of RIPK1 [61,62].
Another related enzyme, RIPK3, was subsequently identified as required for necrosis induced by TNF [6,63] or by viral infection [64,65]. RIPK1 and RIPK3 directly interact via RHIM domains in both proteins [6] and this interaction, as well as kinase activity of each, is required for active necrosis [64] (Figure 3a). In general, it is the presence or absence of RIPK3 that determines if a cell dies upon exposure to death receptor ligation in the absence of caspase activity; in the absence of RIPK3, caspase inhibition promotes survival [6].
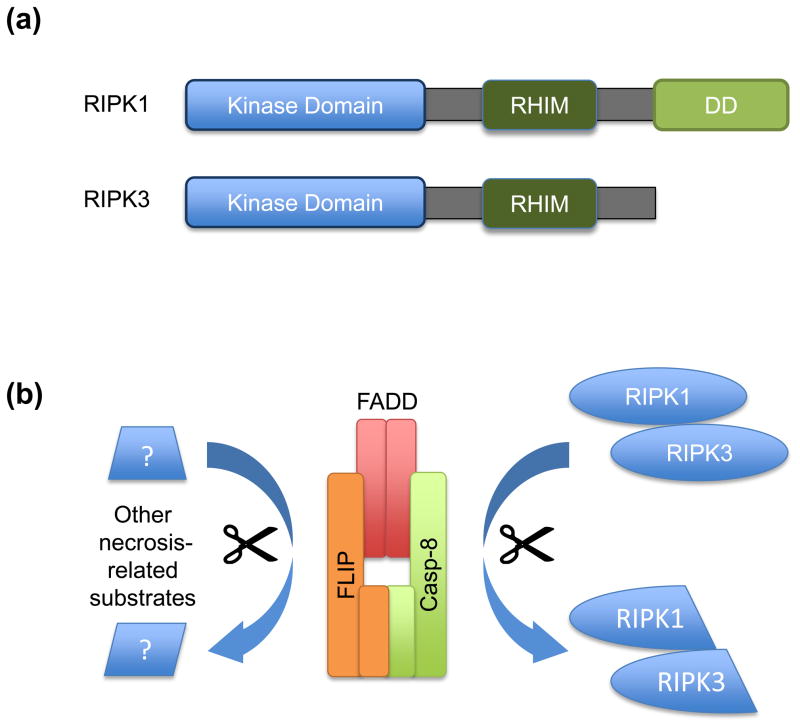
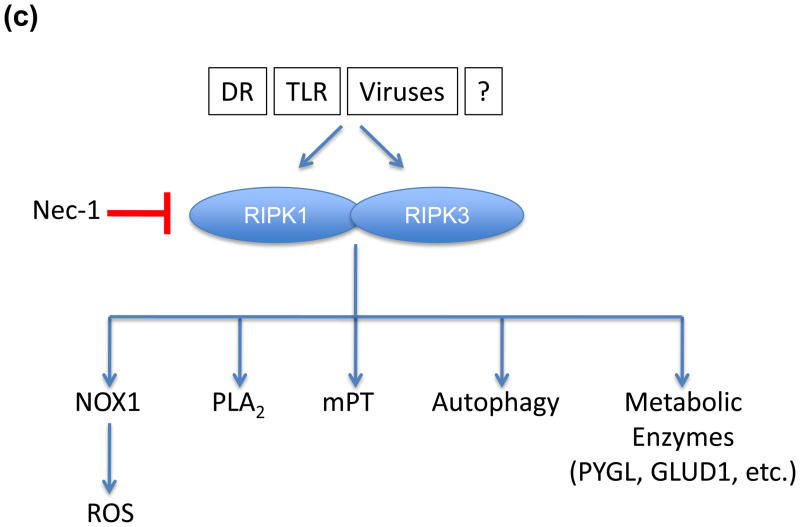
(a) Schematic of domain structures of RIPK1 and RIPK3. Both proteins have RHIM domains by which they interact with each other and to certain adapter molecules, such as TRIF. They also contain kinase domains upon which their function in necrosis is dependent. Additionally, RIPK1 possesses a DD domain, allowing it to interact with other death fold containing proteins such as TRADD and FADD. (b) The caspase-8-FLIP complex may inhibit RIPK1/RIPK3 activity through cleavage. Interestingly, caspase-8-FLIP is not released from the receptor proximal complex suggesting that it may have better access to the RIPKs as cleavage targets. However, other hitherto unidentified cleavage substrates may also play an important role in regulating necrosis. (c) The mechanism by which RIPK1 and RIPK3 mediate necrosis is poorly defined, but may include some or all of the illustrated targets.
Is RIPK-dependent necrosis responsible for the developmental effects of caspase-8 or FADD deletion? Answers to this question came with the findings that development in caspase-8-deficient mice and proliferation in caspase-8-deficient T cells is fully rescued by deletion of RIPK3 [51,66]. Further, embryonic lethality in FADD-deficient mice was rescued by deletion of RIPK1 [67], although these animals died perinatally (as do RIPK1 knockout mice). Together, these results say that the lethality of animals lacking caspase-8 or FADD depends on the presence of RIPK3 and RIPK1, and suggest (but do not formally prove) that this is an effect of blocking RIPK-dependent necrosis during development.
The mechanism by which FADD-caspase-8-FLIP prevents RIPK necrosis remains unclear. FADD acts as a scaffold to bring together caspase-8, FLIP, RIPK1 and RIPK3 in the same complex; without FADD, caspase-8 and FLIP are not recruited and RIPKs initiate necrosis upon their activation [68]. Both RIPK1 [69, 70] and RIPK3 [71] are known to be cleaved by caspase-8, and are, therefore, likely to be cleaved by the caspase-8-FLIP heterodimer (Figure 3b). Unlike mature caspase-8, caspase-8-FLIP is not released from the FADD-scaffolding complex and thus may have sustained access to key substrates that regulate necrosis, which likely include other proteins beyond the RIPKs [43]. Alternatively, the activity of caspase-8-FLIP engages active degradation of the entire complex, thereby inhibiting necrosis without inducing apoptosis. Cleavage of FLIP induces its degradation [72] and therefore this is another reasonable scenario, although currently untested.
As necrosis induced by viral infection requires RIPK3 but not RIPK1 [65], RIPK3 may act as the “downstream” trigger for this death process. But how does RIPK3 promote necrotic death? While several mechanisms have been proposed, none has been compellingly supported by inhibitor or knockdown studies (Figure 3c). For example, TNF can induce the activation of NADPH-oxidase-1 (NOX1) to produce reactive oxygen in some cells, which is further enhanced by caspase inhibition [73,74]. While RIPK-dependent necrosis induced by TNF is somewhat reduced by scavenging of reactive oxygen species [6,73,74], it is only marginally inhibited by blockade of NOX1 [74]. Similarly, while phospholipases D and A2 have been implicated in some TNF-induced cell death [75,76], inhibition of PLA2 does not dramatically rescue RIPK-dependent necrosis [77]. Other candidates, including autophagy and the mitochondrial permeability transition were examined in caspase-8-deficient T cells, and neither was implicated in the activation-induced, RIPK-dependent necrosis [32].
Another intriguing possibility is that RIPK3 phosphorylates several metabolic enzymes to increase their activities, altering metabolism to cause necrosis [63]. But again, knockdown of those enzymes, namely PYGL, GLUL and GLUD1, did not dramatically affect RIPK-dependent necrosis [63]. While it is possible that several (or all?) of these mechanisms act redundantly to mediate cell death, it is also possible that another, elusive mechanism remains to be identified.
Roles of RIPK-mediated necrosis
Several interesting questions remain. Associated with the developmental role of RIPK-dependent death, one important problem is to define which cells are actively shielded from necrosis prior to embryonic day 10.5 and why these cells acquired these protective mechanisms. One possible candidate is the stem cell precursor that originates in the embryonic aorta and participates in the development of the yolk sac, as the specific deletion of caspase-8 induced by the angiopoietin receptor Tie1 promoter recapitulates the lethality of the full caspase-8 knockout [78]. However, it is still not known if specific deletion of RIPK3 in these cells rescues embryonic development or, at least, prolongs survival, as it is still to be determined if other cell types become susceptible to RIPK-dependent necrosis. Further work is needed to determine when and where the triggers of RIPK-mediated death and the components of this pathway appear during development.
Little is currently known about RIPK3 gene expression. Further investigation of the RIPK3 promoter and the transcription factors involved in its modulation would help to define the conditions under which cells become vulnerable to RIPK-necrosis. For example, investigations of the expression of RIPK3 in virally infected cells and its implications for the progression of infections would provide valuable insight into the functionality of RIPK3-mediated necrosis.
RIPK-mediated necrosis is a fundamental cellular defense against viral infections, as viruses bearing apoptosis inhibitors can replicate for a longer period inside infected RIPK3-deficient cells [64,65] (see below); however, this might only partially explain the role of RIPKs in immunity. A complementary hypothesis is that virus-infected RIPK-necrotic cells are more immunogenic than virus-infected apoptotic cells. It is possible that phagocytosis of RIPK-necrotic cells results in enhanced inflammatory responses and better antigen presentation. In this regard, some questions still need to be addressed, such as whether the clearance of these cells is performed by the same cell populations or if they are preferentially phagocytosed by dendritic cells responsible for cross-presentation of viral antigens to T cells. Also, do dendritic cells use the same set of “eat-me” molecules, such as Tim-4, MGF-E8 and BAI-1, to clear RIPK-necrotic cells? Examination of the clearance rate and mechanisms could help elucidate the immunological benefits of each of these cell death mechanisms against viral infection. This may also have implications for tumor immunology if the immune response to cancer cells can be promoted by changing the mode by which these cells die.
Findings from recent studies on ubiquitination and deubiquitination of the TNF receptor signaling complexes suggest that these events play an important role in defining the final outcome of death receptor ligation, including RIPK-mediated death [79,80,81]. Cellular-IAPs are E3 ubiquitin ligases that function as negative regulators of TNF-induced cell death [57]. LUBAC, a complex of several proteins, mediates linear ubiquitination of complex I and induces its stabilization [79]. This avoids complex II formation, thus blocking apoptosis [80,81] and RIPK1-necrosis [81]. Several deubiquitinases, including CYLD, A20, and Cezanne, modify the balance between complex I and complex II [82,83], but of these, only CYLD has been directly implicated in the regulation of TNF-induced necrosis [84]. Therefore, a thorough study of how the different ubiquitin chains (or the lack of them) regulate the recruitment of TNF signaling players, especially RIPK1 and RIPK3, should shed light on the circumstances under which TNF stimulation results in necrosis.
Perhaps a more urgent question is this: Why is this system arranged in this way? Or more rigorously: Why did evolution favor a necrotic cell death mechanism that must be held in check by caspase-8-FLIP, without which the lethal effects of RIPK1-RIPK3 manifest?
Clues to this mystery come from findings that some viruses express inhibitors of caspase-8 [58,85]. Suppression of caspase-8 by viral inhibitors could de-repress RIPK3-dependent necrosis, which would function as a backup death-inducing mechanism, helping to reduce virus persistence and propagation. Indeed, RIPK3-deficient mice are very susceptible to vaccinia virus [64], which naturally expresses caspase inhibitors [86]. Cytomegalovirus, which also expresses caspase-8 inhibitors, similarly expresses M45/vIRA, an inhibitor of RIPK3 (Figure 2d); M45-deficient virus induces a much milder infection in wild type mice, as compared to RIPK3-deficient mice, where virulence fully manifests [65]. Moreover, the recent finding that caspase-8 and RIPKs are recruited together to pathogen-associated pattern receptors (PRRs), specifically TLR3 and TLR4 [25,26], suggests that apoptosis and RIPK-dependent necrosis may act together to control an even broader set of pathogens.
Death receptor-induced apoptosis and RIPK3-dependent necrosis are also redundant in the control of T-cell accumulation and autoimmunity. CD95 or CD95L deficiency induces the accumulation of an aberrant population of T cells that express B220, which coincides with autoimmunity [87]. The same phenomenon is observed only when both caspase-8 and RIPK3 are deleted [32,51,66]; in T cells, the suppression or elimination of FADD or caspase-8 [36,39] or the deletion of RIPK3 [32] by themselves does not result in lymphoaccumulation.
These two lines of evidence, however, do not shed light on why RIPK-dependent necrosis is active and must be restrained by caspase-8-FLIP activation during embryogenesis. The answer to this question, currently unknown, will likely take us still deeper into the physiological roles for RIPK-dependent necrosis, and when “ripping to death” is beneficial to the animal.
Concluding remarks
Taken together, the recent findings regarding this newly defined type of cell death, RIPK-mediated necrosis, and the involvement of a known cell killer, caspase-8, as its suppressor opens an exciting field of research, will likely have major impacts on our understanding of developmental processes, the immune responses to viruses as well as tumor immunity, and may well give rise to new targets for clinical intervention.
Footnotes
Publisher's Disclaimer: This is a PDF file of an unedited manuscript that has been accepted for publication. As a service to our customers we are providing this early version of the manuscript. The manuscript will undergo copyediting, typesetting, and review of the resulting proof before it is published in its final citable form. Please note that during the production process errors may be discovered which could affect the content, and all legal disclaimers that apply to the journal pertain.
References
Full text links
Read article at publisher's site: https://doi.org/10.1016/j.tcb.2011.09.002
Read article for free, from open access legal sources, via Unpaywall:
https://europepmc.org/articles/pmc3205316?pdf=render
Citations & impact
Impact metrics
Citations of article over time
Alternative metrics
Smart citations by scite.ai
Explore citation contexts and check if this article has been
supported or disputed.
https://scite.ai/reports/10.1016/j.tcb.2011.09.002
Article citations
Apoptosis, autophagic cell death, and necroptosis: different types of programmed cell death in bovine corpus luteum regression.
J Reprod Dev, 68(6):355-360, 17 Nov 2022
Cited by: 6 articles | PMID: 36384912 | PMCID: PMC9792655
Review Free full text in Europe PMC
The independence of and associations among apoptosis, autophagy, and necrosis.
Signal Transduct Target Ther, 3:18, 01 Jul 2018
Cited by: 134 articles | PMID: 29967689 | PMCID: PMC6026494
Increased Ripk1-mediated bone marrow necroptosis leads to myelodysplasia and bone marrow failure in mice.
Blood, 133(2):107-120, 09 Nov 2018
Cited by: 22 articles | PMID: 30413413 | PMCID: PMC6328629
T Cells and Regulated Cell Death: Kill or Be Killed.
Int Rev Cell Mol Biol, 342:27-71, 29 Aug 2018
Cited by: 15 articles | PMID: 30635093 | PMCID: PMC7325157
Review Free full text in Europe PMC
Origin and Consequences of Necroinflammation.
Physiol Rev, 98(2):727-780, 01 Apr 2018
Cited by: 96 articles | PMID: 29465288
Review
Go to all (45) article citations
Other citations
Wikipedia
Similar Articles
To arrive at the top five similar articles we use a word-weighted algorithm to compare words from the Title and Abstract of each citation.
RIPK-dependent necrosis and its regulation by caspases: a mystery in five acts.
Mol Cell, 44(1):9-16, 01 Oct 2011
Cited by: 108 articles | PMID: 21981915 | PMCID: PMC3192321
Review Free full text in Europe PMC
Survival function of the FADD-CASPASE-8-cFLIP(L) complex.
Cell Rep, 1(5):401-407, 01 May 2012
Cited by: 227 articles | PMID: 22675671 | PMCID: PMC3366463
Programmed cell death: Apoptosis meets necrosis.
Nature, 471(7338):310-312, 01 Mar 2011
Cited by: 102 articles | PMID: 21412328
Catalytically active Yersinia outer protein P induces cleavage of RIP and caspase-8 at the level of the DISC independently of death receptors in dendritic cells.
Apoptosis, 12(10):1813-1825, 01 Oct 2007
Cited by: 24 articles | PMID: 17624595
Funding
Funders who supported this work.
NIAID NIH HHS (4)
Grant ID: R01 AI047891-14
Grant ID: R01 AI044828-13
Grant ID: R01 AI047891
Grant ID: R01 AI044828