Abstract
Free full text

Role and regulation of coordinately expressed de novo purine biosynthetic enzymes PPAT and PAICS in lung cancer
Abstract
Cancer cells exhibit altered metabolism including aerobic glycolysis that channels several glycolytic intermediates into de novo purine biosynthetic pathway. We discovered increased expression of phosphoribosyl amidotransferase (PPAT) and phosphoribosylaminoimidazole carboxylase, phosphoribosylaminoimidazole succinocarboxamide synthetase (PAICS) enzymes of de novo purine biosynthetic pathway in lung adenocarcinomas. Transcript analyses from next-generation RNA sequencing and gene expression profiling studies suggested that PPAT and PAICS can serve as prognostic biomarkers for aggressive lung adenocarcinoma. Immunohistochemical analysis of PAICS performed on tissue microarrays showed increased expression with disease progression and was significantly associated with poor prognosis. Through gene knockdown and over-expression studies we demonstrate that altering PPAT and PAICS expression modulates pyruvate kinase activity, cell proliferation and invasion. Furthermore we identified genomic amplification and aneuploidy of the divergently transcribed PPAT-PAICS genomic region in a subset of lung cancers. We also present evidence for regulation of both PPAT and PAICS and pyruvate kinase activity by L-glutamine, a co-substrate for PPAT. A glutamine antagonist, 6-Diazo-5-oxo-L-norleucine (DON) blocked glutamine mediated induction of PPAT and PAICS as well as reduced pyruvate kinase activity. In summary, this study reveals the regulatory mechanisms by which purine biosynthetic pathway enzymes PPAT and PAICS, and pyruvate kinase activity is increased and exposes an existing metabolic vulnerability in lung cancer cells that can be explored for pharmacological intervention.
INTRODUCTION
Lung cancer is the leading cause of cancer-related deaths globally [1] with non-small cell lung cancer (NSCLC) accounting for 80% of all lung cancers [2, 3]. In the United States, the overall 5-year survival rate of lung cancer patients is 16% and more than 50% of the cases are diagnosed at advanced stages where curative treatment is not possible. Genomic and gene expression-based characterizations have resulted in improved molecular subtyping of lung cancer and identification of driver mutations including epidermal growth factor receptor (EGFR-10–30%), Kirsten rat sarcoma viral oncogene homolog (KRAS-15–30%), and fibroblast growth factor receptor 1 (FGFR1-20%), among others [4, 5]. Identification of EGFR activating mutations in its kinase domain led to the development of EGFR tyrosine kinase inhibitors, erlotinib and gefitinib [5, 6]. Lung cancer patients harboring ALK gene fusions treated with crizotinib show similar positive response [7, 8]. In recent studies, identification of fusions of CD74-NRG1 that activate HER2:HER3 signaling offered therapeutic possibilities for intractable mucinous lung cancers [9, 10]. Thus, well-defined molecular stratification has become essential for the development of targeted treatment for specific molecular subtypes of cancers and personalized therapy.
Recent studies have identified various metabolic genes and associated metabolites that play a role in oncogenesis. For example, IDH1 and IDH2 mutations confer altered enzyme properties producing D-2-hydroxyglutarate and triggering oncogenesis [11, 12]. Our earlier studies revealed that the metabolite sarcosine and its biosynthetic enzyme, glycine N-methyltransferase (GNMT) were elevated in prostate cancer, while sarcosine dehydrogenase (SARDH) and pipecolic acid oxidase (PIPOX) that metabolize sarcosine, were reduced in prostate tumors [13, 14]. In addition, KRAS-mediated metabolic transformation is known to activate the phosphate pentose pathway and hexosamine biosynthesis in pancreatic cancer [15]. Various oncogenes such as MYC have been shown to induce purine and pyrimidine biosynthesis genes including phosphoribosyl amidotransferase (PPAT), phosphoribosylaminoimidazole carboxylase, phosphoribosylaminoimidazole succinocarboxamide synthetase (PAICS), adenylosuccinate Lyase (ADSL), dihydroorotate dehydrogenase (DHODH) and others [16, 17]. In addition, a yeast screen identified the purine biosynthetic gene PAICS as an anti-apoptotic oncogene [18]. Aerobic glycolysis, also known as the Warburg effect, is a well-known metabolic aberration occurring during oncogenesis [19, 20] that allows cancer cells to siphon glycolytic intermediates into anabolic pathways such as purine and pyrimidine biosynthesis to sustain increased cell proliferation [21]. These studies have advanced our understanding of driver genes and their effect on metabolic pathways involved in cancer and underscore the importance of metabolic dysregulation in cancer progression [22].
Purine nucleotides are synthesized through two distinct pathways, either de novo utilizing simple precursors like amino acids (glutamine, glycine and aspartate) and bicarbonate or through salvage of purine bases released by the hydrolytic degradation of nucleic acids and nucleotides. De novo purine biosynthesis requires 5-phosphoribosyl-1-pyrophosphate (PRPP) as a precursor and glutamine as co-substrate [23]. The initial committed step in this pathway is catalyzed by PPAT leading to production of 5-phosphoribosyl-1-amine (5-PRA). PPAT is divergently transcribed from a locus that also encodes PAICS, another enzyme in the pathway that produces the intermediary metabolite N-succinyl-5-aminoimidazole-4-carboxamide-1-ribose-5′-phosphate (SAICAR), known to activate pyruvate kinase isoform PKM2 under glucose-depleted condition [24]. After the initial committed step, in a series of enzymatic reactions, 5-PRA is converted to inosine monophosphate (IMP), the precursor for nucleotides adenosine monophosphate (AMP) and guanosine monophosphate (GMP).
In this study, we demonstrate that de novo purine biosynthetic pathway enzymes PPAT and PAICS show increased expression in lung cancer and can serve as prognostic markers for patient survival. Further, our investigations indicate PPAT and PAICS genes are necessary for lung tumorigenesis. We show that PPAT and PAICS expression influences the pyruvate kinase activity. Finally, our results reveal glutamine mediated modulation govern PPAT and PAICS over-expression.
RESULTS
De novo purine biosynthetic pathway genes are overexpressed in lung cancers
The de novo purine biosynthetic pathway involves multiple enzymatic steps that convert 5-phosphoribosyl-1-pyrophosphate (PRPP) to inosine monophosphate (IMP), a precursor for adenosine monophosphate (AMP) and guanosine monophosphate (GMP) production. Many of the bifunctional enzymes catalyze more than one step in the pathway. Recent studies have suggested that N-succinyl-5-aminoimidazole-4-carboxamide-1-ribose-5′-phosphate (SAICAR), a product of the enzyme PAICS, is involved in activating pyruvate kinase PKM2 under glucose-depleting condition [24]. PKM2 is involved in increased aerobic glycolysis in cancer [25], and plays a non-metabolic role in cancer by modifying histone H3 [26].
Our analysis using lung cancer gene expression profiling studies [27–33] available in the Oncomine [Oncomine™ Platform (Life Technologies, Ann Arbor, MI) was used for analysis and visualization] [34], showed significantly increased expression of de novo purine biosynthetic enzymes phosphoribosyl pyrophosphate amidotransferase (PPAT), phosphoribosylaminoimidazole carboxylase, phosphoribosylaminoimidazole succinocarboxamide synthetase (PAICS) (Fig. (Fig.1A;1A; Supplementary Fig. S1A). The pyruvate kinase isoform PKM2 expression also showed increased expression in lung cancer. PPAT is involved in conversion of phosphoribosyl pyrophosphate (PRPP) to 5-phosphoribosylamine (5-PRA), a committing step in de novo purine synthesis. We further confirmed the increased expression of divergently transcribed PPAT, PAICS as well as PKM2 expression in lung cancer samples compared to normal samples by next-generation RNA sequencing (Fig. (Fig.1B)1B) and quantitative RT-PCR analysis (Fig. (Fig.1C).1C). We found no difference in PKM1 transcript expression (Fig. (Fig.1C).1C). In addition, Affymetrix microarray analyses showed enhanced expression of PPAT, PAICS and PKM2 in poorly differentiated and stage 3 lung cancers (Supplementary Fig. S2A and S2B, respectively). Furthermore, our analysis showed that PPAT and PAICS are highly expressed in the solid type of adenocarcinoma (Supplementary Fig. S2C) [35]. Analyses of other genes in de novo purine biosynthetic pathway enzymes (purinosome) by oncomine (Supplementary Fig. S1A) [33], gene expression from Seo et al [36] and TCGA datasets [37, 38] (Supplementary Fig. S1B), and next-generation RNA sequencing (Supplementary Fig. S1C) showed increased expression of glycinamide ribonucleotide formyltransferase (a trifunctional enzyme with GARS, AIRS, GART activity) (GART), 5-aminoimidazole-4-carboxamide ribonucleotide formyltransferase/IMP cyclohydrolase (ATIC) and phosphoribosylformylglycinamidine synthase (PFAS), but not adenylosuccinate lyase (ADSL) levels (Supplementary Fig. S1C) [39]. Consistent with RNA expression, immunoblot analyses of PPAT, PAICS and PKM2 showed increased expression in cancer tissues compared to normal lung (Fig. (Fig.1D).1D). However, PKM1 protein did not show increased expression in cancer similar to the transcript data (Fig. (Fig.1D1D).

A. Gene expression profiling [28, 30, 32, 33, 73–75] studies suggest enhanced expression of de novo purine biosynthetic enzymes PPAT & PAICS and PKM2 in multiple lung adenocarcinoma tissues. Expression is represented by a color scale highlighting down-regulation (blue), no alteration (white), and up-regulation (red) of transcripts. B. Quantitative measurement of PPAT, PAICS and PKM2 by Next-Generation RNA sequencing [RPKM (log2)] in lung adenocarcinomas [39]. C. Quantitative real-time polymerase chain reaction (qRT-PCR) measurement of PPAT, PAICS, PKM2 and PKM1 transcripts in lung adenocarcinomas. GAPDH transcript levels was used as control. D. Immunoblot analysis of lung adenocarcinoma tissue lysates (T) and matched normal samples (N) confirm increased expression of de novo purine biosynthetic enzymes PPAT, PAICS as well as specific PKM isoform PKM2 in lung cancer. PKM1 isoform expression was tested and did not show difference between normal and cancer tissues. β-actin was used as a loading control.
PPAT and PAICS overexpression predicts poor survival in lung cancer
Kaplan-Meier analysis using transcript data for PPAT (Fig. 2A and 2B; Supplementary Fig. S2D) and PAICS revealed (Fig. 2C and 2D; Supplementary Fig. S2D) that they predict poor patient overall survival (OS) and disease free survival (DFS) with increased expression of these genes in two independent studies [35, 40]. Similarly, elevated PKM2 expression was also correlated with poor patient survival (Supplementary Fig. S3A) [35, 40]. Multivariate Cox model analysis (with gene, age, gender, stage and differentiation in the model) indicated that PAICS, PPAT and PKM2 are also independently associated with patient survival in lung adenocarcinomas [HR 1.31 (1.05–1.64, P = 0.02), 1.41 (1.03–1.92, P = 0.03), 1.60 (1.18–2.17, P = 0.002) for PAICS, PPAT or PKM2 respectively]. Thus, our observations demonstrate that expression of PPAT, PAICS as well as PKM2 serve as biomarkers of disease progression. Tissue microarray analysis by immunohistochemistry (IHC) using specific antibodies against PPAT and PAICS showed increased staining in lung adenocarcinomas (Fig. (Fig.2E).2E). Furthermore, increased expression of PAICS is associated with poorly differentiated and more aggressive tumors (Fig. (Fig.2F).2F). Kaplan-Meier analysis of those samples also revealed that increased PAICS protein expression related to poor patient survival (Fig. (Fig.2G)2G) corroborating transcript (RNA) and protein data. In addition, PKM2 showed increased expression in adenocarcinomas (Supplementary Fig. S3B). Among the lung adenocarcinoma patients, smokers (both past and current) showed elevated PPAT and PAICS expression than non-smokers while PKM2 expression levels were elevated in lung adenocarcinoma patients above age 60 (Supplementary Table S1). In order to identify the possible genomic events for PPAT and PAICS dysregulation, we performed fluorescence in situ hybridization (FISH) with lung adenocarcinoma samples. We discovered aneuploidy and amplification in the divergently transcribed locus of PPAT and PAICS in a subset of lung adenocarcinoma (~3% cases) and amplification in H661 lung cancer cell line (Fig. (Fig.2H).2H). PPAT and PAICS are located within the 4q12 chromosomal segment that earlier has been reported to be amplified in NSCLC [41]. Thus amplification could be one of the potential mechanisms of increased expression of PPAT and PAICS in a subset of lung adenocarcinomas.
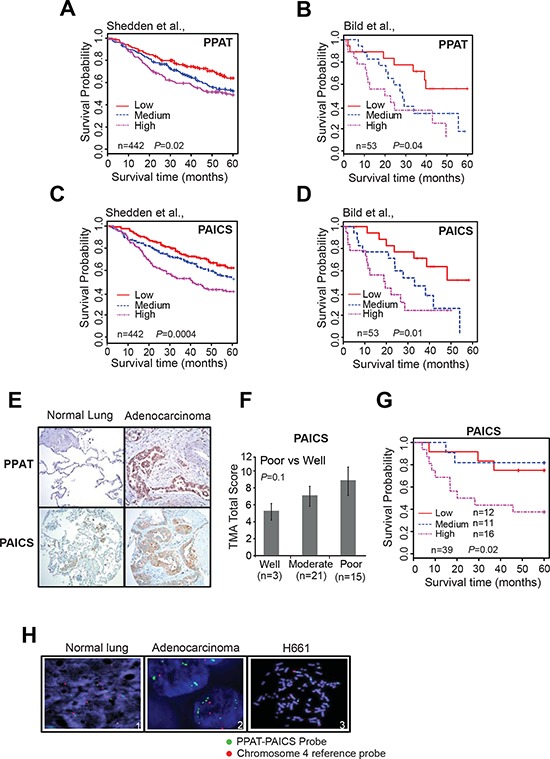
A–D. Kaplan-Meier (K-M) analysis of survival time according to the PPAT and PAICS transcript levels as measured using Affymetrix oligonucleotide microarray datasets by Shedden et al., [35] Bild et al. [40], respectively. The CEL files of microarray data were normalized using Robust Multi-array Average (RMA) method. Expression was classified into low, medium and high expressing groups. Five year survival time was used for K-M calculations. E. Photomicrographs of PPAT and PAICS immunostaining in normal lung (left) and lung adenocarcinoma tissues (right) using PPAT and PAICS-specific antibodies. F. Tumors are classified into well differentiated, moderately differentiated and poorly differentiated class based on the total score of immunostaining. G. The immunohistochemistry on a lung adenocarcinoma tissue microarray was scored and K-M survival curve was calculated based on staining intensity. Like transcript levels, protein expression was also sub-grouped into three classes. H. FISH was performed in normal and lung tumor tissues (Adenocarcinomas) and H661 (large cell carcinoma).
PPAT, PAICS and PKM2 are critical for lung cancer cell proliferation and invasion
Normal cells fulfill their purine and pyrimidine requirements through the salvage pathway. Highly proliferative and cancer cells are speculated to meet these requirements by activation of the de novo biosynthetic pathway [42]. We investigated the functional significance of the de novo purine biosynthetic pathway genes PPAT and PAICS in lung cancer by loss-of-function analyses of these divergently transcribed and elevated in lung tumors. Additionally, we evaluated the functional role of isoform of pyruvate kinase PKM2 in lung carcinomas since they were known to be activated in glucose depleted condition by the PAICS metabolite SAICAR. We performed knockdown experiments using two independent gene-specific siRNAs. Of note we custom synthesized siRNA duplexes for the PKM2 isoform [43]. Knockdown efficiency was confirmed by immunoblot analysis using specific antibodies (Fig. 3A, 3D and 3G) and by qRT-PCR (Supplementary Fig. S4A–S4C). Knockdown of each of these enzymes resulted in significant decrease in cell proliferation (Fig. 3B, 3E and 3H) and reduced cancer cell invasion as measured by Boyden chamber matrigel invasion assays (Fig. 3C, 3F and 3I), implicating a role for these genes in cancer cell growth and invasion. Our observation that PAICS knockdown inhibits cell proliferation concurs with similar observations in melanoma cells [18]. Cell cycle analyses revealed (Supplementary Fig. S5A and S5B) increase in S-phase population in the PPAT and PAICS knockdowns compared to controls. This observation is similar and consistent with slowing down of cell cycle when purine biosynthesis is blocked using anti-folates [44]. In addition, we found reduction in cell proliferation in H661, a large cell lung carcinoma cell line (Supplementary Fig. S6A–S6C) that harbors PPAT and PAICS gene amplification (Fig. (Fig.2H)2H) upon knockdown of PPAT, PAICS and PKM2. Knockdown efficiency was confirmed by immunoblot analysis using specific antibodies (Supplementary Fig. S6A–S6C, insets) and by qRT-PCR (Supplementary Fig. S4D–S4F). These in vitro experiments underscore role of PPAT, PAICS and PKM2 in proliferation and invasion across a cross-section of lung cancers cells.
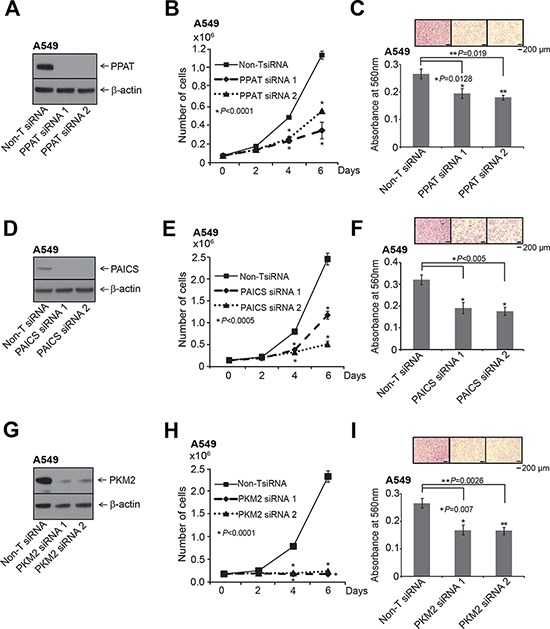
The knockdown of each gene was achieved using two independent siRNAs. A. PPAT gene specific, D. PAICS gene specific and G. PKM2 isoform specific siRNAs were used for the knockdown. A, D. and G. show immunoblot analysis to verify knockdown of respective genes. B, E. and H. PPAT, PAICS and PKM2 knockdown in A549 cells exhibit reduced cell proliferation. Asterisk indicates data is statistically significant (P < 0.05). The solid black line is for Non-specific target (Non-T) siRNA, the dashed line is for siRNA1, and dotted line is for siRNA2. C, F. and I. represent Boyden chamber matrigel invasion assay. Photomicrographs of invaded cells (purple) are shown in inset. For proliferation and invasion experiments, mean (n = 3) +/− SD is shown. Values with P <0.05 has been considered significant and marked with asterisk (*).
PPAT and PAICS expression modulate pyruvate kinase activity
Based on a published report that PAICS product, SAICAR acts as an allosteric activator of PKM2 under glucose depleted conditions [24], we hypothesized that apart from PAICS, PPAT expression also potentially can regulate pyruvate kinase activity. We first knocked down PKM2 and tested for Pyruvate Kinase (PK) activity in A549 lung adenocarcinoma cells. We observed a decrease in PK activity upon PKM2-targeted knockdown in normal medium compared to Non-T siRNA (Fig. (Fig.4A).4A). Moreover PPAT and PAICS knockdowns also showed significant decrease in PK activity (Fig. 4B and 4C, respectively). A similar decrease in PK activity was observed with PAICS (Supplementary Fig. S7A and S7B) and PKM2 (Supplementary Fig. S7C and S7D) knockdowns in H661 cells. Furthermore, our data shows a role for PPAT, an enzyme upstream of PAICS in de novo purine biosynthesis pathway in regulating PK activity.

A, B. and C. PK activity measured in PKM2, PPAT and PAICS knockdown A549 lung adenocarcinoma cell lines. Inset shows immunoblot confirming specific knockdown of the respective genes. D. Measurement of PK activity in A549 cells following treatment with RPMI+10% FBS, no glutamine and glutamine (0.5 mM and 2 mM) treatments. E. Immunoblot analyses of PPAT, PAICS and PKM2 following various treatments for 48 hrs. β-actin and total H3 were used as loading controls. F. Measurement of PK activity in A549 cells following treatment with glutamine (2 mM) and increasing concentration of DON after a period of 48 hrs. G. Immunoblot analyses with PPAT and PAICS in A549 cells following treatment with glutamine (2 mM) or increasing concentration of DON after 48 hrs. Minus glutamine has been considered as negative controls and β-actin has been used as loading control.
Glutamine regulates PK activity, induces PPAT and PAICS expression
Glutamine plays a critical role in cancer metabolism, [45, 46] and serves as a co-substrate for PPAT and PFAS. The final step of de novo pathway in which xanthine 5′-monophosphate is converted to guanosine 5′-monophosphate (GMP) also requires glutamine as amino donor [23]. We therefore tested the effect of glutamine both in regulation of PK activity as well PPAT and PAICS expression. Glutamine deprivation dramatically reduced A549 cell proliferation (Supplementary Fig. S8A) as observed previously [47]. Glutamine deprivation significantly reduced PK activity (Fig. (Fig.4D)4D) and moderately reduced PKM2 protein expression (Fig. (Fig.4E).4E). Treatment with similar concentrations of alanine failed to change PK activity over no glutamine controls (Supplementary Fig. S8B). In addition, pyruvate kinase activity and reduction in expression of PPAT and PAICS by glutamine deprivation could be entirely restored by exogenous addition of glutamine in A549 cells (Fig. 4D and 4E, respectively). We found similar, albeit less dramatic effects of glutamine deprivation on cell proliferation of H661 and H838 cells (Supplementary Fig. S8C and S8E, respectively), and PPAT and PAICS expression (Supplementary Fig. S8D and S8F), suggesting one of several critical roles of glutamine is in activating de novo purine biosynthesis pathway genes and altering cancer cell metabolism. Furthermore, treating A549 cells with increasing concentration of glutamine antagonist, L-diazo-5-oxo-L-norleucine (DON) [48] reduced PK activity (Fig. (Fig.4F)4F) and PPAT and PAICS expression (Fig. (Fig.4G)4G) even in presence of glutamine that were comparable to glutamine starved controls. Conversely, over-expression of PAICS in the benign lung cells, BEAS-2B resulted in increased PK activity (Supplementary Fig. 9A) and invasion (Supplementary Fig. 9B). Taken together, these results demonstrate that glutamine activates de novo purine biosynthetic pathway genes PPAT and PAICS and increases pyruvate kinase activity.
PPAT and PAICS play a role in lung tumor growth
Extending the in vitro studies described above, we next tested the role of PPAT and PAICS in two in vivo lung cancer xenograft models. We generated two independent stable knockdowns of PPAT and PAICS in A549 cells (Fig. 5A and 5B, inset) and used them in a chicken chorioallantoic membrane (CAM) assay that has been successfully used as an in vivo model to faithfully recapitulate several features of oncogenesis including tumor growth, local invasion and metastasis [49–51]. A549 cells with stable knockdown of PPAT or PAICS showed reduction in CAM tumor growth compared to cells with non-targeting shRNA controls (Fig. 5A and 5B, respectively). Similarly, H23 (lung adenocarcinoma cells) with PAICS knockdown (Supplementary Fig. S10A) showed reduced cell proliferation (Supplementary Fig. S10B) and a decrease in CAM tumor growth (Supplementary Fig. S10C). Next, we performed mouse xenograft experiments where athymic nude mice were injected subcutaneously with PPAT and PAICS stable knockdown A549 cells and tumors were subsequently measured. A significant reduction both in tumor growth and weight was observed in both PPAT (Fig. 5C and 5E, respectively) and PAICS (Fig. 5D and 5F, respectively) knockdowns compared to the control cells, demonstrating that PPAT and PAICS play essential role in lung tumor growth in vivo.

A. PPAT and B. PAICS knockdown using two independent shRNAs were utilized in the in vivo chicken chorioallantoic membrane (CAM) assay. Tumor growth was measured in PPAT and PAICS stable knockdown cells as well as in control A549 non-targeting shRNA cells (adenocarcinoma). Tumor size plotted correspond to average tumor size of 8 eggs per group and +/− SE per group (n = 8). C–F. Athymic nude mice were injected with A549 cells that had stable PPAT and PAICS knockdown separately and tumors were monitored over four weeks (C and D respectively); following which the mice were sacrificed and tumor weights were measured (E and F respectively). Non-targeting shRNA was used as control. The solid black line is for Non-T shRNA, the dashed line is for shRNA1 and dotted line is for shRNA2. Each value is tumor mean (n = 16) +/−SE from 8 mice; P <0.05 is considered as significant. G. Our study showed increased expression of de novo purine biosynthesis pathway genes PPAT and PAICS in lung cancer, their regulation by amplification and through induction by glutamine. This study also shows a role for PPAT and PAICS in lung tumorigenesis. Glutamine analog DON blocks the glutamine mediated induction of PPAT and PAICS. Increased expression of PPAT and PAICS modulates PK activity possibly by influencing levels of SAICAR [24], the product of PAICS in cancer cells. These enzymes therefore serve as effective therapeutic targets.
Our data demonstrate (Fig. (Fig.5G)5G) that PPAT and PAICS of de novo purine biosynthesis show increased expression in lung cancer compared to normal lung. PPAT and PAICS are two such genes that are co-transcribed from locus 4q12 and show genomic amplification in small percentage of lung cancer cases. Furthermore, our studies show that PPAT and PAICS play an oncogenic role in lung tumorigenesis. In addition, we show that PPAT and PAICS expression levels and pyruvate kinase activity are modulated by glutamine. Finally, PPAT and PAICS [24] levels regulate pyruvate kinase activity that is comparable to PKM2 depletion. This could possibly be through modulating levels of SAICAR, the PAICS enzyme product that directly interacts and activate PKM2 activity [24]. Further studies are needed to understand the exact mechanisms by which PPAT and PAICS modulate PK activity in normal glucose medium.
DISCUSSION
Recent reports from the World Health Organization warns of a dramatic global rise in cancer incidence and related mortality [52]. Lung cancer, which accounts for the most cancer-related deaths, is a heterogeneous disease and a leading cause of cancer-related mortality in the United States [1]. Non-small cell lung cancer (NSCLC) accounts for 90% of lung cancer while small-cell lung cancer (SCLC) accounts for the remainder [6]. Advancement in whole genome, exome and transcriptome sequencing technology has led to the stratification of lung cancer into specific molecular subtypes based on mutations they harbor [5]. For example, as discussed earlier, mutation in EGFR accounts for 10–30%, KRAS for 15–30%, and FGFR1 for 20% of NSCLC and targeted therapies against specific mutations has proven clinically more beneficial to patients compared to the cytotoxic chemotherapies [4]. Unfortunately, >50% of NSCLC harbor no targetable oncogenic driver mutations and many are usually diagnosed at an advanced stage. The intractable nature of lung cancer necessitates search for early diagnostic markers and identification of relevant therapeutic targets to improve patient prognosis. We showed that PPAT and PAICS of de novo purine biosynthesis are up-regulated in lung adenocarcinomas. Importantly, these genes are amplified in a subset of lung cancer cell lines as well in primary lung tumors. Of clinical significance is our observation that PPAT, PAICS and PKM2 (isoform of the PKM gene) transcript levels correlate with patient survival, differentiation status and smoking status. Furthermore, we also showed that PAICS protein expression predicts disease outcome. As indicated earlier, a clear molecular stratification has been instrumental in early detection and precise therapeutic regimen. In addition, we showed the critical role of PPAT and PAICS in lung cancer progression both in vitro and in vivo. Our study therefore reveals that PPAT and PAICS genes as potential therapeutic targets.
The PKM gene expresses two isoforms, PKM1 and PKM2 both differing by presence of a single exon in a mutually exclusive manner [25, 53]. An earlier study has shown correlation of high PKM2 levels and poor prognosis and demonstrated in vitro and in vivo role in lung cancer [54]. We demonstrated that specific knockdown of the PKM2 isoform dramatically reduces cell proliferation in wider spectrum of lung cancer cell lines including lung adenocarcinomas and large cell carcinoma. Recently SAICAR, the intermediate metabolite of the enzyme PAICS was shown to allosterically activate PKM2 activity under glucose depleting conditions [24] and mediate ERK1/2 phosphorylation thereby induces cancer cell proliferation [55, 56]. Interestingly, we discovered that PPAT and PAICS knockdown substantially diminished pyruvate kinase activity that is comparable to the PKM2 knockdown suggesting that PKM2 could be an important contributor of the pyruvate kinase activity in lung cancer cell lines. PKM2 isoform expression which is predominant in cancers, exists as both dimer and tetramer and plays a role in tumorigenesis in both forms [57]. Our study confirms increased PKM2 expression in lung cancer and regulation of pyruvate activity by de novo purine pathway genes can alter metabolic status and promote oncogenesis of lung cells. The oncogenic role of PPAT and PAICS that we observed therefore could be because of direct contribution to purine supply for efficient DNA replication during S-phase, as observed by our cell-cycle analyses and potentiating pyruvate kinase possibly through PKM2 activity.
De novo purine biosynthesis utilizes charged ribose-5-phosphate, known as phosphoribosyl pyrophosphate (PRPP) and glutamine as substrates by PPAT in the first committed step. Glutamine, a non-essential amino acid for normal cells, is essential for neoplastic transformation. Moreover most of the cancer cells won't survive in the absence of exogenous glutamine, and show glutamine addiction [58]. Recent study has shown that NSCLC cells show glutamine dependency [59]. Our studies confirmed that glutamine strongly effects lung cancer cell growth as well as in regulating pyruvate kinase activity. We showed that glutamine deprivation reduces PPAT and PAICS expression. Glutamine has multi-faceted function in cancer metabolism; including as primary carbon source replacing glucose [45, 58]. Substantial evidence suggests that increased uptake of glutamine in cancer cells undergoes the anapleurotic pathway, where it is converted into glutamate and then α-ketoglutarate (α-KG), a key intermediate in the tricarboxylic acid cycle [21]. We also observed that glutamine mediated induction of PPAT and PAICS expression and PKM2 activity which could be reversed by glutamine antagonist L-diazo-5-oxo-L-norleucine (DON). Glutamine dependency has been demonstrated in multiple cancers [58]. Direct targeting of glutamine dependency by administration of glutamine analogues such as DON, azaserine and acivicin showed their strongest effects on nucleotide biosynthesis [45]. These glutamine analogues showed potent anti-neoplastic effects in vitro [48]. However, efforts to incorporate them in clinical settings were stymied because of neurotoxicity, GI toxicity and myelosuppression [45, 58]. A concerted effort was made to circumvent toxicity issues by blocking glutamine availability to cancer cells either through inhibiting its uptake using GPNA(γ-L-glutamyl-p-nitroanilide) in lung cancer [60], or preventing its synthesis by inhibiting glutamine synthase using inhibitors such as BPTES(Bis-2-(5-phenylacetamido-1, 2, 4-thiadiazol-2-yl)ethyl sulfide) [61], compound 968 [62] and L-asparaginase [63]. Knocking down SLC1A5 [Solute Carrier Family 1 (Neutral Amino Acid Transporter), Member 5], the glutamine transporter has been demonstrated to inhibit tumor formation in acute myeloid mouse xenotransplant model [63]. Our study further illustrates the important role played by glutamine in inducing de novo purine biosynthetic genes PPAT and PAICS. We suggest that oncogenic effects of glutamine can be mitigated by potentially interfering with de novo purine biosynthesis pathway genes such as PPAT and PAICS or PKM2 activity. Inhibiting de novo purine pathway and PKM2 activity would also circumvent toxicity issues associated with glutamine antagonists as these genes are overexpressed in cancer cells.
In summary, we identified increased expression of PPAT and PAICS in de novo purine biosynthetic pathway that is potentially useful for lung cancer prognosis. Our studies indicated that both genes are amplified in a fraction of lung cancer patients. We demonstrated the functional significance of PPAT and PAICS during oncogenesis through several in vitro and in vivo studies and their role in regulating PKM2 activity. We established glutamine-mediated induction of PPAT and PAICS as an important metabolic event for increased expression of these genes during lung oncogenesis, which in turn enhances tumor-specific pyruvate kinase activity. Altogether, these results identify potentially novel targets for therapeutic intervention to treat lung cancer.
MATERIALS AND METHODS
Patient samples
We used frozen primary tumors and matched non-malignant lung samples from lung adenocarcinoma patients who underwent resection at the University of Michigan Health System from 1991–2007. Informed consent was obtained for each subject and clinical investigations were conducted after approval by the Institutional Review Board. Tumor specimens were immediately frozen following resection and stored at −80°C. Regions containing a minimum of 70% tumor cellularity were used for RNA/protein isolation. Tumor grade assessment as well as histopathological analysis of sections adjacent to regions used for RNA isolation was performed according the IASLC/ATS/ERS International Multidisciplinary Classification of Lung Adenocarcinoma [64, 65]. None of the patients included in this study received preoperative radiation or chemotherapy. Clinical data was retrospectively collected from the medical records and all cases were staged according to the revised 7th TNM classification criteria [65]. The median follow-up time was 8.12 years among the patients that remained alive.
Primary tumor-derived gene expression data sets
Two published Affymetrix microarray data sets were used in the survival analysis for genes PPAT, PAICS and PKM2 [35, 40]. The CEL files of microarray data were normalized using Robust Multi-array Average (RMA) method. The patient characteristics for Shedden et al, 442 tumors are provided in Supplementary Table S1. Overall survival is the outcome for all datasets, and it is censored at 5 years.
Cell line treatments
The cell lines used in this study were purchased from ATCC, USA. Lung cancer cell lines A549, H23, H661 and H838 were grown in 10% FBS-RPMI 1640 (Life Technologies, CA) in 5% CO2 cell culture incubator. The reagents L-glutamine and L-diazo-5-oxo-L-norleucine (DON) are purchased from Sigma-Aldrich, USA. As described elsewhere [60, 66, 67], for glutamine studies, the cells were plated in 6-well tissue culture plates at a density of 0.2 million cells/well. The following day, the cells were washed twice with serum free glutamine-free medium and replaced with 10% dialyzed FBS containing glutamine-free RPMI 1640 (Life Technologies, CA), and then incubated for 48 h. The corresponding glutamine-replete medium was prepared by addition of indicated concentration of glutamine. For DON (a glutamine analogue) studies, we added indicated concentration of DON to the cells which are already in 10% dialyzed FBS + 2 mM Gln+ RPMI 1640 medium [68, 69]. Bronchial lung epithelial cells, BEAS-2B were grown in BEBM medium with supplements (Lonza, USA). Adenoviruses and lentiviruses were generated by the University of Michigan Vector Core. Lung cancer cells were infected with lentiviruses expressing PPAT shRNAs, PAICS shRNAs or Non-target controls only, and stable cell lines were generated by selection with 1 μg/ml puromycin (Life Technologies, CA).
Pyruvate kinase activity assay
The pyruvate kinase activity was measured using Pyruvate Kinase Assay kit (Biovision, Catalog #K709-100). Briefly, cell pellets were suspended in 4x assay buffer (10,000 cells/100 μl). The lysed cells were spun (10,000 rpm/5 mins) to clear cell debris and cell supernatant was used to measure pyruvate kinase activity colorimetrically (λ = 570 nm) using 96 well-plate assays. The PK activity as measured by pyruvate production was calculated using standard PK activity provided by the kit. The calculated activity was then normalized to protein concentration of the cell lysate and plotted. The y-axis represents the percentage normalized PK activity (% μmol pyruvate produced/mg of protein).
Immunoblot analysis
For immunoblot analysis, 10 μg of lung adenocarcinoma tissue lysates from regions >70% tumor cellularity and matched normal tissue lysates were used. Tissue and lung cancer cell line lysates were boiled in sample buffer, separated by sodium dodecyl sulfate-polyacrylamide gel electrophoresis, and transferred onto polyvinylidene difluoride membrane (GE Healthcare, Piscataway, NJ). First lane contains 5 ul of spectra multicolor broad range protein ladder (Life Technologies, CA). The membrane was incubated for 1 hour in blocking buffer (TBS, 0.05% Tween, 5% nonfat dry milk) and incubated overnight at 4°C with respective primary antibodies, and signals were visualized after incubating with secondary antibody conjugated with HRP. The list of various antibodies, catalogue numbers and dilutions used are mentioned in Supplementary Table S2.
RNA and DNA isolation and quantitative real-time PCR assays
Total RNA was isolated from tissues and cells using RNeasy Mini Kit (Qiagen, USA). Quantitative PCR (qRT-PCR) with cDNA (Applied Biosystems, USA) was performed with 10 ng of cDNA used as template and quantified using SYBR (Applied Biosystems, USA). qRT-PCR assays were performed in triplicate. Both PKM1 and PKM2 qRT-PCR primers were taken from elsewhere [43]. The primer sequences for the transcript analyzed by SYBR green qRT-PCR as provided in Supplementary Table S3.
Immunohistochemistry of TMA
Immunohistochemistry on lung adenocarcinoma tissue microarray was performed using PPAT mouse monoclonal antibody, PAICS mouse monoclonal antibody and PKM2 specific rabbit monoclonal antibody. Immunohistochemistry was performed using an automated protocol developed for the DISCOVERY XT automated slide staining system (Ventana Medical Systems, Inc.,) using Ultramap anti-mouse HRP or Ultramap anti-rabbit HRP as secondary antibody and was detected using ChromoMap DAB (Cat#760-159, Ventana Medical Systems Inc.,) Hematoxylin II (Cat#790-2208 Ventana-Roche, Tucson, AZ, USA) was used as the counterstain. Immunohistochemistry staining was evaluated by D.T. TMA sections were scored for the proportion of tumor cells staining for the marker and intensity using the methodology described by Harvey et al [70].
Fluorescence in situ hybridization (FISH)
BAC clones were used to generate the locus specific (4q12) FISH probe for PAICS and PPAT (RP11-393M11) and chromosome 4 control probe (RP11-719O22). All clones were tested on normal human metaphase chromosomes to validate map position. PAICS locus probe and chromosome 4 control probes were detected with anti-digoxigenin fluorescein Fab fragments to yield green color and Streptavidin Alexa fluor 594 to yield red color, respectively. 200 ml overnight cultures for each BAC clone were grown in LB medium containing 12.5 μg/ml of chloramphenicol at 37°C for 14–16 hours with constant shaking. DNA was prepared using Qiagen-midiprep kit using Qiatip-100 according to the protocol provided by the manufacturer (Qiagen, USA).
All FISH probes were prepared by nick translation labeling using modified nucleotides conjugated with biotin or digoxigenin utilizing biotin nick translation mix (Cat# 11745824910, Roche, USA) for chromosome 4 control probe; digoxigenin nick translation mix (Cat# 11745816910, Roche, USA) for PAICS locus probe, respectively. Probe DNA was precipitated and dissolved in hybridization mixture containing 50% formamide, 2XSSC, 10% dextran sulphate, and 1% Denhardt's solution. Approximately 200ng of each labeled probe was used for hybridization. Fluorescent signals were detected with Streptavidin Alexa fluor 594 (Cat# S-32356, Invitrogen, USA) and anti-digoxigenin fluorescein Fab fragments (Cat# 11207741910, Roche, USA) for red and green colors, respectively. FISH scoring was performed by an experienced cytogeneticist (NP). Copy number was evaluated based on the ratio between control and locus specific probe. Copy number 4 or above are considered as amplification in at least 15–20% of the cells in the tumor samples. Fluorescent images were captured using a high resolution CCD camera controlled by ISIS image processing software (Metasystems, Germany).
siRNA transfections
Custom made small interfering RNA (siRNA) duplexes for RNA interference of PPAT, PAICS were purchased from Dharmacon, Lafayette, CO (GE Healthcare, USA). PKM2 specific siRNA was custom synthesized from Dharmacon, Lafayette, CO (GE Healthcare, USA) [43]. The list of siRNAs, shRNAs and the catalogue numbers are mentioned in Supplementary Table S4. Transfections were performed with either oligofectamine (Life Technologies, USA) or Lipofectamine® RNAimax (Life technologies, USA). Seventy-two hours after the siRNA transfections, the cells were harvested for RNA isolation or lysed for immunoblot analysis. Short hairpin RNA (shRNA) constructs were generated using pGreen-puro vector (System Biosciences, Mountain View, CA). For PPAT knockdown, the pGIPz plasmids were obtained from Dharmacon, Lafayette, CO (GE Healthcare, USA). All the lentiviruses were generated by the University of Michigan Vector Core.
Cell proliferation assays
Cell proliferation was measured by cell counting. For this, stable and/or transient PAICS, PPAT and PKM2 knockdowns were used. Around 10,000 cells/well were seeded in 24-well plates (n = 4), after transfecting either with specific siRNA duplex or stable knockdowns as described above. Stable knockdown of PAICS was performed using shRNA strategy using lentiviral construct with specific duplex sequences targeting PAICS. These stable knock down cells were plated at same density as mentioned earlier, harvested and counted at specified time points by Coulter counter (Beckman Coulter, Fullerton, CA). Non-targeting siRNA or shRNA-treated cells were served as controls. For glutamine studies, as mentioned earlier lung cancer cell lines were seeded in same density in glutamine-free medium and counted the cells after each indicated time point. Each experiment has been carried at thrice with four replicates in each experiment per sample. Data shown is representative figure of one such experiment.
Cell cycle analysis
siRNA treated cells (as mentioned earlier) were trypsinized and resuspended in 0.5 ml of PBS at a concentration of 106cells/ml. Ice-cold absolute ethanol was added in equal volumes to the sample. The sample was incubated for minimum of 20 min, followed by spin at 2000 rpm for 2–5 min. Ethanol was decanted and cell pellet was resuspended in 0.5 ml propidium iodide+RNase solution and incubated at room temperature for minimum of 20 min in dark. The cell cycle analysis was carried through flow cytometry core facility, University of Michigan, Ann Arbor. Each experiment has been carried at twice in triplicate per sample. Data shown is representative figure of one such experiment.
Matrigel invasion assay
For invasion assays, transiently transfected or stable knockdown of PPAT, PAICS and PKM2 in were used in A549 cells. BEAS-2B (benign transformed lung epithelial) cells were infected with PAICS-adenovirus. Seventy-two hours post-transfection and/or infection, cells were seeded onto BD BioCoat matrigel matrix (BD, CA) present in the insert of a 24-well culture plate. For A549, 10% serum containing RPMI medium was added to the lower chamber as a chemoattractant. For BEAS-2B, BEBM medium with supplementary growth factors was added as chemoattractant. After 36 h, the non-invading cells and matrigel matrix were gently removed with a cotton swab. Invasive cells located on the lower side of the chamber were stained with 0.2% crystal violet in methanol, air-dried and photographed. They were then enumerated microscopically using multiple representative areas. For colorimetric assays, the inserts were treated with 150 μl of 10% acetic acid and the absorbance measured at 560 nm [71]. Each experiment has been carried twice in triplicates per sample. Data shown is representative figure of one such experiment.
In vitro overexpression
PAICS cDNA (Origene, MD; Cat# RC223925) was cloned into Gateway expression system (Life Technologies CA). To generate adenoviral construct, PCR8-PAICS (flag tagged) was recombined with pAD/CMV/V5-Dest™ (Life Technologies, CA) respectively using LR Clonase II (Life Technologies, CA) [71]. Adenoviruses were generated by the University of Michigan Vector Core. Benign lung epithelial cells (BEAS2-B) were infected with adenoviruses expressing PA1CS or lacZ for transient over-expression.
Chicken chorioallantoic membrane (CAM) assay
The CAM assay for tumor (or xenograft) formation was performed as previously described [49, 51, 72]. Briefly, fertilized eggs were incubated in a rotary humidified incubator at 38°C for 10 days. CAM was dropped by making two holes, one through the eggshell into the air sac and a second hole near the allantoic vein that penetrates the eggshell membrane but not the CAM. Subsequently, Dremel 3000 Rotary Tool was used to cut a 1 cm2 window to expose the underlying CAM near the allantoic vein. Approximately 2 million cells in 50 μl of media were implanted in each egg, windows were sealed and the eggs were returned to the incubator. Following 7-day incubation post-tumor cells injection; the primary tumor was excised and weighed. Around 8 eggs per group were used in each experiment.
Tumor xenograft model
All procedures involving mice were approved by the University Committee on Use and Care of Animals at the University of Michigan and conform to their relevant regulatory standards. To evaluate the role of PPAT and PAICS in tumor formation, we propagated stable PPAT and PAICS knockdown A549 pools using two-independent shRNAs, and Non-targeting shRNA control cells and inoculated 1 × 106 cells subcutaneously into the dorsal flank of 4-week-old male athymic nude mice (Foxn1nu) (n = 8 for each group; Harlan Laboratories, USA). Tumor size was measured biweekly, and tumor volumes were calculated using the formula (π/6) (L × W2), where L = length of tumor and W = width and represented as mm3/tumor. Post-monitoring, tumors were dissected out, photographed and weighed. Tumor weight is represented in mg/tumor.
Statistical evaluation
For cell lines and pyruvate kinase assays representative readings in triplicate was averaged and plotted in various graphs. The error indicates the standard deviation (SD) in case of colorimetric assays and cell counts. In case of tumor grafts, the error bars represent standard error (SE) instead of SD. For CAM assays, 8 eggs have been used per treatment while for mice experiments, values represent average of 8 mice/group. Statistical evaluation of the data was carried out using student's t-test and values that showed P <0.05 were considered significant.
The clinical and pathological characteristics were analyzed using the Student's t-test for comparing two groups and by ANOVA for multiple group comparisons, with P < 0.05 considered statistically significant. For values that were not normally distributed the Mann-Whitney rank sum test was used. Univariate and multivariate (adjusted by age, gender, stage and differentiation) Cox proportional hazards regression model were used for continuous values of each gene; Kaplan-Meier survival curve and log-rank test were used to separate patients into three risk tertiles (high, medium, and low-risk, 1/3rd in each group) based on gene/protein expression levels.
Acknowledgments
We would like to thank Jyoti Athanikar for critical reading of the manuscript and editing. We also thank the University of Michigan Vector Core for generating adenovirus and lentivirus. We acknowledge the help of the University of Michigan Flow Cytometry Core for experiment and analysis of flow data, and the Sequencing core for sequencing.
Footnotes
CONFLICTS OF INTEREST
The authors declare no competing financial interests. Correspondence and requests for materials should be addressed to S.V. and D.B.
GRANT SUPPORT
This work was supported in part by the National Institutes of Health through grant R01CA157845 and R01CA154980 (S. Varambally). This work was supported in part by R01CA154365 to (D.G. Beer and A.M. Chinnaiyan) and through the University of Michigan's Cancer Center Support Grant (5 P30 CA46592).
REFERENCES
Articles from Oncotarget are provided here courtesy of Impact Journals, LLC
Full text links
Read article at publisher's site: https://doi.org/10.18632/oncotarget.4352
Read article for free, from open access legal sources, via Unpaywall:
https://www.oncotarget.com/article/4352/pdf/
Citations & impact
Impact metrics
Citations of article over time
Alternative metrics
Article citations
One-carbon-mediated purine synthesis underlies temozolomide resistance in glioblastoma.
Cell Death Dis, 15(10):774, 25 Oct 2024
Cited by: 0 articles | PMID: 39455562 | PMCID: PMC11511812
TMSB4Y restrains sphingomyelin synthesis via de novo purine synthesis to exert a tumor suppressor function in male esophageal squamous cell carcinoma.
Oncogene, 23 Oct 2024
Cited by: 0 articles | PMID: 39443723
Connecting dots between nucleotide biosynthesis and DNA lesion repair/bypass in cancer.
Biosci Rep, 44(9):BSR20231382, 01 Sep 2024
Cited by: 0 articles | PMID: 39189649 | PMCID: PMC11427732
Review Free full text in Europe PMC
Integrated network pharmacology and metabolomics to reveal the mechanism of Pinellia ternata inhibiting non-small cell lung cancer cells.
BMC Complement Med Ther, 24(1):263, 11 Jul 2024
Cited by: 0 articles | PMID: 38992647 | PMCID: PMC11238457
Exploring Molecular Mechanisms and Biomarkers in COPD: An Overview of Current Advancements and Perspectives.
Int J Mol Sci, 25(13):7347, 04 Jul 2024
Cited by: 0 articles | PMID: 39000454
Review
Go to all (60) article citations
Data
Data behind the article
This data has been text mined from the article, or deposited into data resources.
BioStudies: supplemental material and supporting data
Similar Articles
To arrive at the top five similar articles we use a word-weighted algorithm to compare words from the Title and Abstract of each citation.
Expression and Role of PAICS, a De Novo Purine Biosynthetic Gene in Prostate Cancer.
Prostate, 77(1):10-21, 22 Aug 2016
Cited by: 29 articles | PMID: 27550065
Phosphoribosyl pyrophosphate amidotransferase: Novel biomarker and therapeutic target for nasopharyngeal carcinoma.
Cancer Sci, 115(11):3587-3595, 28 Aug 2024
Cited by: 0 articles | PMID: 39196700 | PMCID: PMC11531959
Mapping of the bovine genes of the de novo AMP synthesis pathway.
Anim Genet, 35(6):438-444, 01 Dec 2004
Cited by: 9 articles | PMID: 15566465
PAICS as a potential target for cancer therapy linking purine biosynthesis to cancer progression.
Life Sci, 331:122070, 04 Sep 2023
Cited by: 2 articles | PMID: 37673296
Review
Funding
Funders who supported this work.
NCI NIH HHS (8)
Grant ID: R01CA154980
Grant ID: 5 P30 CA46592
Grant ID: R01 CA157845
Grant ID: R01 CA154980
Grant ID: R01CA154365
Grant ID: R01CA157845
Grant ID: P30 CA046592
Grant ID: R01 CA154365