Abstract
Free full text

Air Pollution Particulate Matter Alters Antimycobacterial Respiratory Epithelium Innate Immunity
Abstract
Inhalation exposure to indoor air pollutants and cigarette smoke increases the risk of developing tuberculosis (TB). Whether exposure to ambient air pollution particulate matter (PM) alters protective human host immune responses against Mycobacterium tuberculosis has been little studied. Here, we examined the effect of PM from Iztapalapa, a municipality of Mexico City, with aerodynamic diameters below 2.5 μm (PM2.5) and 10 μm (PM10) on innate antimycobacterial immune responses in human alveolar type II epithelial cells of the A549 cell line. Exposure to PM2.5 or PM10 deregulated the ability of the A549 cells to express the antimicrobial peptides human β-defensin 2 (HBD-2) and HBD-3 upon infection with M. tuberculosis and increased intracellular M. tuberculosis growth (as measured by CFU count). The observed modulation of antibacterial responsiveness by PM exposure was associated with the induction of senescence in PM-exposed A549 cells and was unrelated to PM-mediated loss of cell viability. Thus, the induction of senescence and downregulation of HBD-2 and HBD-3 expression in respiratory PM-exposed epithelial cells leading to enhanced M. tuberculosis growth represent mechanisms by which exposure to air pollution PM may increase the risk of M. tuberculosis infection and the development of TB.
INTRODUCTION
Air pollution and tuberculosis (TB) embody two of the preeminent problems associated with global public health. While exposure to air pollution is responsible for 1 in 8 deaths globally (1), 1.5 million deaths from TB and an estimated 9 million new TB cases were reported in 2014 (2). Aerogenic exposure to air pollution particulate matter (PM) represents a major risk to public health, particularly in rapidly growing cities of countries where TB is endemic, affecting primarily socioeconomically and environmentally disadvantaged communities. PM is a complex mixture of solid and liquid particles that is released into the air during combustion of coal, wood, gasoline, diesel, or fossil fuels, as well as from natural sources (road dust, fires, volcanic emissions, etc.) (3). Based on its aerodynamic diameters, PM can be categorized as PM0.1 (<0.1 μm, ultrafine nanoparticles), PM2.5 (<2.5 μm, fine), and PM10 (<10 μm) (4).
Epidemiological studies have linked inhalation exposure to cigarette smoke (5,–7), indoor air pollution (8, 9), and urban air pollution (10) with increased risk of TB development. Mechanistic studies have demonstrated that cigarette smoke impairs antimycobacterial immune responses by decreasing the production of tumor necrosis factor alpha (TNF-α) and interleukin 12 (IL-12) and increasing the production of IL-10. These alterations have been shown to enhance the growth of Mycobacterium tuberculosis, the causative agent of TB, in in vitro-infected human alveolar macrophages and inhalation-infected mice (11).
In vitro and in vivo infection with M. tuberculosis stimulates respiratory epithelial cells to initiate antimycobacterial responses, such as the secretion of chemokines, cytokines, and antimicrobial peptides. A549 cells, a type II alveolar epithelial cell line, produce IL-8 and monocyte chemotactic protein 1 (MCP-1), which function as chemoattractants for neutrophils, T cells, basophils, and monocytes, in the early stages of M. tuberculosis infection (12, 13). In addition, A549 cells produce human β-defensin 2 (HBD-2) and HBD-3 upon exposure to mycobacterial lipids and different strains of M. tuberculosis (14, 15). HBD-2 and HBD-3 are small cationic peptides that belong to the family of antimicrobial peptides (16). Due to their antimicrobial and immunomodulatory properties, HBD-2 and HBD-3 play important roles in the early control of M. tuberculosis infection (17) by decreasing the pulmonary bacterial load, as shown in experimentally infected rodents (18).
We recently described immunomodulatory effects of diesel exhaust particles (DEP), one of the components of urban ambient PM2.5, on human antimycobacterial immunity. Exposure of peripheral blood mononuclear cells to DEP impaired the expression of M. tuberculosis-induced Toll-like receptor 3 (TLR-3), TLR-4, TLR-7, and TLR-10 and the NF-κB-induced expression of cytokines and chemokines, such as interferon gamma (IFN-γ), TNF-α, IL-1β, and IL-6, that perform key functions in the control of M. tuberculosis (19).
In the current study, we assessed the effects of exposure of A549 cells to PM2.5 and PM10 ambient air pollution from Mexico City on the production of the antimicrobial peptides HBD-2 and HBD-3, as well as of IL-8 and MCP-1, in response to M. tuberculosis. We observed that exposure of A549 cells to PM induced cellular senescence that resulted in downregulation of HBD-2 and HBD-3 expression and, subsequently, impaired the control of M. tuberculosis growth.
MATERIALS AND METHODS
Cell culture.
Human type II alveolar epithelial cells (A549; ATCC, Manassas, VA, USA) were cultured in complete medium consisting of Kaighn's modification of Ham's F-12 medium (F-12K medium; ATCC) supplemented with 10% fetal bovine serum (FBS) (HyClone, Logan, UT, USA) and 0.1% gentamicin (Sigma, St. Louis, MO, USA). Cells were grown overnight (37°C and 5% CO2) to semiconfluence in 75-cm2 cell culture flasks (Corning, Edison, NJ, USA) and harvested by trypsinization. Cells were then washed by centrifugation at 130 × g for 8 min, resuspended in complete medium, and seeded into 96-well plates for viability assays (15,000 cells/well), and into 12-well plates (500,000 cells/well) for gene and protein expression studies. Prior to PM exposures and M. tuberculosis infection, cells were rested overnight at 37°C and 5% CO2.
Sampling of air pollution PM.
The PM2.5 and PM10 samples used in this study were collected in April 2012 on the rooftop (about 15 m above the ground) of the National Environmental Research and Training Center (CENICA) site in Iztapalapa, Mexico City. Iztapalapa is the most populated of 16 municipalities in Mexico City and characterized by dense traffic, unregulated small industrial emissions, and elevated levels of air contamination (20). PM were sampled using continuously operating high-volume PM10 and PM2.5 samplers (GMW model 1200, volumetric flow control high-volume PM10 sampler; Sierra Andersen, Smyrna, GA, USA) with an airflow rate of 1.13 m3/min ± 10%. PM2.5 and PM10 samples were collected on 20.3- by 25.4-cm cellulose nitrate membranes (Sartorius, Goettingen, Germany) with a nominal pore size of 3 μm. Nitrocellulose membranes were removed from the samplers on alternate days, at least twice a week, and PM mechanically recovered from the membranes and pooled by month and particle size (PM2.5 and PM10). Microbiological analysis of the PM samples in the clinical microbiology laboratory at the Robert Wood Johnson University Hospital showed the presence of a variety of bacterial and fungal species, such as Bacillus, Zygomycetes, and Aspergillus species. To permit cell exposure of cultures and eliminate viable bacteria and fungi, PM were sterilized by autoclaving (121°C and 100 kPa for 20 min using a Steris SV-1262). No differences were found in the type and variety of the bacterial and fungal species contained in the PM2.5 and PM10 samples. Preliminary analyses in conjunction with Gedi Mainelis' laboratory at Rutgers University showed that the presence of the bacterial species in the PM samples derived from the sampling of ambient air and was not due to artifactual contamination of the PM in the sampling and filter removal process.
Preparation of PM suspensions.
PM2.5 and PM10 were weighed on a semimicrobalance (CPA225D; Sartorius, Bohemia, NY, USA) and placed into prewashed and baked (4 h at 200°C) glass flasks. Stock suspensions of PM2.5 and PM10 (1 mg/ml) were prepared by 5 min of sonication (model 351OR-DTH; Branson, Danbury, CT, USA) in F-12K medium enriched with 2% FBS and further diluted in complete culture medium to final concentrations of 0.1, 1, 10, and 50 μg/ml.
Preparation of M. tuberculosis for in vitro infections.
Suspensions of M. tuberculosis (avirulent strain H37Ra) were prepared in Middlebrook 7H9 broth medium supplemented with albumin-dextrose-catalase (ADC) (BD Bioscience, Franklin Lakes, NJ, USA) and 0.2% glycerol. After 21 days of incubation at 37°C on an orbital shaker, M. tuberculosis stock suspensions were harvested, aliquoted, and kept frozen at −86°C until use. The M. tuberculosis concentrations used in each of the infection experiments were confirmed from each thawed M. tuberculosis aliquot by CFU assay of serial dilutions on 7H10 solid agar plates after 21-day incubations at 37°C. Single-cell M. tuberculosis suspensions for A549 infection experiments were prepared as follows. Frozen M. tuberculosis stock suspension aliquots were thawed and centrifuged for 5 min at 6,000 × g, and the resulting M. tuberculosis pellets declumped by vortexing (5 min) with five sterile 3-mm glass beads in 1 ml of RMPI 1640 medium enriched with 10% pooled human AB serum (Gemini Bio-Products, West Sacramento, CA, USA) (19). The remaining M. tuberculosis clumps were removed with an additional centrifugation step at 350 × g for 5 min. The M. tuberculosis suspension volumes required to obtain the desired multiplicities of infection (MOI; i.e., the ratio of M. tuberculosis cells per A549 cell [0.1:1, 1:1, and 10:1]) were calculated based on the CFU numbers known to be present in the M. tuberculosis suspension supernatants. The actual CFU numbers used for in vitro infections were confirmed in each experiment.
A549 cell exposure to PM, infection with M. tuberculosis, and CFU assays.
A549 cells were exposed to either PM alone or to PM prior to infection with M. tuberculosis. For exposure to PM alone, after two washing steps with 1× phosphate-buffered saline (PBS), semiconfluent A549 cells in 12-well plates (Corning) were exposed to PM2.5 or PM10 at final concentrations of 0 (unexposed controls), 0.1, 1, 10, and 50 μg/ml in 1 ml of 2% FBS-enriched F-12K medium and incubated (37°C) for 18 h. For exposure to PM prior to infection with M. tuberculosis, semiconfluent A549 cells were exposed to PM2.5 or PM10 (0, 0.1, 1, 10, and 50 μg/ml) (37°C for 18 h), washed twice with 1× PBS, and then, in F-12K medium enriched with 2% FBS, infected with M. tuberculosis at an MOI of 10 (37°C for an additional 18 h). In detail, 2 h after the addition of M. tuberculosis suspension to the A549 cells, cells were washed twice with warm PBS to remove extracellular M. tuberculosis. The A549 monolayers were then incubated for an additional 16 h (37°C and 5% CO2). Assessments of M. tuberculosis growth and, thus, the mycobactericidal capacity of PM-exposed A549 cells were done as described previously (21, 22). Briefly, A549 cells were washed twice with 1× PBS and then lysed with 0.1% SDS (10 min at room temperature) to release any remaining viable intracellular M. tuberculosis cells. The SDS action was neutralized by the addition of Middlebrook 7H9 broth enriched with 20% bovine serum albumin (BSA) to each well. Four serial cell lysate dilutions (1:10) were then prepared and plated in triplicate (10 μl each) onto 7H10 agar plates. The plates were incubated at 37°C for CFU assessments at 21 days using a stereomicroscope at a magnification of ×40 (Fisher Scientific).
Viability assay.
The viability of A549 cells following PM exposure and M. tuberculosis infection was assessed by an MTS [3-(4,5-dimethylthiazol-2-yl)-5-(3-carboxymethoxyphenyl)-2-(4-sulfophenyl)-2H-tetrazolium] proliferation assay using the CellTiter 96 aqueous one solution cell kit (Promega, Madison, WI, USA) according to the manufacturer's protocol. Briefly, A549 cells were plated in triplicate 96-well plate wells per condition, allowed to grow to semiconfluence, washed twice with 1× PBS, and exposed to PM2.5 or PM10 at concentrations of 0, 0.1, 1, 10, 50, and 100 μg/ml in 100 μl of complete culture medium. The viability of the A549 cells was determined at 24, 48, and 72 h following exposure to PM alone or at 36 h following the initial 18-h exposures to PM and subsequent 18-h infection with M. tuberculosis. After 20 μl of CellTiter 96 aqueous one solution reagent (Promega, WI, USA) was added to each well, the plates were incubated (37°C and 5% CO2) for 1 h. The concentrations of formazan (a compound resulting from the bioreduction of tetrazolium, indicating metabolically active cells) were then measured at 490 nm with a Multiskan FC (Fisher Scientific) plate reader. The results are presented as the mean percentages from three independent experiments performed on three different days.
Preparation of mRNA and cDNA.
Total RNA was extracted from PM-exposed and M. tuberculosis-infected A549 cells, as well as unexposed or uninfected A549 cells, using an RNeasy minikit (Qiagen, Germantown, MD), and DNA was removed from the RNA by RNase-free DNase treatment (Qiagen). The concentration and purity of the RNA were determined by using a Nanodrop spectrophotometer (Nanodrop Technologies, Inc.) prior to transcription of 400 ng of total RNA into cDNA using MultiScribe reverse transcriptase (Applied Biosystems, Foster City, CA), according to the manufacturer's protocol.
qRT-PCR.
The fold changes in IL-8, MCP-1, HBD-2, and HBD-3 mRNA expression following exposure to PM2.5 or PM10, M. tuberculosis infection, or PM exposure prior to M. tuberculosis infection were quantified by quantitative real-time PCR (qRT-PCR) using SYBR green dye (Applied Biosystems, Foster City, CA). Oligonucleotide primers for the β-actin, IL-8, MCP-1, HBD-2, and HBD-3 genes (Table 1) were designed using Primer Express (Applied Biosystems) and purchased from Integrated DNA Technologies (Coralville, IA, USA). The PCR mixtures (final volume of 10 μl) contained 2 μl of template and 8 μl of the reaction mixture (SYBR green, water, and primer). Quantitative fluorogenic amplification of cDNA was performed using the ABI ViiA 7 real-time PCR system (Applied Biosystems). A two-step cycling program consisting of 1 cycle at 95°C for 10 min and 40 cycles at 95°C for 15 s, with a final step of 60°C for 1 min, was used. Primer quality was ensured by controlling primer dissociation curves. mRNA fold changes were calculated using the cycle threshold (CT) relative quantitation method (ΔΔCT).
TABLE 1
Sequences of primers used for qRT-PCR
Gene | Target | Forward primer | Reverse primer |
---|---|---|---|
ACTB | Beta-actin | CCA TCA TGA AGT GTG ACG TGG A | TTC TGC ATC CTG TCG GCA A |
DEFB4 | HBD-2 | GGT ATA GGC GAT CCT GTT ACC TGC | TCA TGG CTT TTT GCA GCA TTT TGT TC |
DEFB103A | HBD-3 | GAG CAC TTG CCG ATC TGT TC | CAG AAA TAT TAT TGC AGA GTC AGA GG |
IL-8 | IL-8 | ACA CTG CGC CAA CAC AGA AAT TA | TTT GCT TGA AGT TTC ACT GGC ATC |
CCL2 | MCP-1 | GCT CAT AGC AGC CAC CTT CAT TC | GGA CAC TTG CTG CTG GTG ATT C |
ELISA.
IL-8, MCP-1, and HBD-2 concentrations were assessed by enzyme-linked immunosorbent assay (ELISA) in 96-well plates (Peprotech, Rock Hill, NJ) according to the manufacturer's instructions. Briefly, capture antibodies were diluted with 1× PBS to concentrations of 0.5 μg/ml (HBD-2 and IL-8) or 0.25 μg/ml (MCP-1) and added (100 μl/well) to 96-well plates for overnight incubation. The plates were then washed 4 times with washing buffer (300 μl/well) and incubated with blocking buffer (300 μl/well) for 1 h at room temperature. Following an additional washing step, standards or samples were added (100 μl/well) and the plates incubated for 2 h (IL-8 and MCP-1) or 2.5 h (HBD-2) and washed, detection antibodies were added and the plates incubated for 2 h (0.5 μg/ml, 100 μl/well) and washed again, and avidin peroxidase (100 μl of a 1:2,000 dilution/well) was added and the plates incubated for 30 min at room temperature. After a final washing step, the plates were incubated with ABTS [2,2′-azino-bis(3-ethylbenzothiazoline-6-sulfonic acid)] substrate (100 μl/well, 20 min at room temperature) and read in quadruplicate using a Fisher Scientific Multiskan FC microplate reader (405 nm, with a correction wavelength of 650 nm).
SA-β-Gal staining assay.
Cell senescence was assessed using the senescence-associated β-galactosidase (SA-β-Gal) staining kit (Cell Signaling Technology, MA, USA) according to the manufacturer's instructions. Briefly, semiconfluent A549 cells in 6-well plates in 1.5 ml of F-12K medium enriched with 2% FBS were incubated overnight and subsequently exposed to 0, 0.1, 1, 10, and 50 μg/ml of PM2.5 or PM10 for 18 h. The supernatants were then removed, the A549 cells washed twice with 1× PBS, and 1 ml of fixative solution (20% formaldehyde, 2% glutaraldehyde in PBS) added to each well (15-min incubation at room temperature) prior to two washing steps with 1× PBS. One milliliter of β-galactosidase staining solution (5-bromo-4-chloro-3-indolyl-β-d-galactopyranoside in N-N-dimethylformamide) was then added, and the cells incubated overnight at 37°C in a CO2-free incubator with 1 ml of β-galactosidase staining solution. To assess the proportions of senescent cells, 200 cells per condition were counted under a microscope (×200 magnification) (Axiovert 40C; Carl Zeiss) and the percentage of cells stained in blue (senescent cells) assessed.
TEM of A549 cells.
To assess the cellular uptake and localization of PM and M. tuberculosis cells, A549 cells were prepared for transmission electron microscopy (TEM) as follows. A549 cells (106/well in 6-well plates) were incubated overnight (in complete medium at 37°C and 5% CO2), washed, and subsequently exposed to either PM2.5 or PM10 (10 μg/ml) for 18 h as the only treatment or were then infected with M. tuberculosis at an MOI of 10 for an additional 18 h. The A549 cells were then removed by trypsinization and fixed in 2.5% glutaraldehyde–4% paraformaldehyde in 0.1 M cacodylate (90 min at room temperature). After washing with PBS once, A549 cells were postfixed in buffered 1% osmium tetroxide, dehydrated in a graded series of acetone, and embedded in Epon resin. Fixed cells were cut into 90-nm thin sections using a Leica EM UC6 ultramicrotome. Sectioned grids were then stained with a saturated solution of uranyl acetate and lead citrate. Images were captured with an AMT XR111 digital camera (Advanced Microscopy Techniques, Woburn, MA) on a Philips CM12 transmission electron microscope.
Statistical analysis.
All data are presented as means ± standard deviations (SD). Linear models were used to examine whether fold changes in mRNA expression and protein production were affected by exposure to each of the PM doses within each experimental condition. Fold changes in mRNA expression and protein production were expressed relative to the change from the results for the zero doses within specific exposure conditions, and log-transformed values were used in the linear models. F tests of the effect of PM dose examined whether any of the mRNA and protein levels at higher PM doses were significantly different than at the zero dose by testing whether any of the means of the mRNA log fold changes were significantly different than those at the zero dose. t tests of individual regression coefficients gave results for each specific dose relative to the results for the zero dose. In addition, the results for different MOI were compared with the results for uninfected cells.
RESULTS
Characterization of PM2.5 and PM10.
PM2.5 and PM10 samples were analyzed for soluble ions (by ion chromatography), carbon (by thermal optical analysis with DRI model 2001), elements (by X-ray fluorescence), polycyclic aromatic hydrocarbons (PAHs) (by thermal desorption and gas chromatography–mass spectrometry [GC-MS]), and endotoxin (by limulus amebocyte lysate [LAL assay] using an ELx808IU microplate reader [BioTek Instrument Inc., Winooski, VT, USA]). All analyses were conducted at the Desert Research Institute (Reno, NV). According to the mass construction approach used previously (23), the major components that accounted for 83% of the mass of PM2.5 consisted of 4.5% sulfate, 2.1% nitrate, 30.9% carbon, and 46.2% crustal elements. Similarly, the major components accounting for 75% of the mass of PM10 consisted of 5.6% sulfate, 1.8% nitrate, 29.0% carbon, and 39.4% crustal elements. Both PM2.5 and PM10 contained toxic trace elements (e.g., vanadium, zinc), some of which are reported to have effects on immune responses (24). The concentrations of vanadium (V), chromium (Cr), iron (Fe), copper (Cu), and zinc (Zn) were 91, 52, 37,383, 4,303, and 2,449 ng/mg in PM2.5 and 104, 45, 28,143, 17,053, and 1,667 ng/mg in PM10. Trace levels of endotoxin were detected in PM2.5 and PM10 samples at 14.7 and 0.74 endotoxin units/mg, respectively.
Cytotoxic effects of PM2.5 and PM10.
A549 cells were exposed to PM2.5 or PM10 at a concentration of 0 (no PM, negative control), 0.1, 1, 10, 50, or 100 μg/ml for 24, 48, and 72 h (Fig. 1A), and their viabilities were assessed by MTS assay. Exposures of A549 cells to PM2.5 or PM10 for 24 h did not result in any significant decreases in mitochondrial activity, a measure of cellular viability. However, A549 cell viability decreased significantly upon exposure to PM2.5 at 10, 50, or 100 μg/ml following 48- and 72-h exposures. Interestingly, the viability of A549 cells exposed to PM10 was significantly reduced only after 72 h at 10, 50, or 100 μg/ml (Fig. 1A).
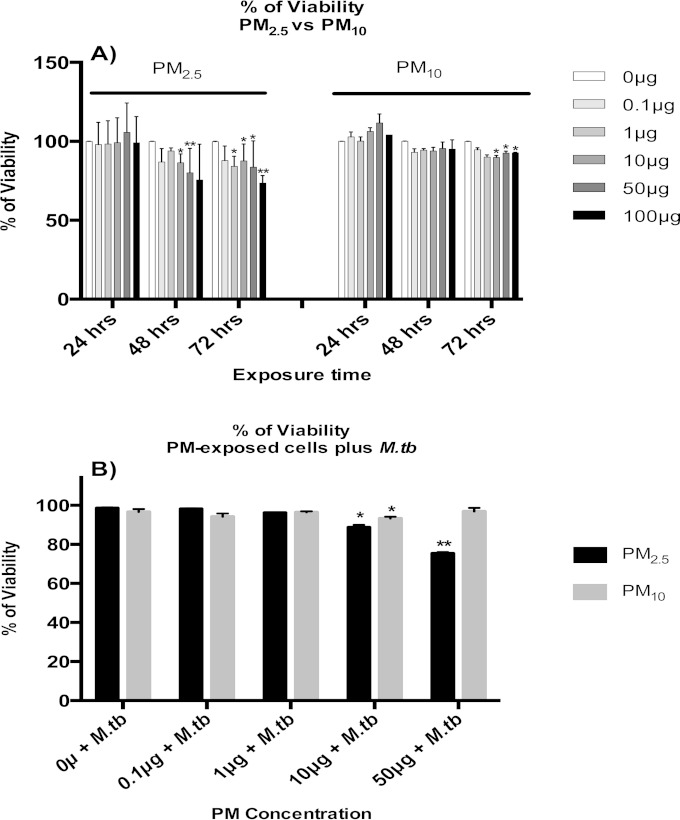
Cytotoxic effects of PM2.5 and PM10 in A549 cells. (A) A549 cells were exposed to 0, 0.1, 1, 10, 50, or 100 μg/ml of PM2.5 or PM10 for 24, 48, and 72 h, and viabilities were determined by MTS assay. (B) Similarly, the effects on A549 cell viability of 18 h of incubation with PM2.5 or PM10 prior to a subsequent M. tuberculosis infection for 18 h were measured by MTS assay. Data represent mean results ± SDs from three independent experiments. Statistically significant differences between results for unexposed and PM-exposed cells are shown with single (P < 0.05) or double (P < 0.01) asterisks.
To assess cytotoxicity in the context of PM exposure and M. tuberculosis infection, A549 cells were exposed to PM2.5 or PM10 at 0, 0.1, 1, 10, or 50 μg/ml for 18 h, followed by M. tuberculosis infection (MOI of 10) for an additional 18 h. A549 cells incubated in complete culture medium for 36 h served as a control. The viabilities of PM- and M. tuberculosis-exposed A549 cells were compared with that of control A549 cells. No statistically significant differences were observed between cells infected with M. tuberculosis alone (MOI of 10; 0 μg/ml PM) and control cells. A549 cells exposed to PM2.5 or PM10 and subsequently infected with M. tuberculosis showed significant decreases in viability at 10 and 50 μg/ml for PM2.5 and at 10 μg/ml for PM10 compared to the viability of M. tuberculosis-infected cells with no PM (P < 0.05). PM2.5 induced greater toxicity than PM10 in cells infected with M. tuberculosis (Fig. 1B).
Effects of PM2.5 and PM10 exposure on antimicrobial peptide and chemokine expression.
To assess the effects of PM exposures on antimicrobial peptide and chemokine production, A549 cells were exposed to PM2.5 or PM10 (0.1, 1, 10, or 50 μg/ml) for 18 h or left unexposed (0 μg/ml). HBD-2, HBD-3, IL-8, and MCP-1 mRNA and protein expression levels were assessed by qRT-PCR and ELISA, respectively. The HBD-2, HBD-3, IL-8, and MCP-1 mRNA expression profiles of PM-exposed A549 cells were compared to that of unexposed control A549 cells (0 μg PM). The HBD-2 mRNA fold changes and protein production in PM2.5-exposed cells followed bell-shaped (quantal) dose-responses (Fig. 2A and andB)B) (18). It is worth noting that the HBD-2 protein expression profile correlated with HBD-2 mRNA fold changes (Fig. 2A and andB).B). In contrast to PM2.5 exposure, PM10 exposure inhibited HBD-2 protein production in a concentration-dependent manner (Fig. 2B). PM10 induced significantly increased HBD-3 mRNA expression at exposure concentrations higher than 1 μg/ml, while exposure to PM2.5 did not result in significant changes compared to the mRNA expression in unexposed control cells (Fig. 2C). HBD-3 protein expression could not be studied as no ELISA was available.
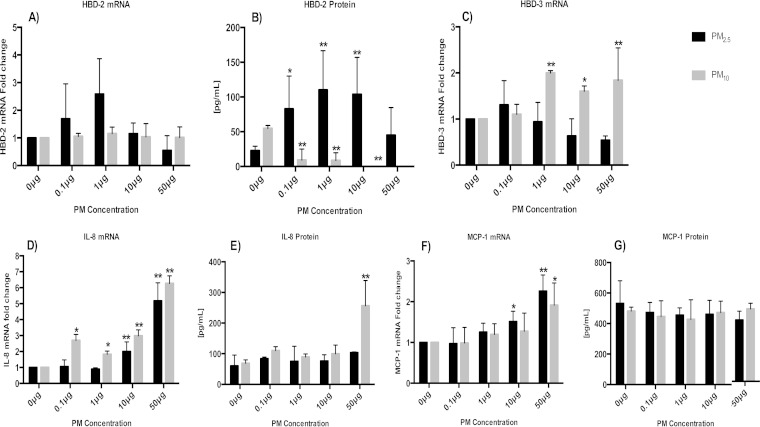
Effects of PM exposure on chemokine and antimicrobial peptide production in A549 cells. A549 cells were incubated with 0, 0.1, 1, 10, or 50 μg/ml of PM2.5 or PM10 for 18 h, and mRNA fold changes and protein production assessed by qRT-PCR and ELISA, respectively. The mRNA fold changes and protein production of HBD-2 (A and B), HBD-3 (C), IL-8 (D and E), and MCP-1 (F and G) are shown. Data represent mean results ± SDs from three independent experiments. Statistically significant differences between results for unexposed and PM-exposed cells are shown with single (P < 0.05) or double (P < 0.01) asterisks.
IL-8 (Fig. 2D and andE)E) mRNA fold changes and protein production were positively correlated with the concentrations of both PM2.5 and PM10 (P < 0.05). However, for MCP-1 (Fig. 2F), this correlation was only observed in mRNA fold changes and not in protein expression. These findings are consistent with other reports (25,–28) showing proinflammatory immune responses in epithelial cells exposed to PM obtained from diverse sources.
Effects of PM2.5 and PM10 exposure on M. tuberculosis-induced antimicrobial peptide and chemokine expression.
To model the effects of air pollution exposure prior to M. tuberculosis infection on HBD-2, HBD-3, IL-8, and MCP-1 production, A549 cells were exposed to PM2.5 and PM10 for 18 h and then infected with M. tuberculosis for an additional 18 h. The mRNA and protein expression levels were assessed by qRT-PCR and by ELISA of culture supernatants, respectively. The levels of HBD-2 and HBD-3 expression in response to M. tuberculosis infection were decreased in PM-exposed A549 cells compared to the levels in cells only infected with M. tuberculosis (Fig. 3A to toC).C). Inhibition of HBD-2 and HBD-3 expression was observed in both PM2.5- and PM10-exposed A549 cells at concentrations as low as 0.1 μg/ml (P < 0.05) (Fig. 3A to toCC).
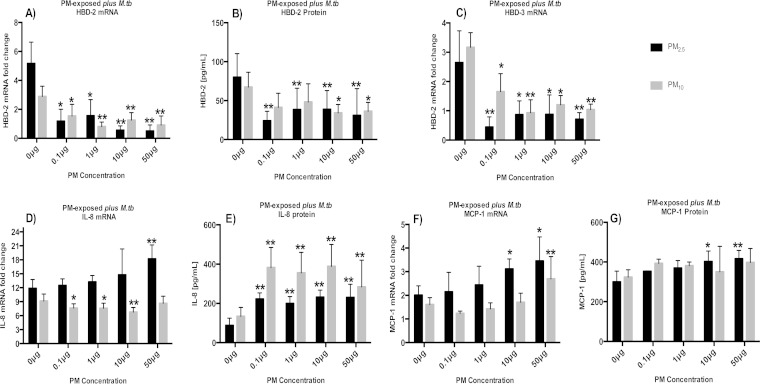
Effects of PM exposure on M. tuberculosis-induced chemokine and antimicrobial peptide production in A549 cells. The mRNA fold changes and protein production of A549 cells incubated with PM2.5 and PM10 at 0, 0.1, 1, 10, or 50 μg/ml for 18 h followed by 18 h of M. tuberculosis infection (MOI 10) were determined by qRT-PCR and ELISA, respectively. Graphs represent mRNA fold changes and protein production of HBD-2 (A and B), HBD-3 (C), IL-8 (D and E), and MCP-1 (F and G). Data represent mean results ± SDs from three independent experiments. Statistically significant differences between results for unexposed and PM-exposed cells are shown with single (P < 0.05) or double (P < 0.01) asterisks.
The IL-8 mRNA expression patterns were different in PM2.5- and PM10-exposed M. tuberculosis-infected A549 cells. While PM2.5 induced IL-8 mRNA expression, PM10 inhibited IL-8 mRNA expression in M. tuberculosis-infected A549 cells. Interestingly, this opposing effect seen in PM2.5- and PM10-exposed cells was not observed when culture supernatants were assessed for the corresponding protein expression. Both PM2.5 andPM10 exposures induced significantly higher levels of IL-8 protein (P < 0.01) than M. tuberculosis infection alone. The discrepancies between IL-8 protein and mRNA expression levels may be due to the short half-life of IL-8 mRNA (29) (Fig. 3D and andE).E). The observed reduction of IL-8 mRNA in PM10-exposed cells (Fig. 3D) may be due to the induction of cellular senescence discussed below (see Fig. 6). In response to M. tuberculosis infection, both the mRNA and protein level corresponding to MCP-1 increased significantly (P < 0.05) in A549 cells exposed to PM2.5 at concentrations of 10 and 50 μg/ml. On the other hand, a significant increase in MCP-1 mRNA expression was observed in A549 cells exposed to PM10 at 50 μg/ml (Fig. 3F and andGG).
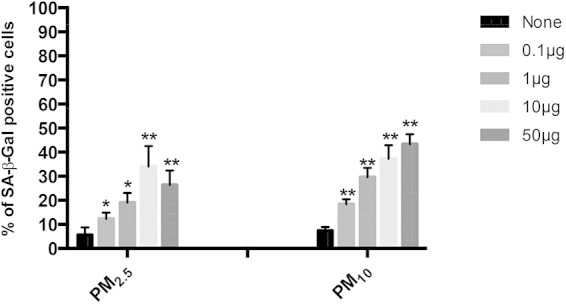
PM induces senescence in A549 cells in a dose-dependent manner. Percentages of senescent cells were determined by SA-β-Gal staining. The proportions of SA-β-Gal-positive cells increased in a dose-dependent manner when A549 cell were exposed to 0, 0.1, 1, 10, or 50 μg/ml of PM2.5 or PM10 for 18 h. The proportions of senescent cells were higher in PM10- than in PM2.5-exposed cells. Mean results ± SD from three independent experiments are shown. Statistically significant differences between results for unexposed and PM-exposed cells are shown with single (P < 0.05) or double (P < 0.01) asterisks.
Effects of PM2.5 and PM10 on M. tuberculosis growth control in A549 cells.
HBD-2 and HBD-3, which are produced by A549 cells in response to M. tuberculosis infection, contribute to A549-mediated M. tuberculosis growth control. To examine whether the observed suppression of HBD-2 and HBD-3 production in PM-exposed A549 cells (Fig. 3A to toC)C) alters the intracellular growth control of M. tuberculosis, A549 cells were exposed to PM2.5 or PM10 (0.1, 1, 10, or 50 μg/ml) or left unexposed, followed by M. tuberculosis infection (MOI of 0.1, 1, and 10), and CFU assays were performed. To ensure that the growth control assay results were not affected by extracellular M. tuberculosis remaining on A549 cells prior to cell lysis and plating of the cell lysates on agar plates, multiple washing steps were performed and the wash fluids assessed for CFU content. No M. tuberculosis (CFU) was found in the wash fluids of the last three of the total of six washing steps done for each sample prior to A549 cell lysis (data not shown). The CFU counts assessed in our experiments (Fig. 4A and andB)B) thus represent M. tuberculosis growth/survival in the intracellular environment of A549 cells and are not falsely increased by extracellular bacteria left over from the experimental infection process. The M. tuberculosis CFU numbers were significantly higher in PM2.5-exposed than in unexposed (0 μg/ml) M. tuberculosis-infected (MOI of 10) cells (Fig. 4A) at all PM concentrations (P < 0.05), indicating suppression of intracellular M. tuberculosis growth control upon PM2.5 exposure. Furthermore, in cells exposed to 10 or 50 μg/ml PM2.5 prior to M. tuberculosis infection, impairment of growth control was observed even at MOI as low as 1 and 0.1. In PM10-exposed A549 cells (Fig. 4B), a PM dose-dependent decrease in M. tuberculosis growth control was observed in cells infected with an MOI of 0.1 but not in cells infected with higher doses of M. tuberculosis (MOI of 1 and 10). Although at present we cannot explain the latter observation, it could be related to reduced M. tuberculosis uptake by the cells and/or induction of cellular senescence (discussed below). Interestingly, M. tuberculosis CFU numbers were lower in A549 cells exposed to 10 or 50 μg/ml PM10 (Fig. 4B) than in A 549 cells exposed to the same concentrations of PM2.5 (Fig. 4A). Taken together, these data suggest that the differential effects of PM on M. tuberculosis growth control in A549 cells were probably related to differences in the physicochemical characteristics of the two PM fractions. As expected, the observed reduction of A549-mediated M. tuberculosis growth control appeared to be correlated with the downregulation of HBD-2 and HBD-3 expression upon exposure to PM (Fig. 3A to toC),C), confirming the importance of the antimicrobial peptide function for M. tuberculosis growth control by A549 cells.
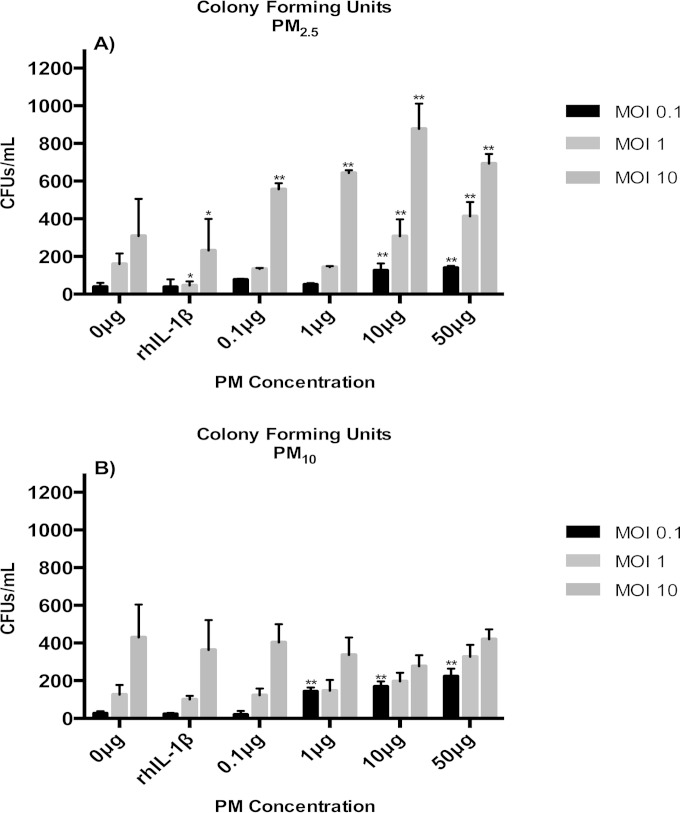
PM exposure decreases intracellular mycobacterial growth control. A549 cells were incubated with 0, 0.1, 1, 10, or 50 μg/ml of PM2.5 (A) or PM10 (B) for 18 h, followed by infection with M. tuberculosis at an MOI of 0.1, 1, or 10 for an additional 18 h. Recombinant human interleukin-1β (rhIL-1β) was used as a positive control for HBD-2 induction. CFU assays were performed to determine the effect of PM on the mycobacterial growth control of A549 cells. CFU numbers are shown as mean results ± SDs from three independent experiments. Statistically significant differences between results for unexposed and PM-exposed cells are shown with single (P < 0.05) or double (P < 0.01) asterisks.
Effects of PM2.5 and PM10 on A549 cell morphology.
To assess whether the exposure to PM and infection with M. tuberculosis induce morphological or ultrastructural changes and alter bacterial uptake in the A549 cells, TEM was performed (Fig. 5). We observed uptake of PM2.5 and PM10 in exposed A549 cells. PM2.5 and PM10 varied by size, density, and shape (Fig. 5F and andI,I, dashed arrows). PM2.5 presents in an irregular structure, while PM10 maintains a well-defined spherical structure that is attributable to PM from industrial sources (30). Interestingly, while M. tuberculosis uptake appeared to lead to endocytic vesicle formation (with a double membrane surrounding the bacteria), no vesicle formation was noted surrounding PM2.5 or PM10 (Fig. 5E, ,F,F, ,H,H, and andI),I), probably indicating differences in the mechanisms underlying cellular uptake of M. tuberculosis and PM. The M. tuberculosis uptake appeared to be greater in cells exposed to PM2.5 than in cells exposed to PM10 (data not shown), a finding that correlated with our CFU data (Fig. 4), indicating that cellular M. tuberculosis uptake may have been related to the size of the PM. In addition, A549 cells revealed major morphological abnormalities upon PM exposure, particularly following PM10 exposure. The abnormalities in cell morphology appeared to be microvillus shedding and elongation and swelling of mitochondria (Fig. 5J and andK),K), which have been described to be indicative of cellular senescence (31,–33). These abnormalities were not observed in PM-unexposed control A549 cells (Fig. 5L) or A549 cells only infected with M. tuberculosis (Fig. 5A), suggesting that the ultrastructural changes observed here were due to the PM exposure.
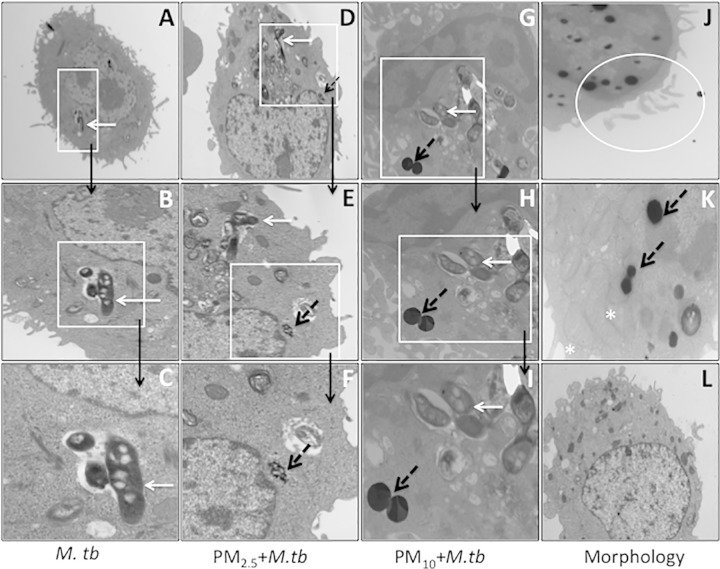
Transmission electron microscopy (TEM) of PM and M. tuberculosis uptake in A549 cells. (A) Overview (×3,800) of uptake of multiple M. tuberculosis cells (white arrow) by an A549 cell. (B and C) Magnifications (×10,000 [B] and ×17,000 [C]) of boxed area from panel A showing endocytic vacuoles containing M. tuberculosis in an infected A549 cell. (D) Overview (×3,800) of a PM2.5-exposed A549 cell (18-h exposure to 10 μg/ml PM followed by M. tuberculosis infection at an MOI of 10 for an additional 18 h). (E) Magnification (×10,000) of boxed area from panel D showing an endocytic vacuole containing M. tuberculosis cells (white arrow) and PM2.5 (black dashed arrow). (F) Magnification (×17,000) of boxed area from panel E showing PM2.5 within the cell. (G) Overview (×3,800) of a PM10-exposed (10 μg/ml for 18 h), M. tuberculosis-infected (MOI of 10) A549 cell showing cytoplasmic PM10 (black dashed arrow) and M. tuberculosis cells (white arrow) in endocytic vacuoles. (H) Magnification (×10,000) of boxed area from panel G showing PM10 (black dashed arrow) and multiple M. tuberculosis cells (white arrow). (I) Magnification (×17,000) of boxed area from panel H showing PM10 (black dashed arrow) and multiple M. tuberculosis cells (white arrow). Multiple M. tuberculosis cells are visible in endocytic vacuoles surrounded by double membranes, while PM10 (black dashed arrow) appears to be directly inserted in the cytoplasm, with endocytic vacuole formation completely missing. (J) Magnification (×10,000) of a PM10-exposed A549 cell showing ultrastructural changes that appear to represent microvillus shedding (circled). (K) Magnification (×22,000) of an A549 cell exposed to PM10 (black dotted arrow) showing mitochondrial elongation and swelling (white asterisks). (L) Magnification (×3,800) of a PM-unexposed and M. tuberculosis-uninfected A549 cell.
Exposure to PM induces senescence in A549 cells.
Air pollution PM has been described to induce senescence in A549 cells (34). We therefore sought to examine whether the induction of senescence by PM2.5 and PM10 may contribute to PM-induced downregulation of HBD-2 and HBD-3 and the decreased M. tuberculosis growth control observed in A549 cells. A549 cells exposed to 0, 0.1, 1, 10, or 50 μg/ml of PM2.5 or PM10 for 18 h were examined for SA-β-Gal activity, a well-established biomarker of senescence. A significant PM dose-dependent induction of senescence (P < 0.05) was observed in PM2.5- and PM10-exposed A549 cells. Furthermore, A549 cells became senescent when exposed to PM2.5 (P < 0.05) or PM10 (P < 0.01) doses as low as 0.1 μg/ml (Fig. 6). Interestingly, PM10 induced a greater proportion of senescent cells than PM2.5. This observation is consistent with our TEM findings showing a broader range and intensity of morphological abnormalities in PM10-exposed A549 cells than in PM2.5-exposed A549 cells.
DISCUSSION
Recent epidemiologic evidence suggests that the risk of developing TB increases with exposure to air pollution (5, 6, 8,–10, 35). To date, only a few studies have explored the immunological and molecular mechanisms and effects of ambient PM on human antimycobacterial immunity (11, 19, 36, 37). Unraveling the links between ambient air pollution exposure and alterations in human antimycobacterial immunity is crucial, as air pollution from growing vehicular traffic and industrial production is increasing in rapidly urbanizing regions of the world where TB is endemic.
In the current study, we examined the effects of PM2.5 and PM10 from Mexico City on M. tuberculosis-induced innate immune responses of A549 cells that represent human alveolar type II pneumocytes, which in vivo interact with both aerosolized PM and M. tuberculosis shortly after their inhalation uptake (15, 17, 38,–40). The PM concentrations used in our experiments reflect the concentrations in the human airways during inhalational real-world urban air pollution exposures. The PM concentrations in the air of Mexico City reach levels of 120 μg/m3. Considering the average air volumes inhaled daily by a person (41), the deposition rate of PM in the lungs (42), and the volume of the pulmonary epithelial lining fluid (43), we calculated that an average person in Mexico City inhales around 80 μg of PM per ml of lining fluid per day. The PM concentrations used in our experiments were 0.1, 1, 10, and 50 μg/ml (or, calculated by area, 0.026, 0.26, 2.6, and 13.15 μg/cm2). In another report assessing the in vitro effects of PM on cytokine production and cell toxicity in epithelial, endothelial, fibroblastic, and monocytic cells, optimal acute effects were reached at concentrations of 40 μg/cm2 (44). The PM concentrations observed in urban settings thus appear to be actually higher than the concentrations reached in our in vitro experiments, indicating that the observed PM effects on antimycobacterial immunity in our in vitro experiments were not due to exaggerated, unrealistic experimental conditions.
The effects of PM exposure on the production of antimicrobial peptides by epithelial cells have neither been studied systematically (24, 45) nor assessed in the context of exposure to PM of different compositions and sizes and M. tuberculosis infection. In this study, an increase in the production of HBD-2 protein was observed in A549 cells exposed to PM2.5 (0.1, 1, and 10 μg/ml) (Fig. 2). This observation differs from a report by Klein-Patel et al. of inhibition of HBD-2 expression in A549 cells following exposure to residual oil fly ash (ROFA), a component of urban PM2.5 (24). It is possible that differences in the content of microbial components (e.g., endotoxin) explain the observed differences between our study and that of Klein-Patel (24). Interestingly, as shown here, PM10, which contained much less endotoxin than PM2.5, inhibited HBD-2 production in all concentrations used (Fig. 2), suggesting that the size and composition of PM components influence biological responses in human cells (46).
We observed a dose-dependent induction of proinflammatory MCP-1 and IL-8 production in both PM2.5- and PM10-exposed A549 cells. These observations are consistent with reports showing the induction of proinflammatory MCP-1 and IL-8 in BEAS-2B cells exposed to PM2.5 and PM10 from different geographical areas (27, 47). Previous studies have linked proinflammatory effects caused by PM2.5 to oxidative stress (28) or to interactions with TLR-3, which is stimulated by polycyclic aromatic hydrocarbons (PAHs), components of cigarette smoke, and air pollution PM (48,–50).
To model the effects of air pollution exposure on innate immune responses to M. tuberculosis by the human respiratory epithelium, we assessed the production of antimicrobial peptides and chemokines in A549 cells exposed to PM and subsequently infected with M. tuberculosis. PM2.5 and PM10 exposure inhibited the production of HBD-2 and HBD-3 in response to M. tuberculosis infection, even at low concentrations (0.1 μg/ml). The inhibition of HBD-2 production in A549 cells is attributed to the presence of vanadium in the study of Klein-Patel et al. with residual oil fly ash (24). The analysis of the PM samples used in the current study revealed the presence of vanadium in PM2.5 and PM10 (91 and 104 ng/mg PM mass, respectively). It is therefore possible that vanadium contributed to the inhibition of HBD-2 production in response to M. tuberculosis. Interestingly, exposure of gingival epithelial and normal human bronchial epithelial cells to cigarette smoke, which also contains vanadium, similarly reduces HBD-2 mRNA expression and peptide production in vitro in response to LPS from Pseudomonas aeruginosa (51). It has been proposed that the downregulation of HBD-2 in P. aeruginosa-infected cells by cigarette smoke may be due to the downregulation of fatty acid-binding protein 5 (FABP5). FABP5 exerts immunomodulatory functions, such as the induction of HBD-2, in primary human airway epithelial cells (52). It was beyond the scope of the current study to determine whether the PM-mediated downregulation of HBD-2 was related to downregulation of FABP5.
We also assessed the proinflammatory effects in A549 cells exposed to PM and infected with M. tuberculosis. Exposure to PM10 resulted in a greater induction of IL-8 protein production than exposure to PM2.5 at all concentrations used. At the lowest concentration of PM2.5 and PM10 (0.1 μg/ml), the production of IL-8 in response to PM and M. tuberculosis was additive and significantly greater (P < 0.05) than that in PM-unexposed but M. tuberculosis-infected cells (Fig. 3D). We also observed significant additive increases of IL-6 mRNA expression in PM2.5- and PM10-exposed, M. tuberculosis-infected cells by qRT-PCR (data not shown), providing further evidence of proinflammatory effects of PM. Published studies have shown similar additive effects in cigarette smoke-exposed primary normal human bronchial epithelial cells infected with P. aeruginosa (52) and in PM-exposed human epithelial-2 cells infected with respiratory syncytial virus (53). Our data also revealed additive effects of PM2.5 exposure and M. tuberculosis infection on MCP-1 expression (Fig. 3G).
Studies in murine models have demonstrated a correlation between deficiencies in HBD-2 and HBD-3 production and significantly increased risk of TB development (17, 18). Based on these antecedents and the suppressive effect of PM exposure on HBD-2 and HBD-3 mRNA expression in M. tuberculosis-infected A549 cells observed here, we assessed the effects of PM2.5 and PM10 on growth control of M. tuberculosis in A549 cells. Indeed, PM2.5 exposure significantly reduced M. tuberculosis growth control (shown by increased CFU numbers) by A549 cells at all PM concentrations at an MOI of 10 and at 10 and 50 μg/ml PM at MOI of 0.1 and 1. In PM10-exposed cells, intracellular M. tuberculosis growth was enhanced only at a low dose of mycobacteria (MOI of 0.1), suggesting that exposure to larger PM may affect M. tuberculosis uptake. The increase of intracellular bacillary loads (reduced bacterial clearance at all MOIs for PM2.5 and at an MOI of 0.1 for PM10) correlated with the PM exposure-induced inhibition of the production of HBD-2 and HBD-3. Both HBD-2 and HBD-3 have potent antimycobacterial (54) and bacteriostatic (55) effects. A previous study of human airway epithelial cells infected with P. aeruginosa showed a similar low bacterial clearance upon epithelial cell exposure to coal fly ash particles (45).
TEM revealed morphological abnormalities, such as microvillus shedding, mitochondrial deformities, and vacuolelike vesicles, characteristics related to senescence in PM10- and PM2.5-exposed A549 cells (32, 56). Senescence is a cellular stage characterized by loss of proliferative capacity without impairment of cell viability and metabolic activity. Earlier studies have linked air pollutant exposures (PM2.5, PM10, and cigarette smoke) to the induction of senescence in epithelial cells via telomeric shortening (57) and oxidative stress (32, 34) inducing irreversible cell growth arrest (31). Our findings demonstrate that cells exposed for 18 h to PM2.5 or PM10 produce higher levels of SA-β-Gal than PM-unexposed control cells and that the number of senescent cells increases in a PM dose-dependent manner (Fig. 6). These observations coincide with earlier findings from our group of the induction of senescence in A549 cells exposed to 10 μg/cm2 of PM10 obtained from the same sampling area in Mexico City (34).
In summary, we propose that the modification of cellular responses directed against M. tuberculosis (such as the observed suppression of HBD-2 and HBD-3 production that correlated with loss of M. tuberculosis growth control in vitro) is due to PM-induced cellular senescence rather than loss of cell viability. Our in vitro findings are relevant for the understanding of the consequences of real-world in vivo air pollutant exposures on antibacterial host immunity. The inhibition of HBD-2 and HBD-3 by air pollution PM exposure in vivo may adversely affect the establishment of efficient antimycobacterial host immune responses in the respiratory tract. Abrogation of the immunoregulatory effects mediated by HBD-2 and HBD-3 adversely affects chemoattraction of immature dendritic cells and T lymphocytes (16, 58), maturation of antigen-presenting cells through TLR-mediated triggering of adaptive Th1 immune responses (59), and induction of IFN-γ production by dendritic cells (60).
Although in vitro findings are important, further studies in vivo will be needed to provide a better understanding of the adverse health effects of PM and its interactions with epithelial cells in the context of innate and adaptive antimycobacterial immunity. For example, decreases of HBD-2 and HBD-3 expression in an in vivo model could affect host immunity to M. tuberculosis not only by directly affecting M. tuberculosis growth control but also by impairing antimicrobial peptide-induced immunomodulatory effects, such as cellular activation and chemotaxis (58, 59). Nonetheless, as shown here, PM exposure increased the expression of IL-8 and MCP-1 in response to M. tuberculosis in PM-exposed A549 cells (Fig. 3E and andG),G), indicating that the effects of reduced HBD-2 and HBD-3 expression could be compensated by, for example, increased recruitment of neutrophils and monocytes via increased IL-8 and MCP-1 expression in vivo.
In conclusion, PM exposure of A549 cells induces cellular senescence, a likely cause of the observed downregulation of HBD-2 and HBD-3 and the subsequent loss of M. tuberculosis growth control. Air pollution may modify protective antimicrobial peptide-induced early innate immunomodulatory effects in the human respiratory epithelium, thus contributing to increased susceptibility to primary aerogenic M. tuberculosis infection and rates of TB.
ACKNOWLEDGMENTS
This work was supported by NIEHS grant R01ES020382-02 (S. Schwander) and Rutgers Center for Environmental Exposures and Disease NIEHS grant number P30ES005022.
We thank Gediminas Mainelis, Department of Environmental Sciences at Rutgers School of Environmental and Biological Sciences, for contributing to the analysis of the PM material.
C. E. Rivas-Santiago, S. Sarkar, Á. Osornio-Vargas, R. Quintana-Belmares, T. J. Kirn, and S. Schwander participated in the conception and design of the study. C. E. Rivas-Santiago, S. Sarkar, Á. Osornio-Vargas, Q. Meng, P. Ohman Strickland, J. C. Chow, J. G. Watson, M. Torres, and S. Schwander participated in analysis and interpretation. Drafting of the manuscript for important intellectual content was by C. E. Rivas-Santiago, S. Sarkar, Á. Osornio-Vargas, Q. Meng, M. Torres, and S. Schwander.
REFERENCES
Articles from Infection and Immunity are provided here courtesy of American Society for Microbiology (ASM)
Full text links
Read article at publisher's site: https://doi.org/10.1128/iai.03018-14
Read article for free, from open access legal sources, via Unpaywall:
https://iai.asm.org/content/iai/83/6/2507.full.pdf
Citations & impact
Impact metrics
Article citations
The effects of meteorological factors and air pollutants on the incidence of tuberculosis in people living with HIV/AIDS in subtropical Guangxi, China.
BMC Public Health, 24(1):1333, 17 May 2024
Cited by: 1 article | PMID: 38760740
PM2.5 Causes Increased Bacterial Invasion by Affecting HBD1 Expression in the Lung.
J Immunol Res, 2024:6622950, 27 Jan 2024
Cited by: 1 article | PMID: 38314088 | PMCID: PMC10838202
Household air pollution and risk of pulmonary tuberculosis in HIV-Infected adults.
Environ Health, 23(1):6, 17 Jan 2024
Cited by: 0 articles | PMID: 38233832 | PMCID: PMC10792790
Air Pollution Drives Macrophage Senescence through a Phagolysosome-15-Lipoxygenase Pathway.
Immunohorizons, 8(4):307-316, 01 Apr 2024
Cited by: 0 articles | PMID: 38625119 | PMCID: PMC11066713
Population impact of fine particulate matter on tuberculosis risk in China: a causal inference.
BMC Public Health, 23(1):2285, 18 Nov 2023
Cited by: 3 articles | PMID: 37980514 | PMCID: PMC10657490
Go to all (53) article citations
Similar Articles
To arrive at the top five similar articles we use a word-weighted algorithm to compare words from the Title and Abstract of each citation.
Season and size of urban particulate matter differentially affect cytotoxicity and human immune responses to Mycobacterium tuberculosis.
PLoS One, 14(7):e0219122, 11 Jul 2019
Cited by: 19 articles | PMID: 31295271 | PMCID: PMC6622489
Long-term exposure to particulate matter from air pollution alters airway β-defensin-3 and -4 and cathelicidin host defense peptides production in a murine model.
Peptides, 142:170581, 28 May 2021
Cited by: 3 articles | PMID: 34052349
Multicity study of air pollution and mortality in Latin America (the ESCALA study).
Res Rep Health Eff Inst, (171):5-86, 01 Oct 2012
Cited by: 48 articles | PMID: 23311234
Are current Chinese national ambient air quality standards on 24-hour averages for particulate matter sufficient to protect public health?
J Environ Sci (China), 71:67-75, 11 Feb 2018
Cited by: 7 articles | PMID: 30195691
Review
Funding
Funders who supported this work.
NIEHS NIH HHS (4)
Grant ID: P30 ES005022
Grant ID: R01ES020382-02
Grant ID: P30ES005022
Grant ID: R01 ES020382