Abstract
Free full text

Inhibition of JAKs in Macrophages Increases Lipopolysaccharide-Induced Cytokine Production by Blocking IL-10–Mediated Feedback
Abstract
Macrophages are an important source of cytokines following infection. Stimulation of macrophages with TLR agonists results in the secretion of TNF-α, IL-6, and IL-12, and the production of these cytokines is controlled by multiple feedback pathways. Macrophages also produce IL-10, which acts to inhibit proinflammatory cytokine production by macrophages via a JAK/STAT3-dependent pathway. We show in this paper that, Ruxolitinib, a recently described selective inhibitor of JAKs, increases TNF, IL-6, and IL-12 secretion in mouse bone marrow-derived macrophages stimulated with LPS. This effect is largely due to its ability to block IL-10–mediated feedback inhibition on cytokine transcription in macrophages. Similar results were also obtained with a second structurally unrelated Jak inhibitor, Tofacitinib. In addition, LPS induced the production of IFN-β, which was then able to activate JAKs in macrophages, resulting in the stimulation of STAT1 phosphorylation. The initial induction of IL-10 was independent of JAK signaling; however, inhibition of JAKs did reduce IL-10 secretion at later time points. This reflected a requirement for the IFN-β feedback loop to sustain IL-10 transcription following LPS stimulation. In addition to IL-10, IFN-β also helped sustain IL-6 and IL-12 transcription. Overall, these results suggest that inhibition of JAKs may increase the inflammatory potential of macrophages stimulated with TLR4 agonists.
Introduction
Cytokines are critical regulators of both the adaptive and innate immune systems. The JAK/STAT signaling pathway is important in mediating many of the responses to cytokines and can be activated by multiple receptors from the IFN, γC, gp130, and single-chain families of cytokine receptors (1, 2). In total, four JAKs (JAK1, 2, 3, and Tyk2) and seven STATs (STAT1, 2, 3, 4, 5A, 5B, and 6) are encoded in the human genome, and different groups of cytokine receptors signal via specific combinations of JAK and STAT isoforms. JAK/STAT signaling is involved in many processes and has been implicated in several diseases including cancer and autoimmunity. For instance, activating mutations in JAK2 are a major cause of myeloproliferative neoplasms, whereas elevated STAT3 phosphorylation has been observed in many cancers (reviewed in Refs. 3–5). In addition, the important roles that the JAK/STAT pathway plays in the immune system have suggested JAKs as targets for the treatment of autoimmunity (6). As a result, there has been considerable interest in the identification of selective JAK inhibitors as therapeutic agents. This has led to the development of several highly selective JAK inhibitors including Tofacitinib (CP-690550) and Ruxolitinib (INCB018424) (7, 8).
Initial interest in JAK inhibitors for the treatment of autoimmunity focused on JAK3, because it is restricted to hematopoietic cells, and therefore, its inhibition may be less likely to result in adverse side effects. An important role for JAK3 in the human immune system has also been demonstrated by the finding that mutation of JAK3 in humans results in a SCID phenotype (9). Tofacitinib is a JAK inhibitor developed by Pfizer that was originally reported to show selectivity for JAK3 over JAK1 and JAK2 (7), although more recent reports have suggested that it can inhibit all three JAK isoforms (10, 11). Tofacitinib has shown considerable promise in clinical trials in autoimmune disorders (12). Ruxolitinib is a second-generation JAK inhibitor that was developed for the treatment of myeloproliferative neoplasms and which is now being evaluated for the treatment of autoimmunity (13). Ruxolitinib has been described to be highly selective for JAKs, with greatest potency against JAK1 and JAK2 (8). In addition to their therapeutic potential, these compounds can also be used as new reagents for the study of the roles of JAKs in vivo.
Macrophages play important roles during the innate immune response and, following detection of a pathogen, are responsible for producing a range of proinflammatory cytokines including TNF-α, IL-1, IL-6, and IL-12 (14). Macrophages detect pathogens via pattern recognition receptors, which interact with different types of pathogen-derived molecules or pathogen-associated molecular patterns. TLRs constitute one of the most important class of pattern recognition receptors in mammalian cells. LPS, a component of Gram-negative bacterial cell walls, acts via TLR4 to activate multiple pathways, including the NF-κB, ERK1/2, p38 MAPK, and IFN regulatory factor signaling cascades, which combine to regulate cytokine production (14). To avoid excess inflammation and tissue damage, as well as to allow the eventual resolution of inflammation, it is important that the production of proinflammatory cytokines is kept under control. This occurs by both the induction of direct intracellular negative feedback mechanisms and by the action of anti-inflammatory cytokines such as IL-10 and IL-1 receptor antagonist (15–19). IL-10 and IL-1 receptor antagonist are also produced by macrophages downstream of the NF-κB and MAPK signaling pathways following LPS stimulation (20–22).
IL-10 is produced by a range of cells including regulatory T cells, B cells, and macrophages. It was initially discovered as a T cell-derived cytokine that could inhibit the secretion of IFN-γ by Th1 cells (23); however, further work demonstrated that it was also a potent inhibitor of cytokine production by macrophages stimulated with TLR agonists (19). Binding of IL-10 to the IL-10R promotes the activation of JAK1 and Tyk2, resulting in the phosphorylation of STAT3. Although it is clear that STAT3 is required for the repressive effects of IL-10 on TLR-mediated cytokine production (24, 25), exactly how this results in the repression of proinflammatory cytokine secretion is not fully established. It is likely, however, that IL-10, through STAT3, induces the expression of genes that can repress cytokines, and roles for ETV3 and NFIL-3 have been suggested in this respect (26, 27). The importance of IL-10 in maintaining the correct balance of pro- and anti-inflammatory mechanisms has been demonstrated by the findings that IL-10 knockout in mice results in the development of inflammatory bowel disease and increased susceptibility to models of infection and endotoxic shock (reviewed in Ref. 24).
Given the importance of IL-10 in the negative feedback control of cytokine production in response to TLR signals, inhibition of JAKs could have a proinflammatory effect in macrophages following TLR stimulation. It is possible, however, that roles for JAKs in mediating positive feedback signals from other cytokines, such as type I IFNs, could mask this effect. We therefore examined the role that JAKs play in modulating cytokine production by macrophages.
Materials and Methods
Inhibitors
Ruxolitinib and Tofacitinib were obtained from Selleck Chemicals, and stock solutions were prepared in DMSO. AG-490 was purchased from Calbiochem.
Kinase selectivity profiling for Ruxolitinib and Tofacitinib was carried out as described previously (http://www.kinase-screen.mrc.ac.uk/) (28). Briefly, protein kinase assays were carried out at room temperature (21°C) and were linear with respect to time and enzyme concentrations under the conditions used. Assays were performed for 40 min using a Biomek 2000 Laboratory Automation Workstation in a 96-well format (Beckman Instruments, Palo Alto, CA). The concentration of magnesium acetate in the assays was 10 mM, whereas the concentration of [γ-33P]ATP (800 cpm/pmol) used was selected to be close to the kinase’s Km for ATP. Assays were initiated with Mg2+ATP and stopped by the addition of 5 μl 0.5 M orthophosphoric acid. Aliquots were then spotted onto P30 filter mats, washed four times in 75 mM phosphoric acid to remove ATP, once in methanol, then dried, and counted for radioactivity. Data are reported in Supplemental Table I. To determine IC50 values, JAK2, TrkA, IL-1R–associated kinase 1 (IRAK1), and MARK3 activities were determined at 10 inhibitor concentrations ranging from 3 pM to 100 μM as indicated. IC50 values of Ruxolitinib and Tofacitinib against the different JAK isoforms was carried out by Reaction Biology (Malvern, PA) using an ATP concentration of 10 μM.
Cell culture
Primary bone marrow-derived macrophages (BMDMs) were isolated as described previously (29). IL-10 mice were obtained from The Jackson Laboratory and IFNαβR (IFNar1) mice from the U.K. National Institute for Medical Research (18). To obtain BMDMs, bone marrow cells were maintained on bacterial grade plates for 7 d in DMEM supplemented with 10% heat-inactivated FBS (Biosera), 2 mM l-glutamine, 100 U/ml penicillin G, 100 mg/ml streptomycin, 0.25 mg/ml amphotericin (Invitrogen), and 5 ng/ml mCSF (R&D Systems). Adherent cells were then replated on tissue culture grade plates in fresh media and used 24 h after replating. Where shown, cells were incubated in the indicated concentrations of Ruxolitinib, Tofacitinib, or IL-10 neutralizing Ab for 1 h before stimulation. Cells were stimulated with 100 ng/ml IL-10, 500 U/ml IFN-β, or 100 ng/ml LPS for the times indicated. LPS was derived from Escherichia coli strain O26:B6 (L2654; Sigma-Aldrich).
Quantitative PCR
Cells were lysed, and total RNA was purified using the Qiagen microRNeasy system, according to the manufacturer’s protocols. Total RNA (0.5–1 μg) was reversed transcribed using iScript (Bio-Rad), and quantitative PCR (Q-PCR) was carried out using Sybergreen-based detection methods. Levels of 18s were used as a normalization control, and fold induction was calculated using the equation:
where E is the efficiency of the PCR, ct is the threshold cycle, u is the mRNA of interest, r is the reference gene (18s RNA), s is the sample, and c is the average of the unstimulated wild-type control samples. Primer sequences have been described previously (20).
Immunoblotting
BMDMs were lysed directly into SDS sample buffer and aliquots run on 10% polyacrylamide gels using standard methods. Proteins were transferred onto nitrocellulose membranes and specific proteins detected by immunoblotting. Abs against phospho-ERK1/2, phospho-p38, phospho-JNK, phospho-Y705 STAT3, phospho-S727 STAT3, phospho-Y701 STAT1, phospho-IκBα, phospho-p105, total ERK1/2, total p38α, total STAT3, total IκBα, and GAPDH were obtained from Cell Signaling Technology. HRP-conjugated secondary Abs were purchased from Pierce (Cheshire, U.K.), and detection was performed using the ECL reagent from Amersham Biosciences (Buckinghamshire, U.K.).
Cytokine measurements
TNF, IL-6, IL-10, IL-12p40, and IL-12p70 were measured using a multiplex-based assay from Bio-Rad, according to the manufacturer’s protocols.
Results
Ruxolitinib is a selective JAK inhibitor and blocks IL-10 signaling in cells
Ruxolitinib has been reported as a specific inhibitor of JAK1 and 2 (8). To further examine its selectivity, Ruxolitinib was profiled in vitro against a panel of 121 kinases that included JAK2 as a representative of the JAK family. This confirmed that Ruxolitinib was highly selective for JAK2 at 0.1 μM (Fig. 1A). At this concentration, 97% inhibition of JAK2 was observed, whereas only one other kinase, TrkA, was inhibited by >50%. At 1 μM, Ruxolitinib did show some weak activity against some other kinases in the panel, including IRAK1 and MARK3. To further examine this, IC50 values were determined for the JAK2, TrkA, IRAK1, and MARK3. Ruxolitinib inhibited JAK2 with an IC50 of 0.56 nM, which compared with 192 nM for TrkA (Fig. 1B). IC50 values for IRAK1 and MARK3 were considerably higher at 565 and 617 nM, respectively.
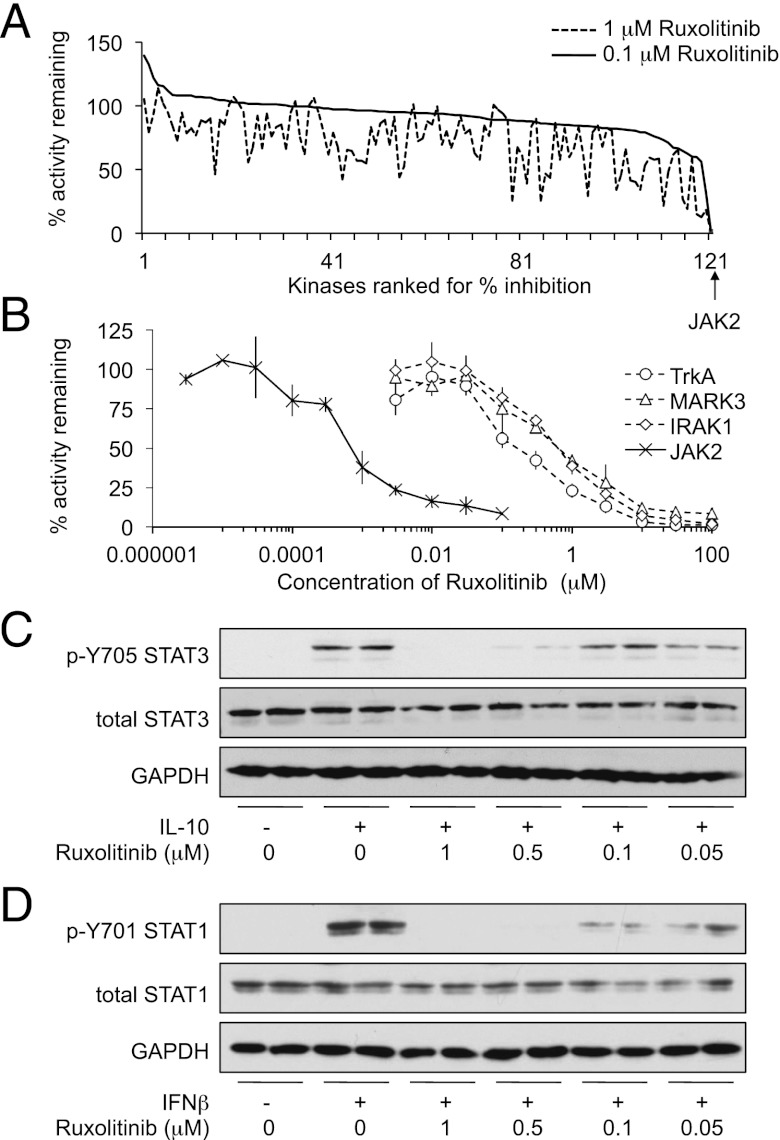
Ruxolitinib selectively inhibits JAK and blocks STAT phosphorylation in BMDMs. (A) Ruxolitinib was screened at 0.1 or 1 μM against a panel of 121 kinases in vitro as described in Materials and Methods. For the graph in (A), kinases were then ranked in order of the percentage activity remaining at 0.1 μM Ruxolitinib. (B) JAK2, IRAK1, MAPK3, and TrkA kinases assays were carried out in the indicated concentrations of Ruxolitinib as described in Materials and Methods. Percentage activity was calculated relative to the activity in the absence of Ruxolitinib. Data are reported in Supplemental Table I. Error bars represent the SD of four replicates. (C) BMDMs were isolated from wild-type mice and incubated in the indicated concentrations of Ruxolitinib for 1 h before stimulation with 100 ng/ml IL-10 for 30 min. Levels of GAPDH as well as total and phospho-Tyr705 STAT3 were determined by immunoblotting. (D) As in (C), but cells were stimulated with 500 U/ml IFN-β instead of IL-10.
In vitro IC50 values for kinase inhibitors are frequently lower than the cellular IC50; this is due to differences between the in vitro and cellular ATP concentrations as well as potential issues with the cell permeability of some inhibitors. It is therefore also necessary to determine the optimal concentration required to block kinase activity in cells. IL-10 stimulates the phosphorylation of Y705 in STAT3 via the activation of JAK1 and Tyk2. To determine the concentration of Ruxolitinib required to inhibit JAKs in macrophages, BMDMs were stimulated with recombinant IL-10 in the presence of various concentrations of Ruxolitinib. This demonstrated that 0.5 μM Ruxolitinib was required to completely inhibit IL-10–induced STAT3 phosphorylation in cells (Fig. 1C). IFN-β can also activate JAK1/Tyk2 signaling in macrophages and lead to the phosphorylation of STAT1. A similar concentration of Ruxolitinib was required to block IFN-β–induced STAT1 phosphorylation as was required to block IL-10–induced STAT3 phosphorylation (Fig. 1D).
LPS-induced STAT phosphorylation is dependent on JAKs
LPS has previously been shown to promote the phosphorylation of STAT3 on Y705. This process is dependent on IL-10 production downstream of TLR4, because it does not occur in macrophages cultured from IL-10 knockout mice (20, 30). In response to LPS, Ruxolitinib did not affect the TLR4-induced activation of the ERK1/2, p38, or JNK MAPK cascades. There were also no major effects on the activation of the NF-κB pathway as judged by degradation of IκBα or the phosphorylation of the IKKβ substrates p105 and IκBα (Fig. 2). In contrast, Ruxolitinib completely blocked the phosphorylation of STAT3 on Y705 in response to LPS (Fig. 2). The phosphorylation of STAT3 on S727, a site phosphorylated independently JAKs, was however not blocked by Ruxolitinib pretreatment. LPS also induced STAT1 Y701 phosphorylation, and this was blocked by Ruxolitinib.
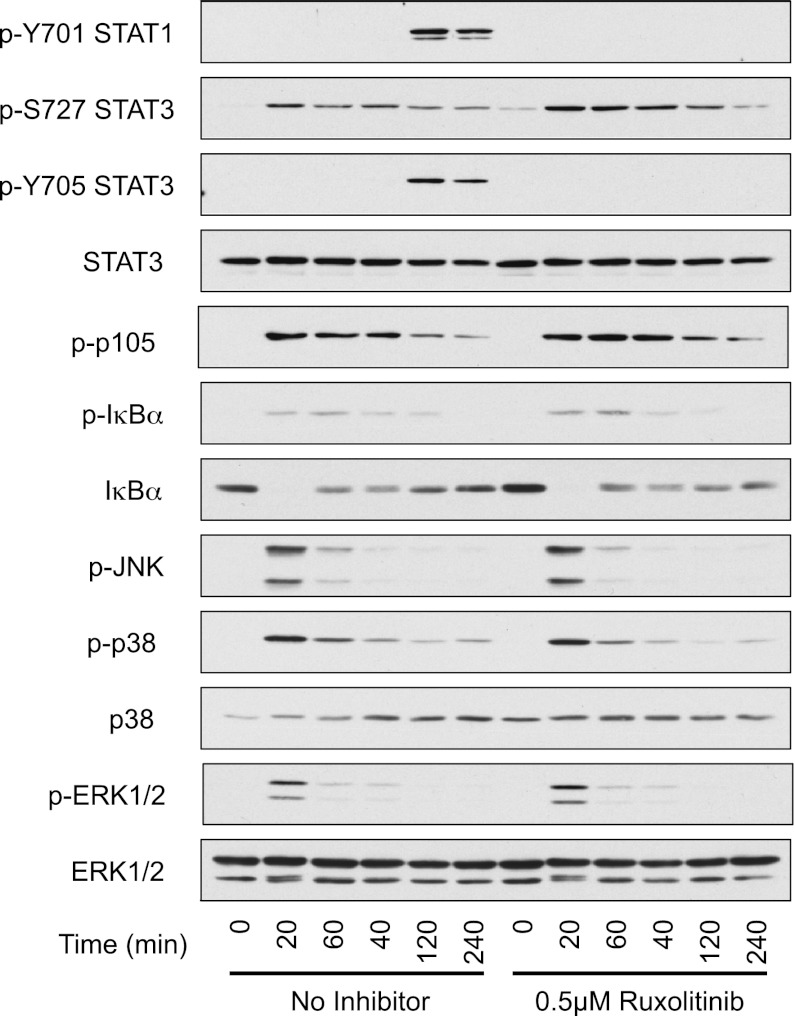
Ruxolitinib inhibits LPS-mediated STAT3 phosphorylation but not MAPK or NF-κB activation. BMDMs were prepared from wild-type mice and where indicated were treated with 0.5 μM Ruxolitinib for 1 h. Cells were then stimulated for the indicated times with 100 ng/ml LPS. Levels of STAT3 (total, p-Y705, and p-S727), STAT1 (p-Y701), ERK1/2 (total and phospho), phospho-JNK, p38 (total and phospho), phospho-p105, and IκB (total and phospho) were determined by immunoblotting.
IFN-β is required for the sustained production of IL-10 in response to LPS
The ability of Ruxolitinib to block LPS-induced phosphorylation of STAT3 on Y705 could result from a reduction in IL-10 production, an inhibition of IL-10 signaling, or a combination of both mechanisms. The effect of Ruxolitinib on the LPS-stimulated transcription of IL-10 was therefore measured. Interestingly, although Ruxolitinib did not affect the initial transcription of IL-10, it did reduce the transcription of IL-10 at later time points (Fig. 3A). One possible explanation for this could be that the IL-10 secreted in response to LPS exerts a positive feedback on IL-10 transcription. To test this, BMDMs were stimulated with LPS in the presence of an IL-10 neutralizing Ab to inhibit restimulation of the BMDMs by any secreted IL-10 (20). The neutralization of IL-10 did not affect the mRNA levels of IL-10 induced in response to LPS (Fig. 3B), indicating that the effect of Ruxolitinib on IL-10 transcription was independent of IL-10 signaling. The IL-10 neutralizing Ab did, however, increase the LPS-induced transcription of IL-6 and IL-12, confirming that restimulation of the macrophages by endogenous IL-10 had been blocked (data not shown).
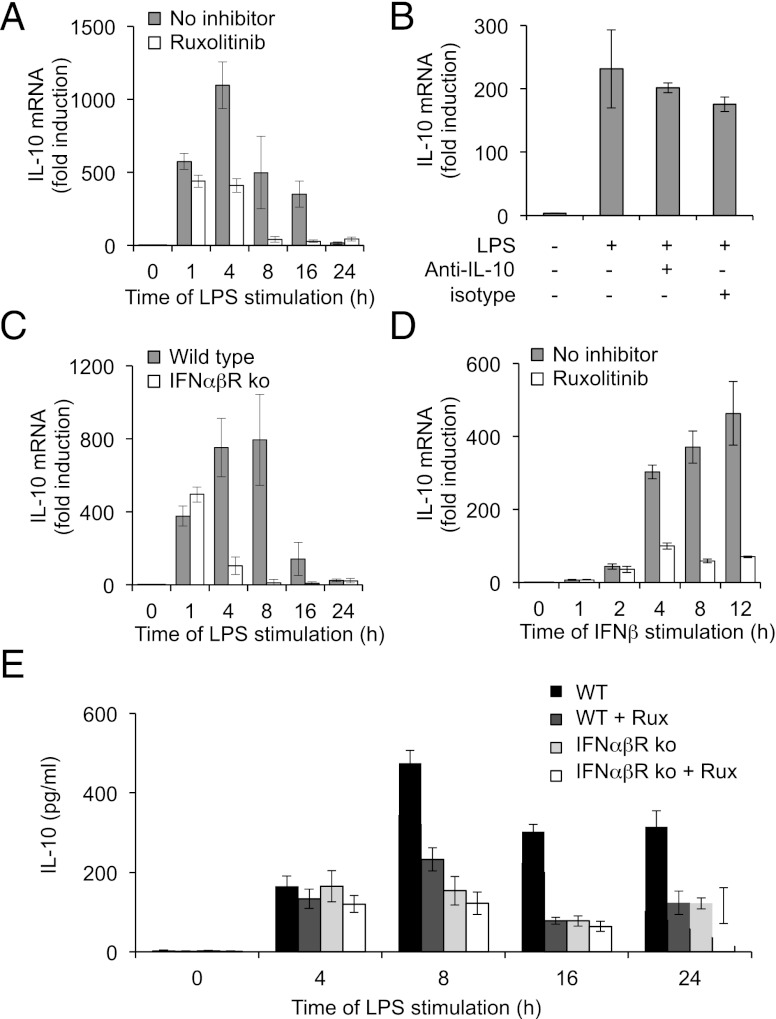
Ruxolitinib inhibits sustained IL-10 production in response to LPS. (A) BMDMs were isolated from wild-type mice and where indicated were treated with 0.5 μM Ruxolitinib. Cells were then stimulated with 100 ng/ml LPS for the indicated times. Total RNA was isolated, and IL-10 mRNA levels were determined by Q-PCR. (B) BMDMs were treated with either 100 ng/ml LPS alone or in the presence of 10 μg/ml of either an IL-10 neutralizing Ab (anti–IL-10) or isotype control. After 16 h, cells were lysed, and IL-10 mRNA levels were measured by Q-PCR. (C) BMDMs were isolated from wild-type mice or IFNαβR knockout mice. Cells were stimulated with 100 ng/ml LPS for the indicated times, and total RNA isolated and IL-10 mRNA levels determined by Q-PCR. (D) BMDMs were isolated from wild-type mice and where indicated were treated with 0.5 μM Ruxolitinib. Cells were then stimulated with 500 U/ml IFN-β for the indicated times, and IL-10 mRNA levels were determined by Q-PCR. BMDMs were isolated from wild-type and IFNαβR knockout mice and were treated with 0.5 μM Ruxolitinib. Cells were then stimulated with 100 ng/ml LPS for the indicated times, and levels of IL-10 secreted into the media were determined by multiplex. In all panels, error bars represent the SD from independent cultures from four mice per genotype.
LPS also promotes the induction of IFN-β, a type I IFN that can activate JAK signaling (1, 31). To test the involvement of an IFN-β feedback loop in LPS-induced IL-10 transcription, BMDMs were isolated from type I IFNR (IFNαβR) knockout mice. Comparison of the IFNαβR knockouts with wild-type macrophages showed that type I IFN signaling was not required for the initial transcription of IL-10 in response to LPS, although the late phase of IL-10 transcription was decreased (Fig. 3C). Consistent with a role for IFN-β in IL-10 transcription, stimulation of wild-type BMDMs with IFN-β led to the induction of IL-10 transcription, and this was greatly reduced by pretreatment of the cells with Ruxolitinib (Fig. 3D). To understand the effects that these changes in IL-10 transcription have on IL-10 secretion, the levels of IL-10 in the media were analyzed after LPS treatment. LPS resulted in IL-10 secretion from wild-type cells. At 4 h after LPS treatment, this was unaffected by Ruxolitinib, however, at later time points (8–24 h). Ruxolitinib treatment resulted in a decrease in the amount of IL-10 secreted into the media (Fig. 3E). In line with this, IFNαβR knockout did not greatly affect IL-10 secretion at 4 h but did result in decreased levels of IL-10 relative to wild-type cells at later time points. Consistent with the suggestion that Ruxolitinib affects prolonged IL-10 production by inhibition of IFN-β signaling, Ruxolitinib did not affect IL-10 levels secreted from IFNαβR knockout macrophages (Fig. 3E). Although Ruxolitinib exhibits a high degree of selectivity for JAKs, the possibility that it could act in a JAK-independent manner in cells cannot be excluded. Therefore, a structurally unrelated JAK inhibitor, Tofacitinib, was also tested. Like Ruxolitinib, Tofacitinib is a highly selective Jak inhibitor, as evidenced by screening against a panel of 121 different kinases in vitro (Supplemental Fig. 1). Tofacitinib was able to block both IL-10–stimulated STAT3 phosphorylation and IFN-β–stimulated STAT1 phosphorylation in BMDMs (Fig. 4A, ,4B).4B). In contrast to Ruxolitinib, however, a higher concentration of Tofacitinib was required to block IL-10–stimulated STAT3 phosphorylation than IFN-β–stimulated STAT1 phosphorylation. This may reflect the different specificities of the two inhibitors. In vitro, Tofacitinib was found to inhibit Jak3 and Jak1 with a lower IC50 than for Jak2 and TYK2. In contrast, Ruxolitinib inhibits JAK1, JAK2, and Tyk2 with similar IC50 values but is less potent against JAK3 (Fig. 4C, Supplemental Fig. 1). In agreement with the results obtained for Ruxolitinib, Tofacitinib did not affect the initial phase of IL-10 transcription but inhibited the levels of IL-10 mRNA at later time points (Fig. 4D). In line with this, IL-10 secretion at 8 and 16 h after LPS stimulation was also decreased by Tofacitinib treatment (Fig. 4E).
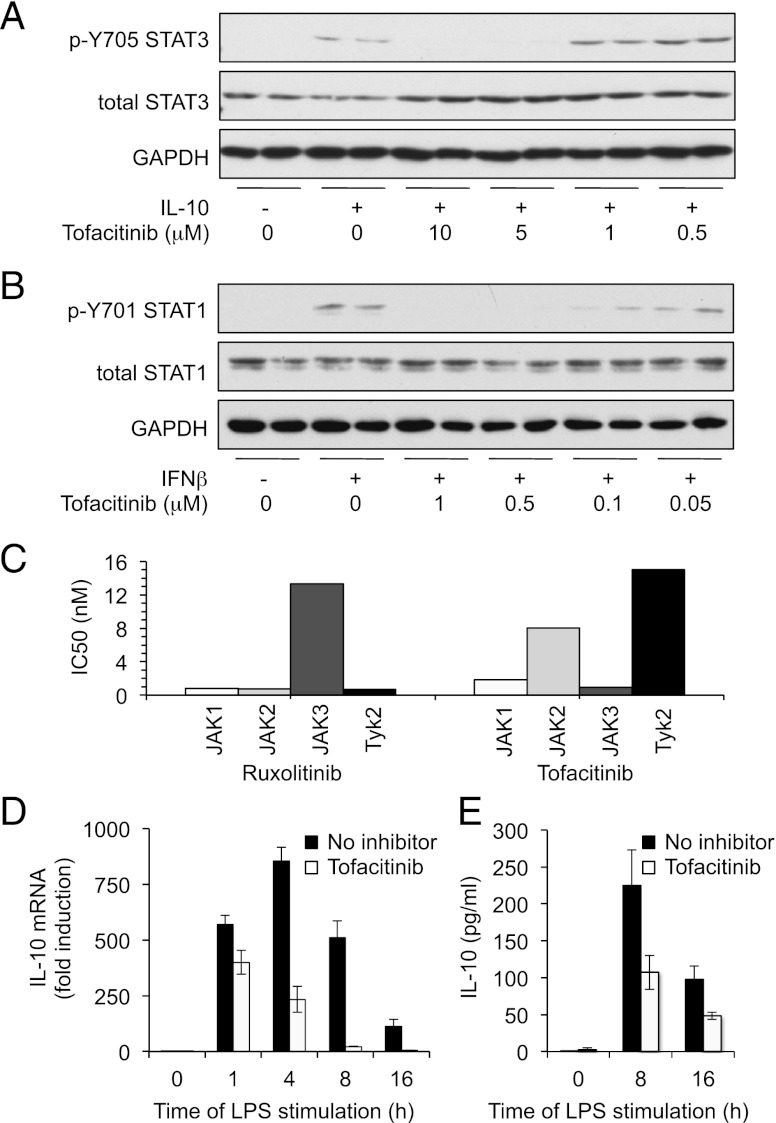
Tofacitinib inhibits sustained IL-10 production in response to LPS. BMDMs were isolated from wild-type mice and incubated in the indicated concentrations of Tofacitinib for 1 h before stimulation with 100 ng/ml IL-10 (A) or 500 U/ml IFN-β (B) for 30 min. Levels of GAPDH, STAT3 (total and p-Y705), and STAT1 (total and p-Y701) were determined by immunoblotting. (C) In vitro IC50 values for Tofacitinib and Ruxolitinib were determined as described in the supplemental information. (D) BMDMs were pretreated with 5 μM Tofacitinib for 1 h where indicated. Cells were then stimulated with 100 ng/ml LPS for the indicated times, and IL-10 mRNA levels were measured by Q-PCR. (E) As in (D), except the levels of IL-10 secreted into the culture media was measured. For both (D) and (E), error bars represent the SD of independent cultures from four mice.
Type I IFNs are reported to induce the tyrosine phosphorylation of multiple STATs including both STAT1 and STAT3 (32, 33). Downstream of LPS, however, STAT3 Y705 phosphorylation appears to be predominantly IL-10 dependent, because it does not occur in IL-10 knockout mice (20). In agreement with this, LPS-induced Y705 STAT3 phosphorylation was normal in IFNαβR knockout BMDMs (Fig. 5). In contrast, LPS-induced Y701 STAT1 phosphorylation was completely absent in the IFNαβR knockout cells (Fig. 5).
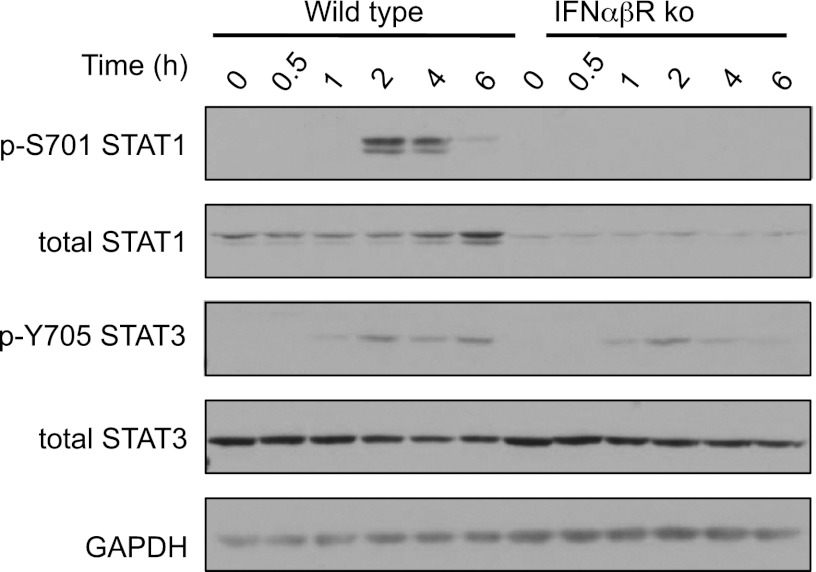
Induction of signaling by IFN-β is required for LPS-induced Tyr phosphorylation of STAT1 but not STAT3. BMDMs were isolated from wild-type or IFNαβR knockout mice and stimulated with 100 ng/ml LPS for the indicated times. Levels of phospho-STAT1, STAT3 and total STAT1, STAT3, and GAPDH were determined by immunoblotting.
Ruxolitinib inhibits the ability of IL-10 to block proinflammatory cytokine production
The above experiments demonstrate that Ruxolitinib can potentially affect the IL-10 and IFN-β feedback loops downstream of LPS in macrophages. We therefore next determined whether Ruxolitinib could block the inhibitory action of IL-10 on LPS-induced cytokine production. To avoid complications from endogenously produced IL-10, BMDMs were isolated from IL-10 knockout mice. These cells were stimulated with either LPS or a combination of LPS and exogenous IL-10. As expected, costimulation with IL-10 and LPS greatly reduced the production of TNF-α and IL-6 from these cells compared with stimulation with LPS alone (Fig. 6). Ruxolitinib moderately decreased the production of IL-6 and, to a lesser extent, TNF-α by LPS in IL-10 knockout BMDMs. Despite this, Ruxolitinib blocked the inhibitory effects of IL-10 on both TNF-α and IL-6 production (Fig. 6).
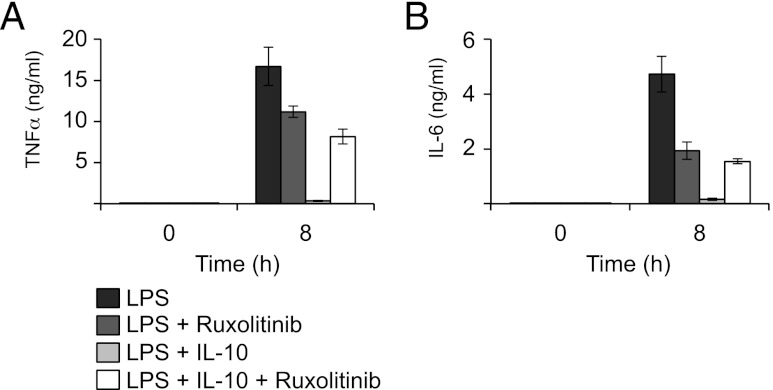
Ruxolitinib prevents the IL-10–mediated repression of TLR4-induced cytokine production. BMDMs were isolated from IL-10 knockout mice and where indicated preincubated for 1 h in 0.5 μM Ruxolitinib. Cells were then stimulated with either 100 ng/ml LPS or a combination of LPS and 100 ng/ml IL-10 for 6 h. Secreted levels of TNF-α (A) and IL-6 (B) were determined using a Luminex-based multiplex assay. Error bars represent the SD of independent BMDM cultures from four mice.
JAKs modulate LPS induced proinflammatory cytokine production via inhibition of both IL-10 and IFN-β signaling
LPS stimulates IL-10 production by macrophages (20), thus setting up an autocrine feedback loop to dampen proinflammatory cytokine production. As a result of this, BMDMs from IL-10 knockout mice secrete more proinflammatory cytokines in response to LPS relative to wild-type cells (Fig. 7). Because Ruxolitinib inhibits IL-10–induced STAT3 Y705 phosphorylation (Fig. 1C), it should mimic some or all of the effects of IL-10 knockout on cytokine production. In wild-type BMDMs, LPS induced both TNF-α mRNA transcription and secretion of TNF-α protein (Fig. 6A). LPS-induced TNF-α production was greatly increased in IL-10 knockout cells relative to wild-type macrophages (Fig. 7A). In wild-type cells, Ruxolitinib increased TNF-α mRNA and secreted protein levels to similar values to those seen in the IL-10 knockout BMDMs. Consistent with Ruxolitinib affecting LPS-induced TNF secretion predominantly via the inhibition of IL-10 signaling, Ruxolitinib had little effect of TNF-α production in IL-10 knockout cells (Fig. 7A). Similar effects were seen for IL-12p40 and p70 secretion (Fig. 7B, ,7C).7C). Interestingly, however, for induction of IL-12p35 and IL-12p40 mRNA, Ruxolitinib did not increase the peak mRNA levels in the wild-type cells to the same levels as those seen in the IL-10 knockout cells. In line with this, Ruxolitinib decreased the peak amount of LPS-induced IL-12 mRNA in IL-10 knockout cells to a similar level as obtained in Ruxolitinib-treated wild-type cells (Fig. 7B, ,7C,7C, right panels). This reduction in IL-10 knockout cells could indicate that inhibition of JAKs with Ruxolitinib affects an additional IL-10 independent pathway that promotes IL-12 transcription. A similar effect was also observed for IL-6 mRNA induction (Fig. 7D). For IL-6, however, Ruxolitinib treatment had this effect on the secreted levels of IL-6 in addition to the effects on the mRNA; for both wild-type and IL-10 knockout cells, Ruxolitinib treatment resulted in levels of IL-6 that were intermediate between the wild-type and IL-10 knockout cells in the absence of inhibitor (Fig. 7D).
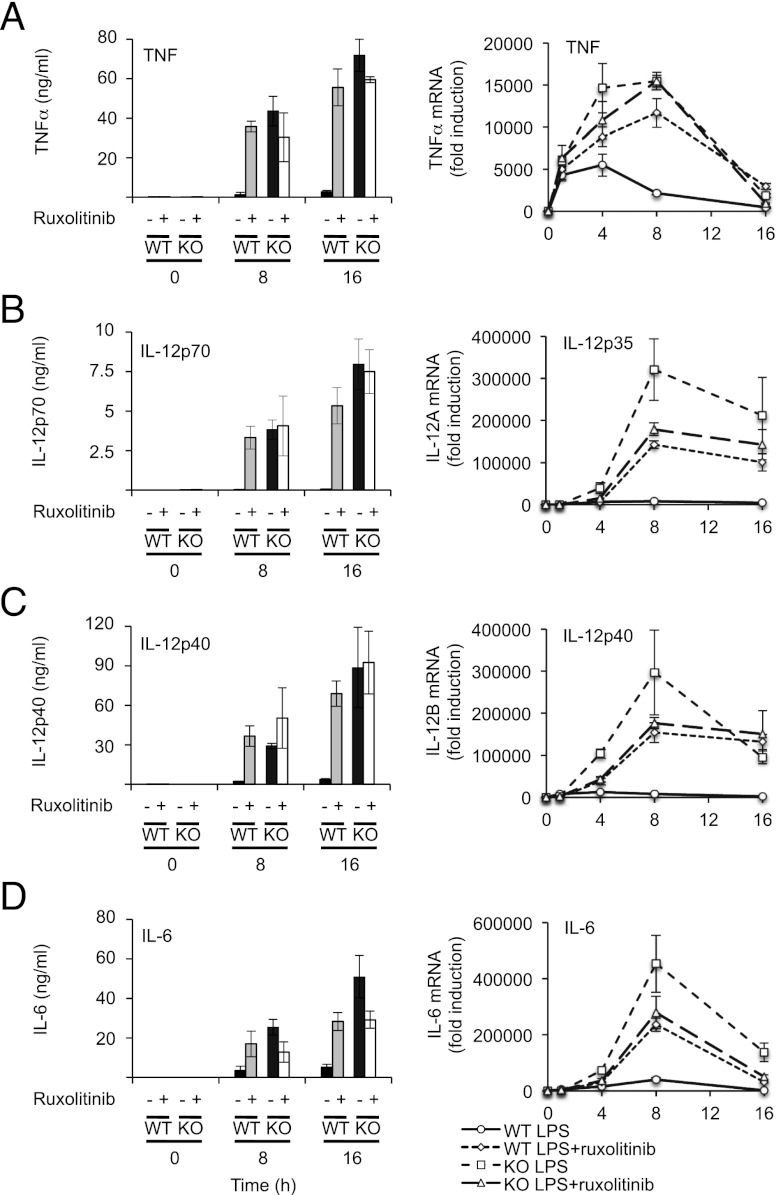
Ruxolitinib treatment mimics the effect of IL-10 knockout on LPS-mediated cytokine production. Wild-type or IL-10 knockout BMDMs were pretreated with 0.5 μM Ruxolitinib where indicated and then stimulated with 100 ng/ml LPS for the indicated times. Levels of TNF-α (A), IL-12p70 (B), IL-12p40 (C), and IL-6 (D) secreted into the media were determined by Luminex-based multiplex assay (left panels). Levels of TNF-α (A), IL-12p35 (B), IL-12p40 (C), and IL-6 (D) mRNA were determined by Q-PCR (right panels). Error bars represent the SD of independent BMDM cultures from four mice per genotype.
As shown in Fig. 4, Tofacitinib can also block IL-10 signaling. Similar to Ruxolitinib, when used at a concentration able to inhibit STAT3 phosphorylation, Tofacitinib increased TNF and IL-12 production in response to a prolonged stimulation with LPS. Tofacitinib had less effect on IL-6 than Ruxolitinib, although treatment with Tofacitinib did increased IL-6 secretion inhibitor following 24 h of LPS stimulation (Supplemental Fig. 1).
Taken together, these effects suggest that although inhibition of JAKs can block the IL-10–mediated feedback loop on TLR4-induced cytokine production, JAKs are also required in alternative pathways that may help sustain the production of other cytokines, particularly IL-6 (Fig. 8). IFN-β is one such potential pathway, so the ability of IFN-β to directly stimulate IL-6 transcription was measured. Treatment of BMDMs with exogenous IFN-β was able to stimulate IL-6 transcription, and this was inhibited by pretreatment of the cells with Ruxolitinib. To further examine a role for IFN-β, type I IFNR knockout (IFNαβR) BMDMs were used. Loss of the IFNαβR did not have any major effects on the secreted levels of TNF-α following LPS stimulation (Fig. 9A). Similar results were also obtained for IL-12p40 and IL-p70 secretion (Fig. 9B, ,9C).9C). IFN-β did, however, contribute to the sustained production of IL-6; levels of IL-6 were lower in IFNαβR macrophages relative to wild-type cells after LPS stimulation. In line with Fig. 7D, Ruxolitinib increased IL-6 production from wild-type cells. Ruxolitinib also increased IL-6 production from IFNαβR knockout macrophages, although unexpectedly, these levels were still lower than from wild-type Ruxolitinib-treated macrophages (Fig. 9D).
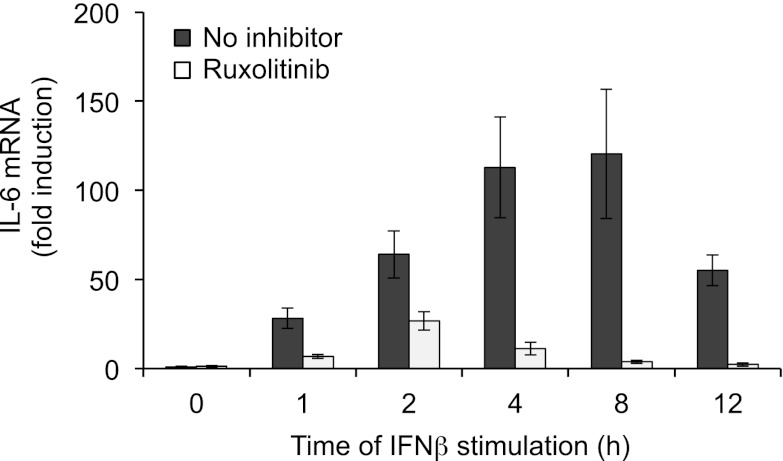
IFN-stimulated transcription of IL-6. Wild-type BMDMs were incubated for 1 h in 0.5 μM Ruxolitinib where indicated. Cells were then stimulated with 500 U/ml IFN-β for the indicated times and IL-6 mRNA levels determined by Q-PCR. Error bars represent the SD of independent BMDM cultures from four mice.
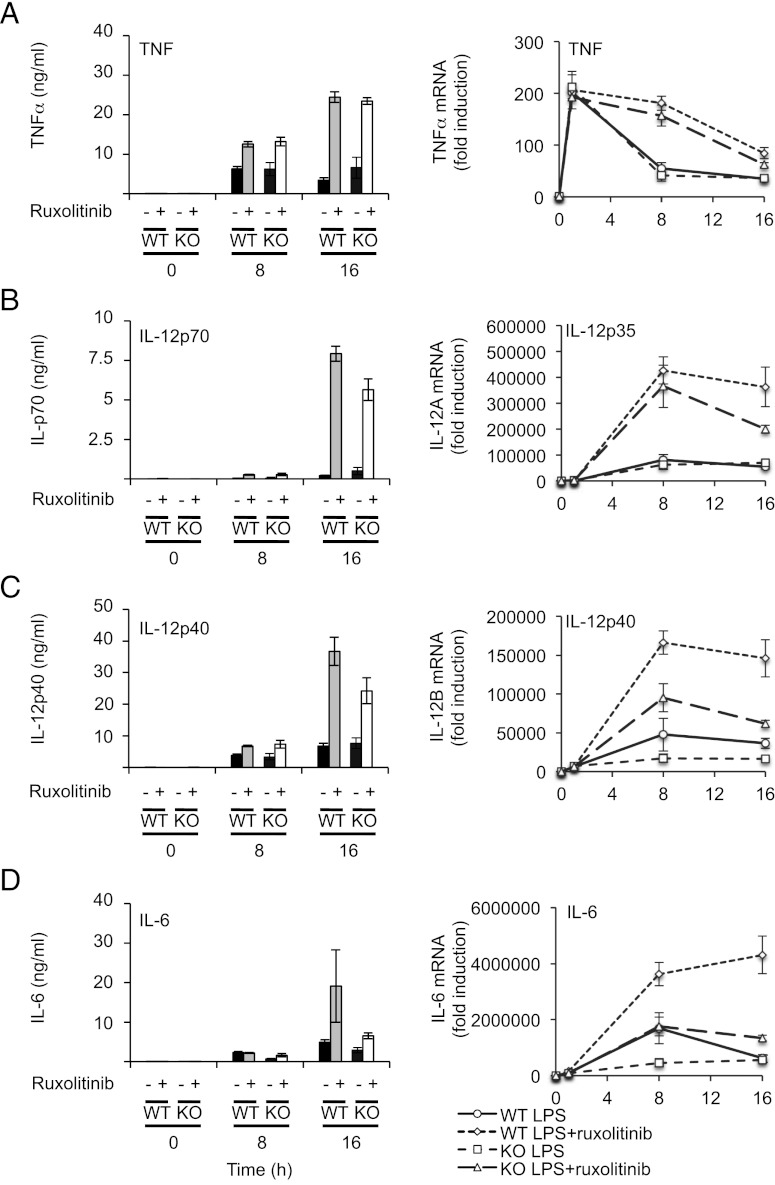
IFNαβR knockout affects LPS-stimulated IL-6 but not TNF-α or IL-12 production. Wild-type or IL-10 knockout BMDMs were pretreated with 0.5 μM Ruxolitinib where indicated and then stimulated with 100 ng/ml LPS for the indicated times. Levels of TNF-α (A), IL-12p70 (B), IL-12p40 (C), and IL-6 (D) secreted into the media were determined by Luminex-based multiplex assay (left panels). Levels of TNF-α (A), IL-12p35 (B), IL-12p40 (C), and IL-6 (D) mRNA were determined by Q-PCR (right panels). Error bars represent the SD of independent BMDM cultures from four mice per genotype.
Discussion
TLR4 activation in macrophages is a potent stimulus for the production of cytokines. The regulation of cytokine production by TLR4 is, however, complex and involves both the initial signaling pathways activated by the TLR, such as the MAPK, NF-κB, and IFN regulatory factor pathways as well as multiple autocrine feedback pathways. We show in this paper that Ruxolitinib, a selective JAK inhibitor, modulates LPS-induced cytokine production in macrophages by interfering with IL-10 and IFN-β feedback pathways. Although our data suggest that IL-10 and IFN-β may represent two of the predominant feedback mechanisms that use JAKs in this system, our data do not rule out an involvement for other stimuli or cytokines not directly addressed in this study but which can activate JAKs in macrophages. We show that IFN-β contributes to the sustained production of IL-10 in response to LPS and that IFN-β stimulation alone is sufficient to induce IL-10 mRNA transcription. Although this feedback pathway operates for LPS-induced IL-10 production, it is worth noting that not all TLRs induce significant amounts of IFN-β, and so this may not be a general mechanism to all TLRs (34). Significantly, IFN-β was not required for the initial transcription of IL-10 in response to LPS. This initial phase of IL-10 transcription has been linked to several transcription factors including Sp1, CREB, and NF-κB (20, 21, 35–40). In contrast, the sustained transcription of IL-10 requires type I IFNs as indicated by the decreased production of IL-10 in IFNαβR macrophages relative to wild-type cells at later time points (Fig. 3D). The ability of type I IFNs to promote IL-10 transcription was inhibited by Ruxolitinib, indicating a role for JAKs in this process. This could suggest a role for STAT1 phosphorylation, because LPS-induced phosphorylation of STAT1 at Y701 was dependent on stimulation via the type I IFNR (Fig. 5). This most likely reflects a role of IFN-β rather than IFN-α because LPS induces IFN-β but little IFN-α in BMDMs. In line with this, earlier studies have shown that LPS-induced STAT1 Y701 phosphorylation requires protein synthesis and can be blocked by neutralizing Abs against IFN-β (34, 41) and that LPS can induce IFN-β–dependent gene transcription (34, 42). STAT binding sites have been identified in the IL-10 promoter and both STAT1 and STAT3 have been implicated in IL-10 transcription (43–47). The synergistic induction of IL-10 by LPS and type I IFNs is blocked by the knockout of STAT1 in BMDMs (45). In addition, it has been shown that the direct induction of IL-10 transcription by IFN-α is reduced in STAT1 knockout cells (46). Thus, it is possible that IFN-β could directly induce IL-10 transcription via the activation of STAT1, allowing it to bind the IL-10 promoter. Our data do not, however, exclude the possibility that the effect of IFN-β on IL-10 transcription is indirect and requires the production of an IFN-inducible protein. With respect to this, it has recently been reported that an IFN-β–dependent induction of IL-27 is required to promote IL-10 transcription downstream of TLR signaling (46).
The timing of IL-10 production and STAT3 phosphorylation is significant regarding the feedback control of the macrophages by IL-10. Much of the ability of IL-10 to repress TLR-induced cytokine production is thought to require the phosphorylation of STAT3 by JAK1/Tyk2 downstream of the IL-10R. Because IFN-β does not affect initial IL-10 transcription or secretion of IL-10 in response to LPS, it is not required for LPS-induced STAT3 phosphorylation, at least within 4–6 h of stimulation. It therefore seems unlikely that in the BMDM system studied, IFNαβR knockout affects the endogenous IL-10–dependent negative feedback loop on TLR-induced cytokine production over the relatively short time course studied in our experiments. This is borne out by the observation that knockout of the IFNαβR did not affect TNF-α production or the ability of Ruxolitinib to increase TNF-α production by LPS-stimulated BMDMs. In contrast, IFN-β does appear to play a separate role in sustaining the production of IL-6 and, to a much lesser extent, IL-12. This is also reflected in the ability of IFN-β alone to directly stimulate the transcription of these genes in BMDMs; IFN-β was able to directly induce IL-6 transcription but only weakly promote TNF-α or IL-12 transcription in macrophages (Fig. 8; data not shown). A role for an IFN-β feedback mechanism in maintaining IL-12 transcription has been demonstrated previously in dendritic cells (48). Interestingly, in this study, the transcription of both IL-12p35 and IL-12p40 was reduced in response to TLR agonists including LPS by knockout of the type 1 IFNR or STAT1; however, only the secretion of IL-12p70 and not IL-12p40 was decreased (48). This suggests that the levels of IL-12 mRNA were more sensitive to the IFN-β feedback loop than the levels of secreted protein, a finding consistent with what we observed in this study in macrophages. IL-6 transcription has also been linked to IFN-β. Array profiling of LPS-stimulated genes showed that IL-6 mRNA was less strongly increased in IFN-β knockout macrophages (33), whereas an IFN feedback loop has also been shown to be important for IL-6 production in dendritic cells (49).
JAK inhibitors, in particular Tofacitinib, have shown considerable promise in the treatment of autoimmune disorders (12, 50), and an important aspect of this success is their ability to inhibit JAKs in the adaptive immune system. We show in this paper that the inhibition of JAKs using two structurally unrelated compounds, Tofacitinib and Ruxolitinib, can have proinflammatory effects in isolated LPS-stimulated macrophages because of an inhibition of IL-10, resulting in an increase in proinflammatory cytokine production. Both these compounds are highly selective and represent an improvement over AG-490, a tyrosine kinase inhibitor frequently used to inhibit JAKs. AG-490 is less potent than either Tofacitinib or Ruxolitinib and has also been reported to inhibit the epidermal growth factor receptor. In our selectivity screen, AG-490 could also block several Ser/Thr kinases, making its results in cells harder to interpret (Supplemental Table I).
Interestingly, Tofacitinib was found to inhibit IFN-β signaling in macrophages more potently than IL-10 signaling. The reason for this is not clear. Both IL-10 and IFN-β signal via JAK1 and Tyk2. We show in this paper that Tofacitinib can inhibit JAK1 with a similar IC50 to JAK3 but that JAK2 and Tyk2 are less potently inhibited. Of note, other studies have also reported that higher concentrations of Tofacitinib are required to inhibit STAT3 phosphorylation compared with STAT1 phosphorylation in response to IL-6 (50, 51). Because Tofacitinib is less potent against Tyk2 relative to JAK1, one explanation for this could be that Tyk2 has a greater role in the phosphorylation of STAT3 than STAT1. Against this, however, it has been reported that Tyk2 knockout mice show normal IL-10–induced STAT3 phosphorylation but reduced STAT1 phosphorylation in response to IFN (52, 53). Further work will therefore be necessary to resolve these issues.
The effect of JAK inhibitors in vivo will be considerably more complex. The effects of Ruxolitinib have not to our knowledge been studied in an in vivo system in response to TLR stimulation. The effect of Tofacitinib on plasma cytokine levels in response to an i.p. injection of LPS into DBA/1J mice has, however, been reported. In this study, treatment of the mice with 5 mg/kg Tofacitinib reduced the plasma levels of TNF, IL-6, and IL-12 but increased IL-10 levels (50). This is in contrast to our in vitro results with BMDMs using either Tofacitinib or Ruxolitinib. This difference could be due to several reasons, because the in vivo experiment represents a more complex situation than the BMDM cultures. For instance, multiple cell types will contribute to the response to LPS in vivo, and cytokine production from these different cells may not all be regulated in the same way by JAKs. In addition, macrophages in vivo, but not in our BMDM cultures, would be subject to IFN priming, which would be predicted to be blocked by JAK inhibitors; in line with this, STAT1, Tyk2, and IFN-β knockout mice are protected from LPS-induced endotoxic shock (33, 54). Finally, it is not clear how the effective concentration of Tofacitinib in the in vivo experiments compares with the 5 μM concentration used in our experiments. This is an important consideration given that in BMDMs a 10-fold higher concentration was required to block IL-10 signaling relative to IFN-β signaling. Thus, the effective concentration in the in vivo experiment may not have been sufficient to inhibit IL-10 signaling. Further work would be required to resolve these issues.
In summary, we show that in primary macrophage cultures, IFN-β production is required to sustain IL-10 transcription in response to LPS and that this requires IFN-β–induced STAT1 activation. IL-10 then represses LPS-induced proinflammatory cytokine production in a JAK-dependent manner. As a result, JAK inhibitors can promote LPS-induced proinflammatory cytokine production in isolated macrophages.
Acknowledgments
We thank Anne O’Garra (National Institute of Medical Research, London, U.K.) for the IL-10 neutralizing Ab and IFNαβR knockout mice. We also thank the National Centre for Protein Kinase Profiling, Medical Research Council Protein Phosphorylation Unit, University of Dundee (Dundee, Scotland, U.K.) (http://www.kinase-screen.mrc.ac.uk) for analyzing the in vitro specificity of Ruxolitinib and Tofacitinib.
This work was supported by the Medical Research Council (U.K.) and by the Division of Signal Transduction Therapy (University of Dundee), which is supported by grants from AstraZeneca, Boehringer-Ingelheim, GlaxoSmithKline, Pfizer, and Merck KgA.
The online version of this article contains supplemental material.
Abbreviations used in this article:
- BMDM
- bone marrow-derived macrophage
- IRAK1
- IL-1R–associated kinase 1
- Q-PCR
- quantitative PCR.
References
Full text links
Read article at publisher's site: https://doi.org/10.4049/jimmunol.1200310
Read article for free, from open access legal sources, via Unpaywall:
https://www.jimmunol.org/content/jimmunol/189/6/2784.full.pdf
Citations & impact
Impact metrics
Citations of article over time
Alternative metrics
Article citations
The mechanisms behind heatstroke-induced intestinal damage.
Cell Death Discov, 10(1):455, 28 Oct 2024
Cited by: 0 articles | PMID: 39468029 | PMCID: PMC11519599
Review Free full text in Europe PMC
Short-chain fatty acid-producing bacterial strains attenuate experimental ulcerative colitis by promoting M2 macrophage polarization via JAK/STAT3/FOXO3 axis inactivation.
J Transl Med, 22(1):369, 18 Apr 2024
Cited by: 0 articles | PMID: 38637862 | PMCID: PMC11025230
Topical Delivery of Tofacitinib in Dermatology: The Promise of a Novel Therapeutic Class Using Biodegradable Dendritic Polyglycerol Sulfates.
Pharmaceuticals (Basel), 17(1):77, 08 Jan 2024
Cited by: 0 articles | PMID: 38256910 | PMCID: PMC10821331
Butyrate increases methylglyoxal production through regulation of the JAK2/Stat3/Nrf2/Glo1 pathway in castration‑resistant prostate cancer cells.
Oncol Rep, 51(5):71, 05 Apr 2024
Cited by: 0 articles | PMID: 38577936 | PMCID: PMC11019463
Highly Active Myeloid Therapy for Cancer.
ACS Nano, 17(20):20666-20679, 12 Oct 2023
Cited by: 4 articles | PMID: 37824733 | PMCID: PMC10941024
Go to all (82) article citations
Data
Data behind the article
This data has been text mined from the article, or deposited into data resources.
BioStudies: supplemental material and supporting data
Similar Articles
To arrive at the top five similar articles we use a word-weighted algorithm to compare words from the Title and Abstract of each citation.
Cutting edge: involvement of the type I IFN production and signaling pathway in lipopolysaccharide-induced IL-10 production.
J Immunol, 178(11):6705-6709, 01 Jun 2007
Cited by: 149 articles | PMID: 17513714
Inhibition of IL-6 and IL-10 signaling and Stat activation by inflammatory and stress pathways.
J Immunol, 165(9):5227-5237, 01 Nov 2000
Cited by: 78 articles | PMID: 11046056
Signal transducer and activator of transcription 3 is the dominant mediator of the anti-inflammatory effects of IL-10 in human macrophages.
J Immunol, 172(1):567-576, 01 Jan 2004
Cited by: 243 articles | PMID: 14688368
COVID-19 and the potential of Janus family kinase (JAK) pathway inhibition: A novel treatment strategy.
Front Med (Lausanne), 9:961027, 30 Aug 2022
Cited by: 4 articles | PMID: 36111104 | PMCID: PMC9469902
Review Free full text in Europe PMC
Funding
Funders who supported this work.
Medical Research Council (3)
The functions of MAPK signalling pathways in cell regulation and disease
Professor Simon Arthur, MRC Protein Phosphorylation Unit
Grant ID: MC_U127081014
The functions of MAPK signalling pathways in cell regulation and disease
Professor Simon Arthur, MRC Protein Phosphorylation Unit
Grant ID: U.1270(81014)
Grant ID: 999692
Versus Arthritis (1)
Regulation of anti-inflammatory systems
Professor Simon Arthur
Grant ID: 18084