Abstract
Free full text

Parallel evolution of influenza across multiple spatiotemporal scales
Abstract
Viral variants that arise in the global influenza population begin as de novo mutations in single infected hosts, but the evolutionary dynamics that transform within-host variation to global genetic diversity are poorly understood. Here, we demonstrate that influenza evolution within infected humans recapitulates many evolutionary dynamics observed at the global scale. We deep-sequence longitudinal samples from four immunocompromised patients with long-term H3N2 influenza infections. We find parallel evolution across three scales: within individual patients, in different patients in our study, and in the global influenza population. In hemagglutinin, a small set of mutations arises independently in multiple patients. These same mutations emerge repeatedly within single patients and compete with one another, providing a vivid clinical example of clonal interference. Many of these recurrent within-host mutations also reach a high global frequency in the decade following the patient infections. Our results demonstrate surprising concordance in evolutionary dynamics across multiple spatiotemporal scales.
eLife digest
Influenza or flu viruses change fast to escape the body’s defenses. While a single course of vaccines will protect someone against polio or measles for their whole life, people need a new flu shot every year to be protected against influenza. Also, some years the flu vaccine is not as effective as hoped because the virus has changed in an unpredictable way.
All of the change that happens in flu viruses around the world ultimately begins in individual infections, as random mistakes or mutations in the virus’s genetic material that arise as the viruses replicate. Mutations that help the virus aid in its ability to spread from person to person, and eventually spread around the world. As such, understanding flu’s evolution within individual people may help scientists to understand and eventually predict how it changes worldwide. Yet, unlike some viral infections that last months or years, flu infections are usually short and over in a few days. This makes it harder to measure how the viruses change over time in a single infection.
To get around this issue, Xue et al. analyzed flu samples taken over several weeks from four cancer patients who had longer-than-average flu infections because of their weaker immune systems. In some cases, the exact same mutations were seen in viruses from two or more of the patients. Also, some of the mutations that happened within the patients were the same mutations that later went on to spread around the world. These findings show that the flu virus can change in a single person in some of the same ways that it has been seen to change around the world.
Xue et al. studied how flu changes in people with weak immune systems, who are infected for longer periods of time. Further studies are needed to reveal more about how flu viruses evolve in the more typical, shorter infections in otherwise healthy people. This kind of investigation is becoming easier because new methods are making it possible to examine the genetic material from many viruses at once. It is hoped that eventually, detecting mutations in individual infections could help predict how viruses will change worldwide, which might help researchers to design vaccines that will be more effective each year.
Introduction
Viruses rapidly acquire de novo mutations as they replicate within infected hosts (Andino and Domingo, 2015), but only a small fraction of these variants transmit between hosts and eventually fix on a global scale. Within hosts, a mutation’s impact on viral replication and immunogenicity affect whether it increases in frequency. At larger scales of space and time, transmission bottlenecks (Varble et al., 2014; Poon et al., 2016) and host heterogeneity also shape viral genetic diversity. The selective pressures at these various scales reflect complex molecular, immunological, and epidemiological constraints (Grenfell et al., 2004; Pybus and Rambaut, 2009; Luksza and Lässig, 2014; Neher et al., 2016), which have formed the basis of recent efforts to forecast influenza evolution (Luksza and Lässig, 2014; Neher et al., 2016, Neher et al., 2014; Lässig et al., 2017).
Influenza’s rapid global evolution has been the subject of intense study (Ghedin et al., 2005; Rambaut et al., 2008), but the origins of this variation within single infected hosts are still poorly understood. Recent deep-sequencing studies of human clinical samples suggest that influenza accumulates relatively limited genetic diversity within hosts during most acute infections (Dinis et al., 2016; Poon et al., 2016; Sobel Leonard et al., 2016; Debbink et al., 2017), in line with earlier studies in dogs and horses (Murcia et al., 2010; Hoelzer et al., 2010). Some within-host mutations may confer novel antigenic properties (Dinis et al., 2016), but most lack clear functional interpretation. Altogether, it remains unclear how influenza’s within-host diversity is transformed into global evolution.
Influenza infections usually last less than a week and provide limited opportunity for longitudinal study. But among some immunocompromised patients, infections can last weeks or months (Nichols et al., 2004; Memoli et al., 2014), making it possible to examine longer-term within-host evolutionary dynamics (Rocha et al., 1991; McMinn et al., 1999; Rogers et al., 2015). Here, we use deep-sequencing to characterize the evolutionary dynamics of influenza within immunocompromised hosts. We identify a small set of mutations that arise repeatedly within individual patients, across multiple patients in our study, and at the global scale, revealing surprising similarities in evolutionary dynamics across multiple spatiotemporal scales.
Results
The same mutations often arise in multiple patients
We deep-sequenced 37 viral samples collected longitudinally from four immunocompromised patients with long-term H3N2 influenza infections in the 2005–2006 and 2006–2007 seasons (Figure 1). These patients developed influenza infections in the months after receiving hematopoietic cell transplantations when immune cell counts were still low, and nasal wash samples were collected approximately every week. All patients were treated with the neuraminidase inhibitor oseltamivir for at least some duration of their infections (Campbell et al., 2015) (Figure 1, Figure 1—figure supplement 1).
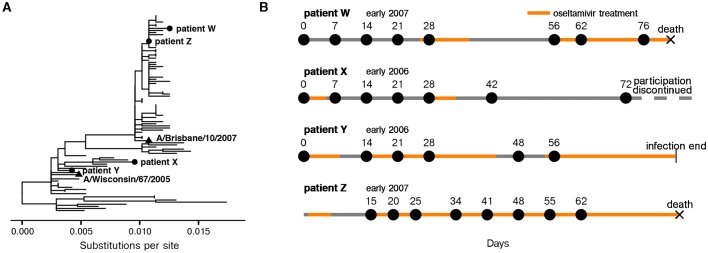
(A) Phylogenetic relationship between initial patient consensus sequences and 63 unique circulating influenza strains collected in the USA from 2004 to 2007, as inferred from the HA gene. (B) Overview of patient influenza infections and treatments. Periods of oseltamivir treatment are shown in orange. Dates of sequenced nasal wash samples are calculated relative to the first influenza-positive nasal wash. Low-quality samples are not shown here and were excluded from downstream analysis. Materials and methods and Figure 1—figure supplement 1 give full clinical histories.
Figure 1—figure supplement 1.
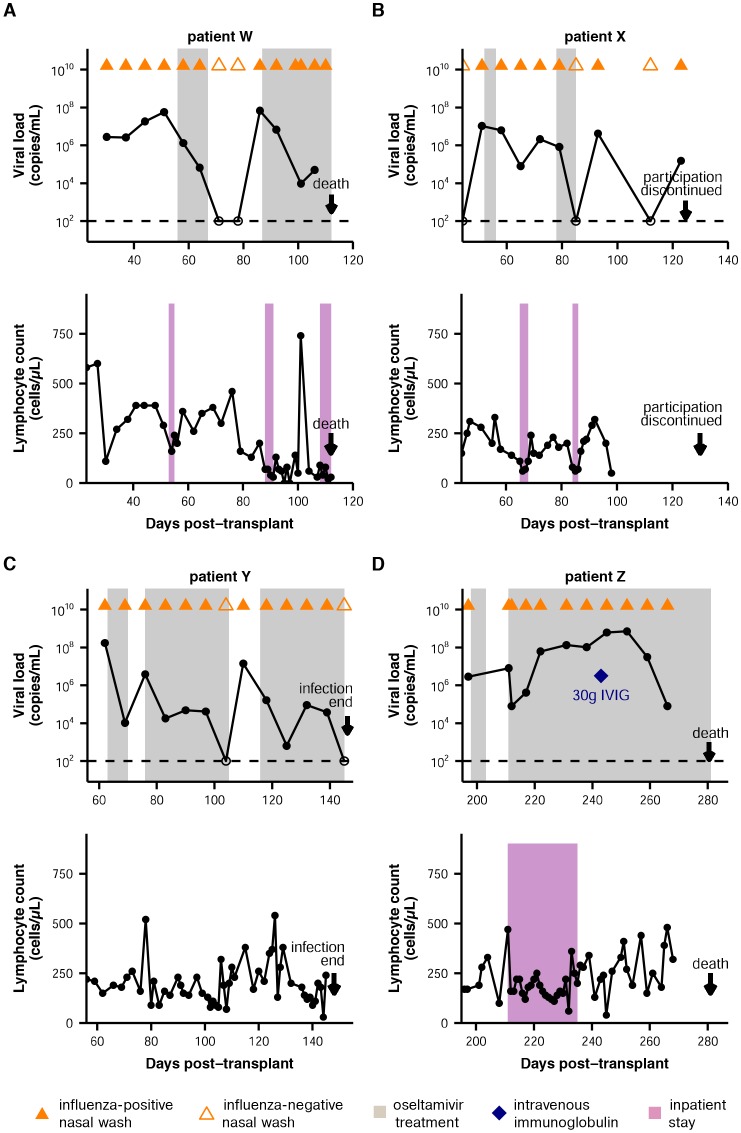
Influenza viral load over time, as quantified by qRT-PCR, and lymphocyte counts over time are shown for all patients. For the plots of viral load, nasal wash samples are marked with triangles, with influenza-positive nasal washes indicated by solid coloring. Oseltamivir treatment is indicated by gray shading. Intravenous immunoglobulin treatment is marked with a blue diamond. The limit of detection is marked with a dashed line. For the plots of lymphocyte counts, inpatient stays are indicated in purple.
We sequenced the full viral genome to high coverage directly from patient nasal wash samples by using influenza-specific reverse transcription and PCR (Hoffmann et al., 2001) to enrich for viral genetic material (Figure 2—figure supplement 1). To limit the impact of library preparation and sequencing errors on estimates of variant frequency (McCrone and Lauring, 2016), we prepared sequencing libraries in duplicate for each sample, beginning from separate reverse-transcription reactions. We excluded from downstream analyses eight low-quality samples for which sequencing coverage was low or variant frequencies differed greatly between replicates (Figure 2—figure supplement 1).
Across the influenza genome, de novo mutations arise most commonly in the surface proteins hemagglutinin (HA) and neuraminidase (NA) (Figure 2A), which undergo rapid global evolution (Bhatt et al., 2011). These mutations fluctuate in frequency but rarely fix, showing that complex evolutionary dynamics can emerge within single infected individuals (Figure 2B, Figure 2—figure supplements 2–5). We focused on within-host mutations that reached a frequency of at least 5% in two independent sequencing replicates from any patient sample. Many nonsynonymous mutations occur at sites that affect the antigenicity of HA (Koel et al., 2013) and the antiviral sensitivity of NA (Baz et al., 2006; van der Vries et al., 2013) (Figure 2—figure supplement 6). In NA in particular, we observe the emergence and persistence of mutations T242I and R292K, which are known to be associated with oseltamivir resistance (Baz et al., 2006; van der Vries et al., 2013), a phenomenon of strong clinical importance (Renaud et al., 2011) (Figure 2—figure supplements 2–5).
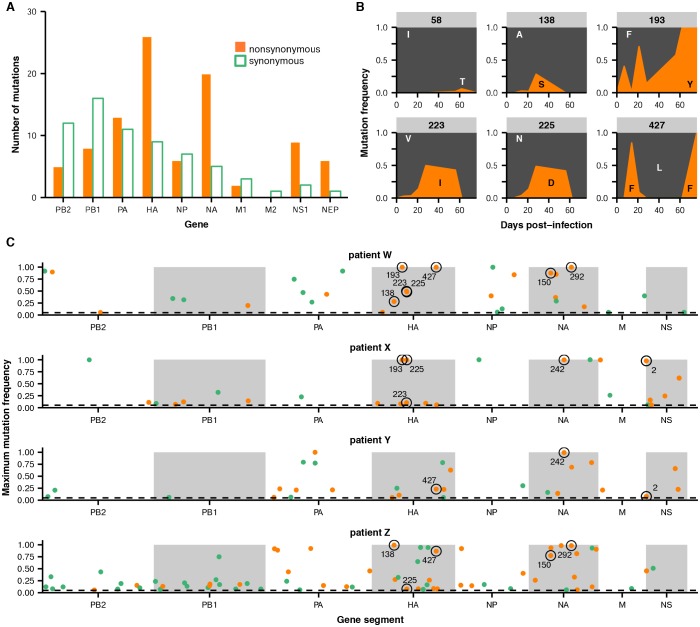
(A) Number of nonsynonymous (orange) and synonymous (green) variants in each influenza gene. We identified within-host viral mutations that reached a frequency of at least 5% in two independent sequencing replicates from any patient sample. (B) Frequencies over time for all HA mutations in patient W. Each subplot represents a site in HA and is labeled by codon number. Ancestral identities are colored in gray and mutant ones in orange. (C) Maximum frequencies reached by all nonsynonymous (orange) and synonymous (green) mutations in each patient. Mutations circled in black emerged independently in multiple patients and are labeled by codon number. The dotted line indicates the minimum frequency threshold of 5%. Materials and methods and Figure 2—figure supplement 1 describe procedures used for variant calling and quality control. Figure 2—figure supplements 2–5 give full frequency trajectories for all mutations in all patients. Figure 2—figure supplement 6 shows mutations in HA and NA on their respective crystal structures. Figure 2—figure supplement 7 describes permutation tests that assess the significance of the observed parallelism between patients.
DOI: http://dx.doi.org/10.7554/eLife.26875.005
Figure 2—source data 1.
Primers used for viral deep sequencing.5’ primer tail sequence is indicated in plain text, homology to the U12/U13 regions in bold text, and gene-specific sequence in bold underlined text. Primers were modified from the universal primers described in (Hoffmann et al., 2001) to account for the A/G polymorphism at the U4 site of the U12 region.
DOI: http://dx.doi.org/10.7554/eLife.26875.006
Figure 2—figure supplement 1.
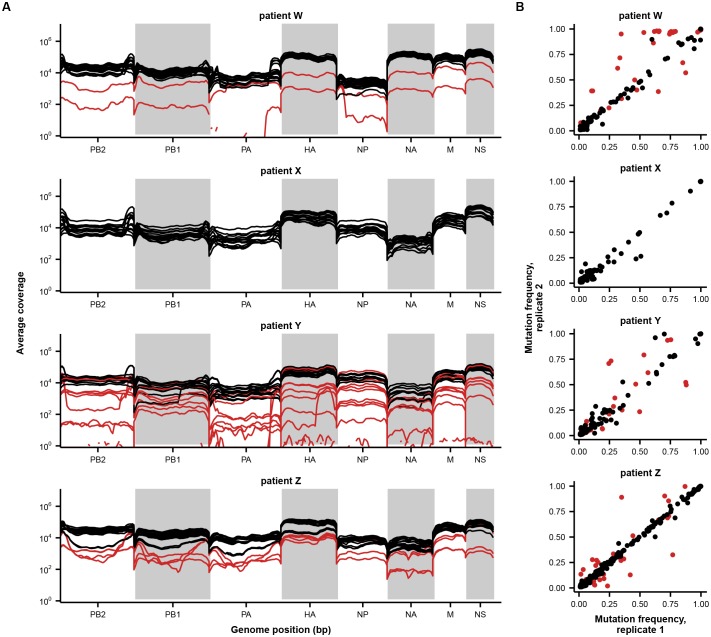
(A) Sequencing coverage across the genome. Average sequencing coverage for each sample and library replicate is shown in 50 bp bins across the genome. Each line indicates a separate library replicate. Low-quality samples are colored in red and were not included in downstream analyses. (B) Correlation between mutation frequencies in replicate sequencing libraries. Mutations in low-quality samples are colored in red and were excluded from downstream analyses. These samples were not shown in Figure 1B. A mutation was called if a base other than the initial consensus base reached a frequency of at least 1% with a total coverage of at least 200 reads in both replicates. Note that this variant calling threshold is more lenient than the 5% threshold used to call variants in downstream analyses; this more lenient threshold identifies more variants to get a better measurement of replicability. Samples were excluded from downstream analyses if the average difference between mutation frequencies exceeded 0.05. Replicate libraries were prepared beginning with independent reverse-transcription reactions.
Figure 2—figure supplement 2.
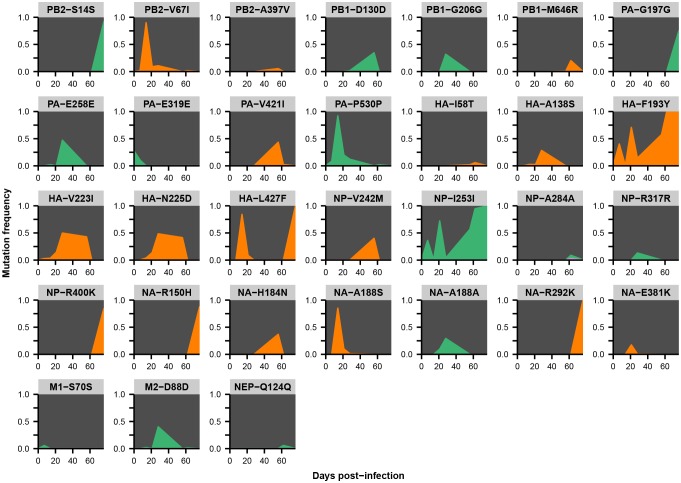
Mutation frequencies over the course of the infection are shown at all variable sites in patient W. Ancestral identities are colored in gray, derived nonsynonymous identities in orange, and derived synonymous identities in green. Sites were called as variant if a base other than the initial consensus reached a frequency of at least 5% in both replicate libraries of at least one sequenced time point. The frequencies shown here are the average of the two replicates. Mutations located in the overlap of M1/M2 or NS1/NEP genes are displayed twice with the appropriate annotation for each gene.
Figure 2—figure supplement 3.
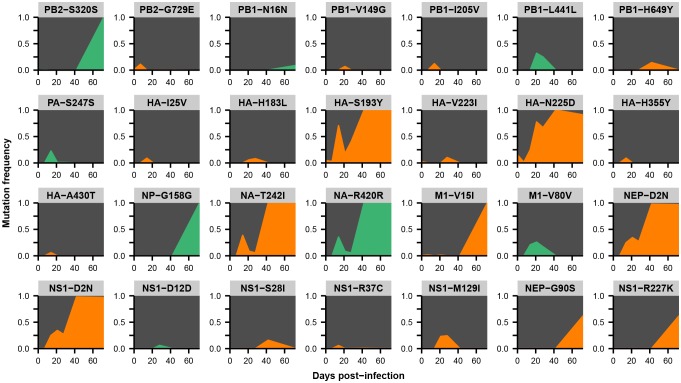
Mutation frequencies over the course of the infection are shown at all variable sites in patient X. Ancestral identities are colored in gray, derived nonsynonymous identities in orange, and derived synonymous identities in green. Sites were called as variant if a base other than the initial consensus reached a frequency of at least 5% in both replicate libraries of at least one sequenced time point. The frequencies shown here are the average of the two replicates. Mutations located in the overlap of M1/M2 or NS1/NEP genes are displayed twice with the appropriate annotation for each gene.
Figure 2—figure supplement 4.
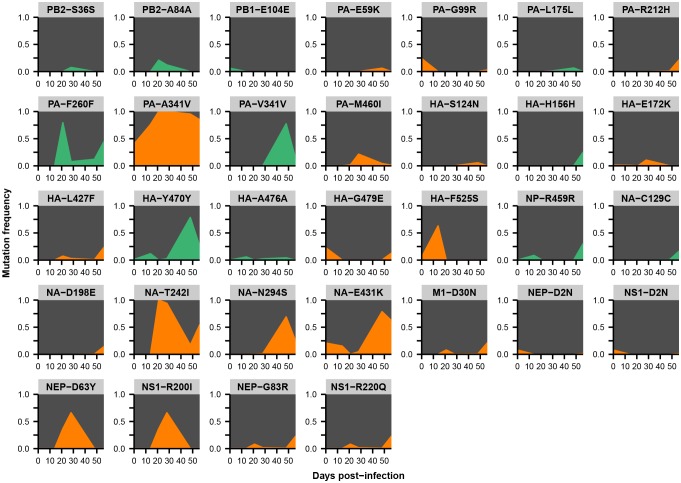
Mutation frequencies over the course of the infection are shown at all variable sites in patient Y. Ancestral identities are colored in gray, derived nonsynonymous identities in orange, and derived synonymous identities in green. Sites were called as variant if a base other than the initial consensus reached a frequency of at least 5% in both replicate libraries of at least one sequenced time point. The frequencies shown here are the average of the two replicates. Mutations located in the overlap of M1/M2 or NS1/NEP genes are displayed twice with the appropriate annotation for each gene.
Figure 2—figure supplement 5.
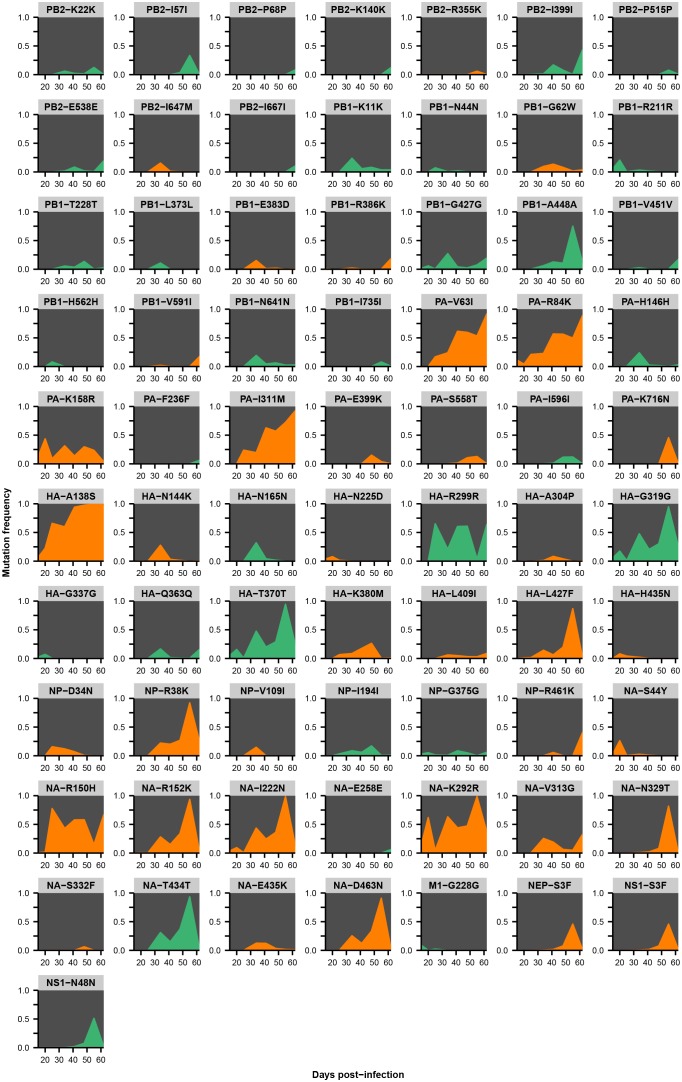
Mutation frequencies over the course of the infection are shown at all variable sites in patient Z. Ancestral identities are colored in gray, derived nonsynonymous identities in orange, and derived synonymous identities in green. Sites were called as variant if a base other than the initial consensus reached a frequency of at least 5% in both replicate libraries of at least one sequenced time point. The frequencies shown here are the average of the two replicates. Mutations located in the overlap of M1/M2 or NS1/NEP genes are displayed twice with the appropriate annotation for each gene.
Figure 2—figure supplement 6.
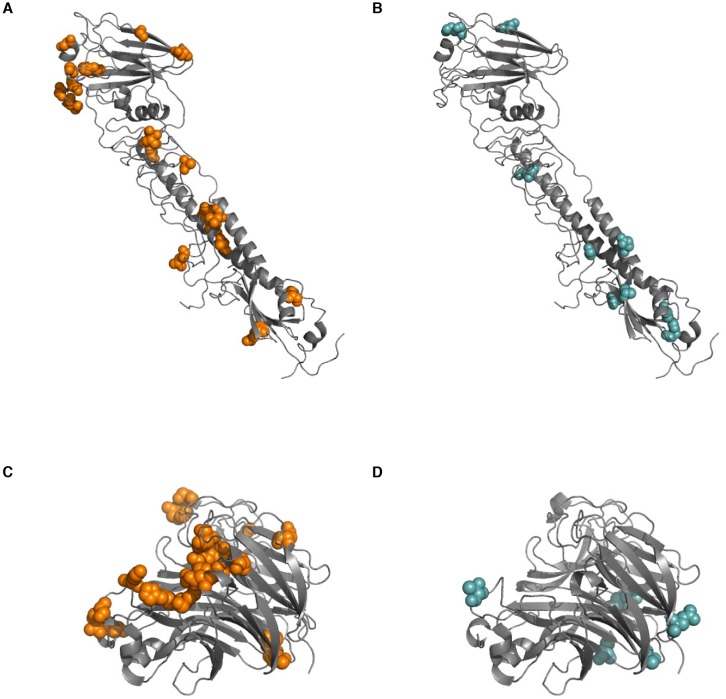
Sites of (A) nonsynonymous and (B) synonymous within-host mutations are shown on an HA crystal structure (PDB 4HMG [Weis et al., 1990]). Sites of (C) nonsynonymous and (D) synonymous within-host mutation are shown on an NA crystal structure (PDB 2BAT [Varghese et al., 1992]). All sites of synonymous and nonsynonymous mutation are shown here, in contrast to Figure 4A, which only shows sites in HA at which nonsynonymous mutations arise in multiple patients in our study.
Figure 2—figure supplement 7.
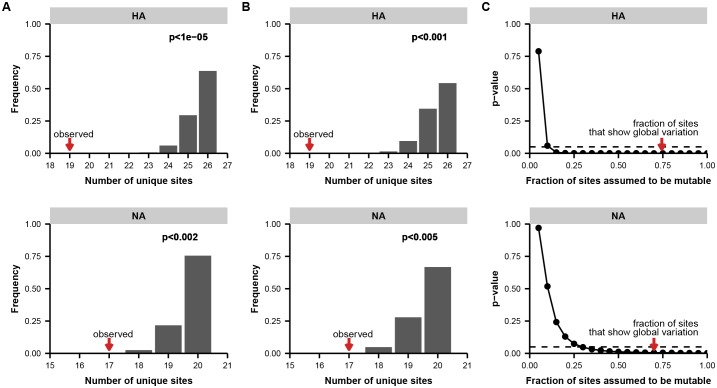
(A) Distribution of unique variable sites when sites are drawn at random along the full length of the indicated gene, matching the number of variants empirically observed in each patient. These simulations test a simple null model in which each site in the gene is equally likely to mutate. We calculated the number of unique sites of mutation in each simulation as a metric of parallelism: fewer unique sites means that more parallel mutation has occurred. The p-value indicates the proportion of 100,000 simulations in which the number of unique sites is less than or equal to what is empirically observed. (B) Distribution of overlapping sites for simulations as described in (A), but with a constrained null model in which the fraction of sites considered mutable is the fraction that shows at least two instances of nonsynonymous mutation in the global H3N2 influenza population between 2000 and 2015 (see Materials and methods) to account for constraints on protein evolution. For both HA and NA, the observed overlap of mutations between patients is statistically significant under this set of constraints. (C) p-values as described in (A), calculated across a range of constraints on the fraction of mutable sites. The constrained null model indicated in (B) is indicated with a red arrow. For both HA and NA, the observed parallelism is statistically significant at a threshold of 0.05 unless it is assumed that fewer than 15% of the sites in HA or 35% of the sites in NA are mutable.
In several cases, the same mutations arise independently and reach high frequency in multiple patients (Figure 2C). We identified nine sites in the influenza genome where parallel mutations arose in two or more patients in our study: five in HA, three in NA, and one in the nonstructural (NS) segment (Figure 2C, Figure 2—figure supplement 7; HA: p<0.001; NA: p<0.01; permutation test). In subsequent analyses, we focused primarily on HA because of its prominent role in antigenic evolution (Koel et al., 2013).
Recurrent mutations drive clonal interference within individual patients
Although the same HA mutations arise in multiple patients, we found that evolutionary outcomes sometimes diverge. For instance, A138S arises in patients W and Z, but it fixes only in patient Z. In three patients, N225D reaches a detectable frequency, but it fixes only in patient X (Figure 2C).
We suspected that the complex dynamics of these within-host mutations might arise from competition among mutant lineages. The influenza genome consists of eight linear segments that freely re-assort with one another but do not recombine (Boni et al., 2008), meaning that each segment evolves clonally. In the absence of homologous recombination, lineages carrying beneficial mutations rise and fall in frequency as they compete with one another, making it harder for any one variant to fix. This phenomenon, known as clonal interference, has been characterized extensively in experimental evolution (Hegreness et al., 2006; Kao and Sherlock, 2008; Lang et al., 2013; Neher, 2013) and affects influenza’s global evolution (Strelkowa and Lässig, 2012).
We examined clonal dynamics within individual patients by analyzing patterns of linkage among within-host mutations. We identified read pairs that spanned multiple variable sites to infer linkage, and we summarized these relationships as haplotypes: for instance, ‘0000’ represents ancestral residues at four variable sites, and ‘1100’ represents a double-mutant at the first two sites (Figure 3A).
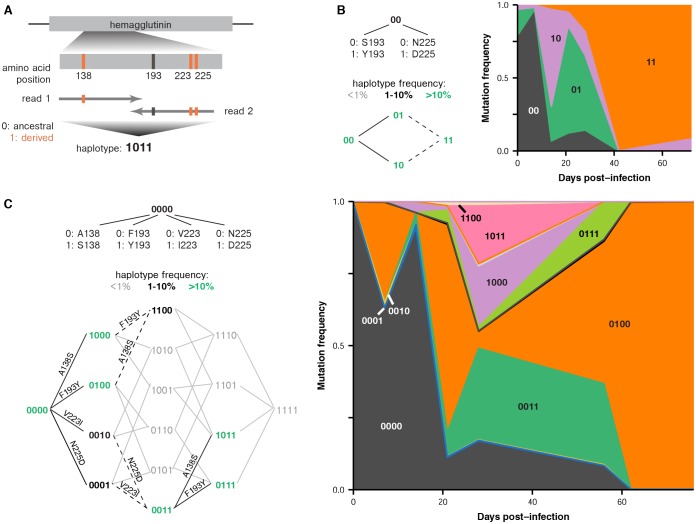
(A) Method for inferring partial haplotypes from short-read sequencing data. We identified paired-end reads that spanned multiple sites of interest along a gene and determined whether the read carried the ancestral or derived allele at each site. (B) Frequencies of haplotypes at HA sites 193 and 225 in patient X. Evolutionary paths from the ancestral to double-mutant state are shown, with haplotypes colored according to their maximum frequency during the infection. Solid black lines connect pairs of haplotypes that are both present at a frequency of above 1% and that unambiguously occurred through the indicated mutation. Dashed lines indicate that multiple mutations could have produced a particular haplotype. Gray lines indicate that a mutation did not arise at a detectable frequency on a particular haplotype background. (C) Frequencies of haplotypes at HA sites 138, 193, 223, and 225 in patient W. Figure 3—figure supplement 1 estimates the rate of PCR recombination as described in Materials and methods. Figure 3—figure supplement 2 and and33 show the number of paired-end reads that spanned the mutations in the haplotypes in patients X and W.
Figure 3—figure supplement 1.
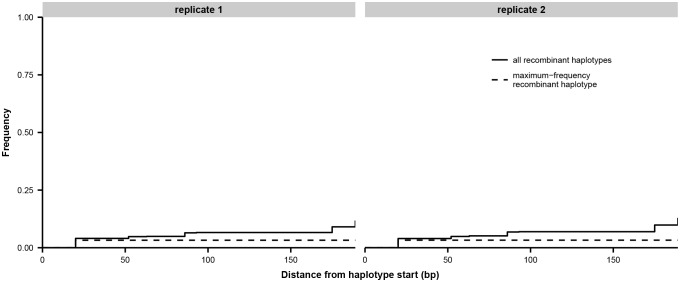
The total frequency of all recombinant haplotypes and the frequency of the single most common recombinant haplotype are shown as a function of distance from the haplotype start. We mixed equal volumes of extracted RNA from the first influenza-positive nasal washes from patients W and X and prepared replicate libraries for sequencing as described in Materials and methods. In the absence of PCR recombination, all haplotypes should consist entirely of bases from one or the other original sample: for instance, 00000000 or 11111111. Based on sequencing of the unmixed samples from patients W and X, we identified eight sites of fixed differences within a 200 bp region of the HA gene, and we inferred haplotypes in the mixture sample at these eight sites. Beginning at the first haplotype site, we tallied the proportion of haplotypes that had experienced recombination by each successive site in the haplotype. We did not seek to distinguish between PCR recombination and sequencing errors: the haplotypes 00100000 and 00111111 were both recorded as having experienced recombination by the third haplotype site. The maximum-frequency recombinant haplotype never exceeded 3.5% of the total haplotypes.
Figure 3—figure supplement 2.
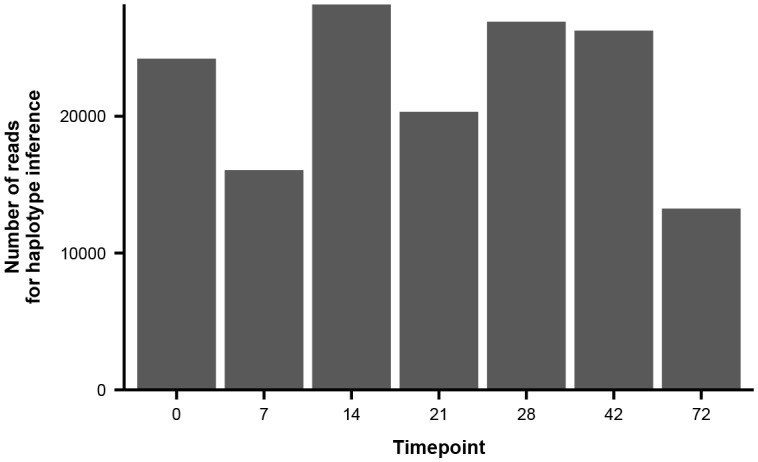
Each bar represents the average number of paired-end reads that spanned both variable sites of interest in Figure 3B across the two sequencing replicates.
Figure 3—figure supplement 3.
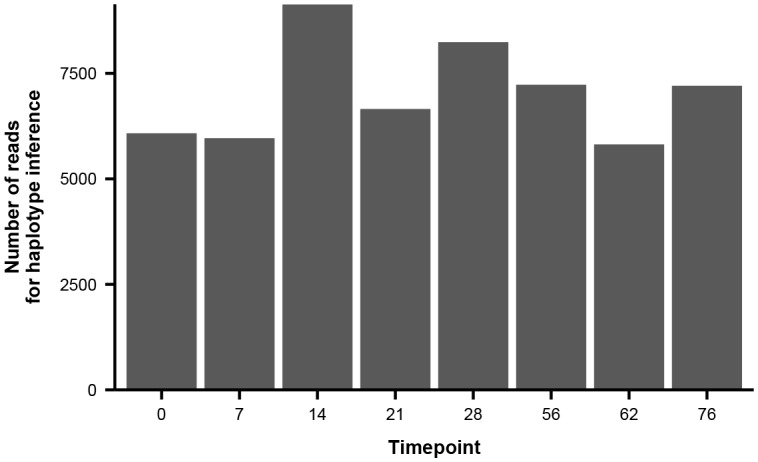
Each bar represents the average number of paired-end reads that spanned the four variable sites of interest in Figure 3C across the two sequencing replicates.
In several instances, the same mutations arise in parallel on distinct genetic backgrounds within the same patient—echoing our observation that these same mutations arise in parallel in multiple patients in our study. In patient X, lineages carrying S193Y and N225D initially compete, but a double-mutant carrying both mutations eventually fixes (Figure 3B). The A138S and F193Y mutations also arise multiple times in parallel in patient W: once on the ancestral haplotype ‘0000’ to form the single-mutant ‘1000’ and ‘0100’ lineages; once on these single-mutant lineages to form the double-mutant ‘1100’; and once on the double-mutant ‘0011’ to form the triple-mutant ‘1011’ and ‘0111’ lineages (Figure 3C). These recurrent mutations also contribute to the large number of clonal lineages present. Several weeks into patient W’s infection, we observe at least five distinct HA lineages at a frequency of at least 5%, and the lineages differ from each other by one to three nonsynonymous mutations (Figure 3C). Eventually, all lineages that carry A138S, V223I, and N225D are outcompeted by a lineage that carries F193Y.
Our analysis shows that in large, clonally evolving influenza populations within hosts, a small set of beneficial mutations repeatedly arise and compete against one another in various combinations. Although many of these beneficial mutations are selected in parallel in multiple patients, the unpredictability of clonal competition determines which mutations eventually fix.
Within-host variants often arise at sites that are polymorphic in influenza globally
We compared viral mutations that arose within our patients and at the global scale. Strikingly, many of the HA mutations that arise in parallel in multiple patients in our study also reach a high global frequency, which may reflect concordant antigenic selection at the within-host and global scales. We identified all variants that reached a frequency of at least 10% in any given year after 2000 in the GISAID database of global influenza sequences (Bogner et al., 2006) and compared them to variants that we identified in the patients in our study.
In HA, most sites that varied within hosts also varied in the global influenza population, compared to about a quarter of such sites in the other influenza genes (Figure 4). We tested whether this overlap between sites of variation within patients and globally was greater than expected by chance for HA, NA, and the rest of the viral genome combined. We calculated the expected overlap when the observed number of within-host and global variants were drawn at random from each gene (Figure 4—figure supplements 1–2). Not all sites are expected to tolerate mutation, so we also performed simulations where we only considered sites for which there was variation in human H3N2 influenza globally between 2000 and 2015: for instance, in HA about 25% of codon sites show no variation within the GISAID database. We found significant parallelism in HA (p<0.01), but not in NA or in the rest of the genome (p>0.05) when we consider all sites of global variation. This parallelism in HA evolution remains statistically significant at a 0.05 threshold until we assume that less than 50% of HA codon sites tolerate variation.
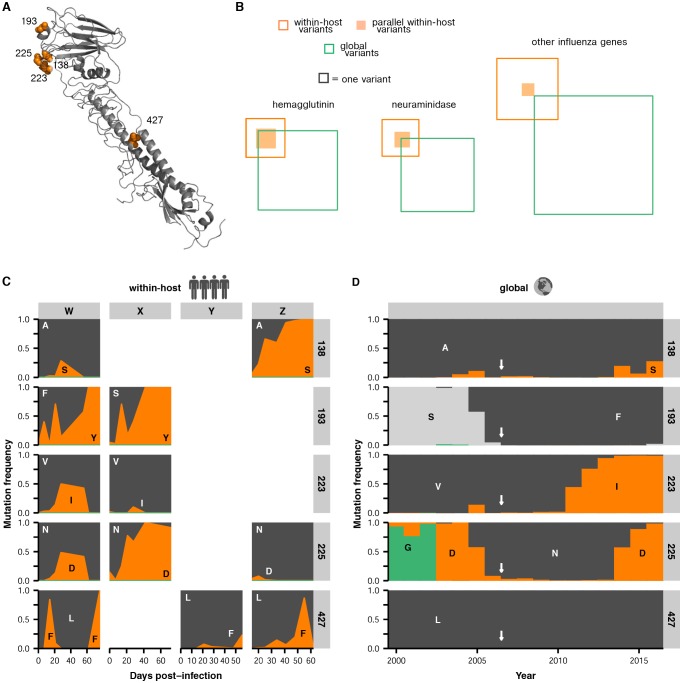
(A) Sites of parallel within-host mutation plotted on an HA crystal structure (PDB 4HMG [Weis et al., 1990]). (B) Overlap of within-host (orange) and global (green) variable sites in HA, NA, and all other influenza genes. Sites at which mutations arise in more than one patient are indicated in solid orange. We defined global variable sites as those at which a variant reached a frequency of at least 10% in a given year after 2000 in the GISAID database of global influenza sequences (Bogner et al., 2006). Numbers of within-host and global mutations are given in Figure 4—source data 1. (C) Mutation frequencies over time within individual patients for parallel within-host mutations in HA. Ancestral identities are colored in gray and mutant ones in orange. (D) Global variant frequencies between 2000 and 2015 in H3N2 influenza at sites of parallel within-host mutation in HA. The approximate timing of the patient infections (2006–2007) is indicated by a white arrow. Figure 4—figure supplement 1 displays variant frequencies for all sites of parallel mutation at the within-host and global scales. Figure 4—figure supplement 2 describes permutation tests that assess the significance of the overlap in mutations at the within-host and global scales.
DOI: http://dx.doi.org/10.7554/eLife.26875.018
Figure 4—source data 1.
Overlap of mutations at the within-host and global scales.DOI: http://dx.doi.org/10.7554/eLife.26875.019
Figure 4—figure supplement 1.
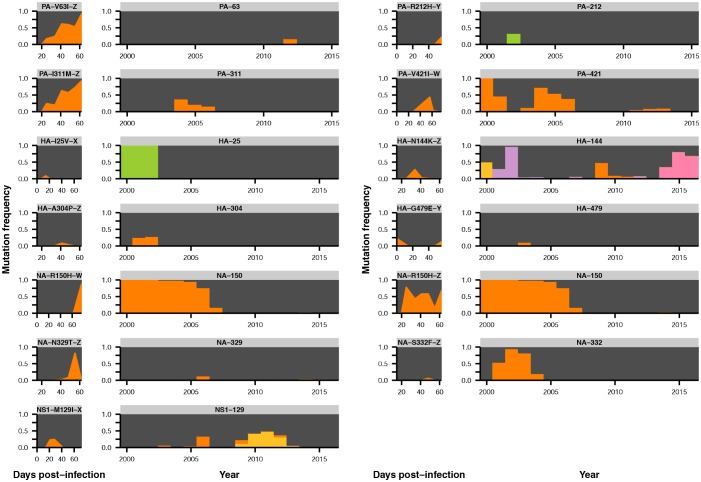
Mutation frequencies over time are plotted within hosts and in the global population for sites that are variable across both scales. Parallel within-host mutations in HA are shown in Figure 4C and are omitted. This figure shows mutation frequencies for the remaining sites that were variable across scales. Within-host mutations are labeled by gene name, amino acid change, and patient ID. Ancestral identities are colored in gray and mutant ones in orange at sites of within-host mutation, and the same colors are applied to those amino acids in the global frequency plots where present. Global variant frequencies are shown twice for NA site 150 because mutations arise at that site in two independent patients.
Figure 4—figure supplement 2.
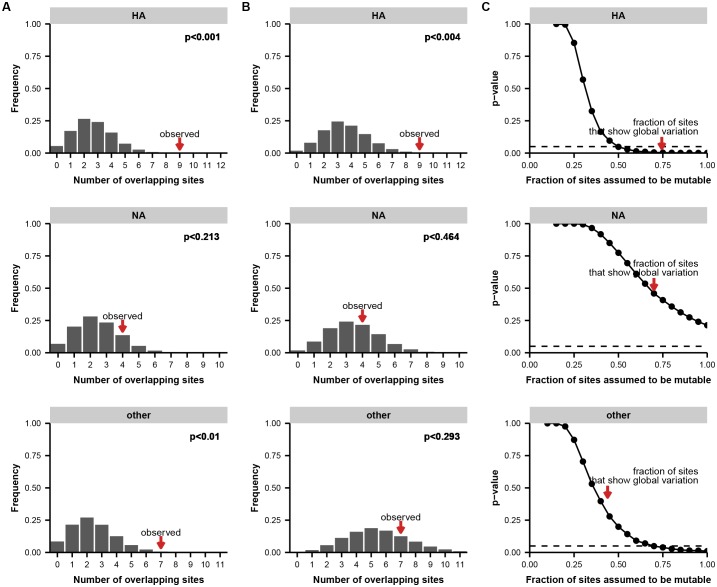
(A) Distribution of overlapping sites when two sets of sites are drawn at random along the full length of the indicated gene or genes, matching the number of unique variable sites empirically observed in each patient and in the global influenza population (see Materials and methods). These simulations test a simple null model in which each site is equally like to mutate. We calculated the overlap between the two sets of sites in each simulation as a metric of parallelism: greater overlap means that more parallelism has occurred. The p-value indicates the proportion of 100,000 simulations in which the number of overlapping sites is greater than or equal to what is empirically observed. (B) Distribution of overlapping sites for simulations as described in (A), performed with a constrained null model in which the fraction of sites considered mutable is the fraction that shows at least two instances of nonsynonymous mutation in the global H3N2 influenza population between 2000 and 2015 (see Materials and methods) to account for constraints on protein evolution. For HA, but not NA or the other influenza genes in aggregate, the observed overlap of mutations at the within-host and global scales is statistically significant under this set of constraints. (C) p-values as described in (A), calculated across a range of constraints on the fraction of mutable sites. The constrained null model indicated in (B) is indicated with a red arrow. For HA, the observed parallelism is statistically significant at a threshold of 0.05 unless it is assumed that fewer than half the sites in the protein are mutable.
The parallelism is especially striking at the sites of HA mutations found in multiple patients in our study. In particular, four of the five sites of recurrent within-host mutation in HA are also sites of global influenza variation (Figure 4, Figure 4—source data 1). The V223I and N225D mutations arise in multiple patients, and then fix globally in the decade after the patient infections (Figure 4D). Mutations also reach high global frequencies at sites 138 and 193, although the F193 and S193 variants that spread globally differ from the Y193 variant that arises within our patients. However, the concordance is incomplete. Mutation L427F reaches a frequency of >75% in three patients but is rare or nonexistent in influenza globally (Figure 4D), suggesting that this mutation may have within-host benefits that are not reflected in global evolution. But overall across hemagglutinin, within-host variants tend to arise at sites that vary on the global scale.
Discussion
It is remarkable that influenza evolution shows such extensive parallelism at these disparate spatiotemporal scales despite heterogeneity in host immunity, viral genetic background, and the severity and duration of infection. In particular, the immunocompromised patients in our study had complex underlying conditions and diverse immune histories. Notably, the four HA sites that displayed parallel within-host and global evolution in our study (138, 193, 223, and 225) also gave rise to mutations in another study that used Sanger sequencing to analyze laboratory-passaged influenza isolated longitudinally from an immunocompromised child (Baz et al., 2006). Another previous study used hemagglutination inhibition assays to show that antigenic drift of influenza within an immunocompromised patient resembled global antigenic change (McMinn et al., 1999). These similarities further support our finding that influenza evolution shows parallelism across diverse patients.
The parallel evolution that we observe in influenza at the within-host and global scales contrasts with HIV, where similar mutations can arise within hosts that share an HLA type, but tend to revert upon transmission to recipients with different HLA types (Leslie et al., 2004; Herbeck et al., 2006; Lemey et al., 2006; Zanini et al., 2015). Part of the difference may be that immune epitopes in influenza are broadly similar among individuals, with some exceptions (Li et al., 2013; Linderman et al., 2014), whereas the targets of anti-HIV immunity vary more widely due to patient-specific factors like HLA type.
We suggest that parallelism in HA evolution may emerge from the confluence of several evolutionary conditions (Lässig et al., 2017). First, if selection acts concordantly across environments, it will favor a common set of beneficial mutations. Second, in a constrained evolutionary landscape, this set of beneficial mutations will be relatively small. Finally, given sufficiently large population sizes, high mutation rates, and time, these beneficial mutations will emerge and be selected to detectable frequencies. Our observation that similar mutations arise repeatedly within single patients, within multiple different patients, and at the global scale, suggests that at least some of these conditions may hold true.
The parallelism and extensive evolution that we observe in long-term influenza infections contrasts with the limited within-host variation found in prior studies, which sample from acute infections of immunocompetent hosts (Murcia et al., 2010; Hoelzer et al., 2010; Dinis et al., 2016; Debbink et al., 2017; Sobel Leonard et al., 2016; Poon et al., 2016). For instance, one recent study deep-sequenced HA from several hundred patients but only found a small number of antigenic variants, and mostly at low frequencies (Dinis et al., 2016). But our study suggests that influenza may experience many of the same selective pressures within acute infections as it does globally, even if the short durations of these infections make it difficult for selected mutations to reach frequencies that are detectable with current methods. We suggest that within-host viral diversity may act as a noisy early measurement of global viral evolution, shaped by some of the same immunological and evolutionary constraints. As high-throughput sequencing continues to improve, detailed characterization of within-host variation will be increasingly valuable for understanding how molecular, immunological, and epidemiological forces interact to shape viral evolution.
Materials and methods
Patient material
Samples were prospectively collected during a surveillance study for respiratory viruses performed in allogeneic hematopoietic stem cell transplant (HCT) recipients undergoing transplantation between December 2005 and February 2010 at Fred Hutchinson Cancer Research Center (Campbell et al., 2015). Following written informed consent, weekly nasal wash samples (or nasopharyngeal swabs if nasal wash samples were precluded clinically) and oropharyngeal swab specimens were obtained at least once before and weekly after HCT up to 100 days. Afterwards, samples were collected as long as the patients continued to test positive for respiratory viruses, if they developed new symptoms, or at least every three months until one year post-transplantation. Nasal wash samples were collected using 5 mL of saline per nostril, and combined with oropharyngeal swabs for real-time PCR testing for a panel of 12 respiratory viruses, including influenza A and B. Samples were considered positive if the assay’s cycle threshold was less than 40, for a limit of detection of approximately 2000 viral copies/mL. All samples sequenced in this study tested positive for influenza A. The timing of each sample during an infection was calculated as the number of days since the first influenza-positive nasal wash for that patient.
Descriptions of individual patients and their clinical courses are summarized below, with detailed information in Figure 1—figure supplement 1. All patients were severely immunocompromised: although their influenza infections occurred after transplant engraftment, their lymphocyte counts remained well below those found in immunocompetent individuals, and they were concurrently treated with immunosuppressive medications. Influenza sometimes co-occurred with other respiratory viruses, and the patients were frequently taking multiple antiviral and antibiotic medications at any given point in the infection.
Patient W
A female in the 25–44 age group developed upper respiratory symptoms in early 2007, 30 days after receiving a non-myeloablative HCT for Hodgkin’s disease and 18 days following engraftment. Patient nasal wash samples repeatedly tested positive for influenza A for the next 80 days until the patient died of pulmonary failure, with diffuse alveolar damage found on autopsy. The patient received a 12 day course of oseltamivir at 75 mg PO BID approximately 30 days into the infection and was treated continuously with oseltamivir for the last 26 days of her life, first at 75 mg PO BID and then increasing to 150 mg PO BID. The patient was co-infected with coronavirus for the duration of the influenza infection and also tested positive for human metapneumovirus for the last 26 days of her life.
Patient X
A male in the 65+ age group developed upper respiratory symptoms in early 2006, 45 days after receiving a non-myeloablative HCT for Hodgkin’s disease. Patient nasal wash samples repeatedly tested positive for influenza A for the next 72 days, after which the patient chose to discontinue study participation. The patient was treated with two courses of oseltamivir: a 5 day course at 75 mg PO BID following the first positive nasal wash, and an 8 day course at 75 mg PO BID approximately four weeks into the infection. The patient also tested positive for cytomegalovirus (CMV) and Aspergillus early in the influenza infection.
Patient Y
A male in the 45–64 age group developed upper respiratory symptoms in spring 2006, 62 days after receiving a non-myeloablative HCT for acute myeloid leukemia (AML) and 52 days after engraftment. Patient nasal wash samples repeatedly tested positive for influenza A for the next 77 days, after which the patient began testing negative. The patient was treated with three courses of oseltamivir: an 8 day course following the first positive nasal wash, a 30 day course beginning approximately two weeks into the infection, and a second 30 day course starting approximately seven weeks into the infection, all at 75 mg PO BID. The patient also intermittently tested positive for CMV and coronavirus during the influenza infection.
Patient Z
A male in the 65+ age group developed upper respiratory symptoms in early 2007, 197 days after receiving a non-myeloablative HCT for AML and 175 days after engraftment. Nasal wash samples repeatedly tested positive for influenza A over the next 69 days, after which monitoring ceased due to severe illness, and the patient died 15 days after the last influenza-positive sample from relapsed AML. The patient was treated with two courses of oseltamivir: a 6 day course at 150 mg PO BID following the first flu-positive nasal wash, and a 66 day course starting approximately two weeks into the infection that began at 150 mg PO QD and increased to 150 mg PO BID. The patient also received 30 g of IVIG 46 days into the flu infection. The patient intermittently tested positive for respiratory syncytial virus over the same period and also experienced Epstein-Barr viremia.
Viral deep sequencing
To deep-sequence viral populations, we extracted bulk RNA from nasal wash samples using the QIAamp Viral RNA Mini Kit (QIAGEN) according to manufacturer’s instructions. Where possible, we extracted RNA from 560 μL of sample, the maximum volume recommended for use with the QIAamp kit, to capture as much viral diversity as possible.
To amplify the influenza genome, we modified the primers designed by Hoffmann et al. (2001) for full-length amplification of the influenza A genome (Figure 2—source data 1). We performed reverse transcription using Superscript III First-Strand Reaction Mix (Thermo Fisher) and an equimolar mix of the 5’-Hoffmann-U12-A4 and 5’-Hoffmann-U12-G4 primers, which bind to the conserved U12 region present on each influenza gene segment. To 6 μL RNA eluent, we added 1 μL annealing buffer and 1 μL of 2 uM primer mix, then incubated at 65 degrees C for 5 min. We added 10 μL 2X First-Strand Reaction Mix and 2 μL Superscript III/RNaseOUT Enzyme Mix on ice for a 20 μL total reaction volume, then incubated at 25 degrees C for 10 min (this initial incubation is designed to help with the binding of short primers), 50 degrees C for 50 min, and 85 degrees C for 5 min.
We used the entire 20 μL volume of the reverse-transcription reaction as template in a 100 μL PCR reaction using KOD HotStart Reaction Mix (EMP Millipore) and a 24-primer cocktail as described in Figure 2—source data 1 at a total concentration of 600 nM. We performed 35 cycles of PCR amplification with an annealing temperature of 55 degrees C and an extension time of 3 min.
We purified the PCR product using 1X AMPure beads (Beckman Coulter) and prepared libraries for Illumina sequencing using Nextera XT (Illumina). We sequenced the libraries on a NextSeq 500 platform (Illumina) with 150 bp paired-end reads. We performed library preparation and sequencing in duplicate, starting from independent reverse-transcription reactions.
Read mapping
We first used bowtie2 (Langmead and Salzberg, 2012) to filter out reads that mapped to the human genome. Remaining reads are available in the SRA as BioProject PRJNA364676. We used cutadapt 1.8.3 (Martin, 2011) to trim adapter sequences from the remaining reads, remove bases at the ends of reads with a Q-score below 25, and filter out reads whose remaining length was shorter than 20 bases. We locally aligned trimmed reads to the A/Brisbane/10/2007 (H3N2) genome (Genbank accessions CY035022 to CY035029) using bowtie2 (Langmead and Salzberg, 2012) and tallied the counts of each base at each genome position using custom scripts. We discarded reads with a mapping score below 20, as well as bases with a Q-score below 20.
Quality filtering
We calculated average sequencing coverage in 50 bp bins along the viral genome. Because we prepared sequencing libraries using Nextera tagmentation, we expect coverage to be low at the two ends of the eight viral gene segments, corresponding to 16 bins. We discarded samples with more than 16 bins with average coverage below 200x (Figure 2—figure supplement 1A). We also identified sites at which a non-consensus base reached a frequency of at least 1% in both sequencing replicates and compared variant frequencies between replicates. We discarded samples for which the average difference between variant frequencies in the two replicates exceeded 0.05 (Figure 2—figure supplement 1B). In total, we excluded eight samples from downstream analyses. The samples shown in Figure 1B are high-quality samples only.
Variant calling and annotation
For each patient, we identified variable nucleotide sites in the viral genome. We defined these sites as positions with a sequencing coverage of at least 200x, at which multiple bases are present at a frequency of at least 5% in both replicate libraries. We used custom scripts to determine each variant’s codon position and whether it created a synonymous or nonsynonymous substitution.
A note on codon numbering and gene annotation
We numbered HA codons according to the H3 numbering system. This HA numbering scheme assigns 1 to codon 17 of the full HA gene, which is the beginning of the mature HA protein. The codons for all other genes are numbered sequentially beginning with one at the N-terminal methionine. The M1 and M2 genes have 27 bp of in-frame and 44 bp of out-of-frame overlap, and the NS1 and NEP genes have 30 bp of in-frame and 251 bp of out-of-frame overlap. We annotated variants separately for each gene if they occurred in these regions of overlap.
Phylogenetic analysis
For each patient in our study, we determined the viral consensus sequence at the first sequenced time point. We also downloaded the set of 503 sequences in the Global Initiative on Sharing All Influenza Data (GISAID) EpiFlu database (Bogner et al., 2006) corresponding to all full-length HA coding regions from human H3N2 influenza A isolates collected in the USA from January 1, 2004 to December 31, 2007 (GISAID acknowledgement tables provided in Supplementary file 1). We analyzed only sequences with passage annotation ‘Unpassaged,’ ‘Original’, or ‘P0,’ indicating that the strains were sequenced directly from the clinical isolates, leaving 63 unique sequences for phylogenetic inference. We pairwise aligned each sequence to the A/Brisbane/10/2007 (H3N2) coding sequence (Genbank accession CY035022) using the program needle from EMBOSS 6.6.0 (Rice et al., 2000), which implements a Needleman-Wunsch alignment. We used RAxML 8.2.3 (Stamatakis, 2014) to infer a phylogeny from this alignment using a GTRCAT codon-substitution model and visualized the tree using the R package ggtree (Yu et al., 2017).
Haplotype inference
We identified paired-end reads that spanned n variable sites of interest within a single gene and determined which bases were present at each variable site. We summarized this information as an n-digit binary haplotype, in which each digit represented one variable site, 0 represented the ancestral base, and 1 represented the derived base. We discarded reads that did not span all sites of interest, or that contained genotypes other than the most common derived base. We estimated the rate of PCR recombination as described in Figure 3—figure supplement 1. In Figure 3—figure supplement 2 and Figure 3—figure supplement 3, we show the number of paired-end reads used to infer the haplotypes in Figure 3.
Analysis of global variation
To identify sites of global variation in influenza, we downloaded all sequences in the Global Initiative on Sharing All Influenza Data (GISAID) EpiFlu database (Bogner et al., 2006) corresponding to all full-length influenza coding regions from human H3N2 influenza A isolates collected from January 1, 2000 to December 31, 2015. Acknowledgement tables are provided as Supplementary file 1. We pairwise aligned each sequence to the A/Brisbane/10/2007 (H3N2) coding sequence (Genbank accession CY035022) using the program needle from EMBOSS 6.6.0 (Rice et al., 2000), which implements a Needleman-Wunsch alignment. We calculated the amino-acid distance of each sequence from the Brisbane/2007 reference and excluded outliers whose distance deviated significantly from the other sequences originating from that year, since these sequences may have been misannotated. We tallied the amino acids present at each codon position in each year, discarding sequences that contained indels, and we identified sites at which multiple amino acids were present at a frequency of at least 10% within a single year, or at which the consensus base changed from year to year.
Statistical tests of parallelism
We sought to test the probability that the parallel emergence of mutations across multiple patients in our study was due to chance. We began with a simple null model in which all sites are equally likely to mutate, and we drew sites from each gene at random without replacement, matching the number of mutations observed in each patient. We calculated the number of unique sites of mutation among all four patients in this simulated data set, and we compared this distribution to the number of unique sites observed in our sequencing data: fewer unique sites of mutation indicates more parallelism (Figure 2—figure supplement 7A). This null model is overly simplistic, since some sites in a protein experience more evolutionary constraint. To estimate this constraint, we limited the number of sites considered mutable to the sites that show at least two instances of nonsynonymous mutation in the global H3N2 population between 2000 and 2015 (see Analysis of Global Variation) (Figure 2—figure supplement 7B). The p-values given in the main text are calculated under this more conservative null model. We also performed permutation tests for a range of possible proportions of mutable sites and calculated the fraction of simulations that matched or exceeded the amount of parallelism observed in our data (Figure 2—figure supplement 7C).
We used a similar approach to test whether the overlap of mutations observed within patients in our study and in the global flu population was likely to be due to chance. We drew two independent sets of sites from each gene at random without replacement, matching the total number of unique variable sites within all patients and the number of variable sites observed in the global population. We then calculated the overlap between these two sets of sites. We used the approach above to calculate the overlap under a simple null model in which all sites in the gene are equally like to mutate; a constrained null model in which the only mutable sites are ones that show nonsynonymous mutation between 2000 and 2015; and across a range of possible constraints (Figure 4—figure supplement 2).
Data and code availability
The FASTQ files are available on the SRA as BioProject PRJNA364676. The computer code that performs the analysis is available at https://github.com/ksxue/parallel-evolution (with a copy archived at https://github.com/elifesciences-publications/parallel-evolution) and in Supplementary file 2 (Xue, 2017).
Acknowledgements
We thank Choli Lee and Seungsoo Kim for assistance with sequencing; Darneshia Smith for sample management; Louise Kimball and Alpana Waghmare for interpretation of patient clinical data; and Seungsoo Kim, Alexander Greninger, and Trevor Bedford for comments and discussion about the manuscript. We also thank Thomas Friedrich, Louise Moncla, and Nick Florek for helpful discussions about methods for deep-sequencing and Mike Famulare for helpful discussions about evolution at the within- and between-host scales.
Funding Statement
The funders had no role in study design, data collection and interpretation, or the decision to submit the work for publication.
Funding Information
This paper was supported by the following grants:
National Institute of General Medical Sciences R01GM102198 to Jesse D Bloom.
National Institute of Allergy and Infectious Diseases R01AI127893 to Jesse D Bloom.
National Heart, Lung, and Blood Institute R01HL081595 to Michael Boeckh.
Howard Hughes Medical Institute Faculty Scholar Award to Jesse D Bloom.
Simons Foundation Faculty Scholar Award to Jesse D Bloom.
National Science Foundation DGE-1256082 to Katherine S Xue.
Hertz Foundation Hertz Graduate Fellowship to Katherine S Xue.
National Heart, Lung, and Blood Institute K24HL093294 to Michael Boeckh.
National Heart, Lung, and Blood Institute K23HL091059 to Angela P Campbell.
Additional information
Competing interests
JAE: JAE reports research support from Gilead Sciences, GlaxoSmithKline, Chimerix, and Pfizer, and fees for participation in a Data Safety Monitoring Board for GlaxoSmithKline.
MB: MB reports research support and consulting fees from Aviragen Therapeutics, Gilead Sciences, and Ansun BioPharma, and research support from GlaxoSmithKline.
The other authors declare that no competing interests exist.
Author contributions
KSX, Conceptualization, Software, Investigation, Visualization, Methodology, Writing—original draft, Writing—review and editing.
TS-A, Resources, Data curation, Writing—review and editing.
APC, Resources, Data curation, Project administration, Writing—review and editing.
JAE, Resources, Data curation, Funding acquisition, Project administration, Writing—review and editing.
SAP, Conceptualization, Resources, Data curation, Project administration, Writing—review and editing.
MB, Resources, Data curation, Funding acquisition, Project administration.
JDB, Conceptualization, Supervision, Funding acquisition, Writing—original draft, Writing—review and editing.
Ethics
Human subjects: Samples were prospectively collected during a surveillance study for respiratory viruses performed in allogeneic hematopoietic stem cell transplant (HCT) recipients undergoing transplantation between December 2005 and February 2010 at Fred Hutchinson Cancer Research Center (Campbell et al. 2015). Following written informed consent, weekly nasal wash samples (or nasopharyngeal swabs if nasal wash samples were precluded clinically) and oropharyngeal swab specimens were obtained at least once before and weekly after HCT up to 100 days. Afterwards, samples were collected as long as the patients continued to test positive for respiratory viruses, if they developed new symptoms, or at least every three months until one year post-transplantation.
Additional files
Supplementary file 1.
GISAID acknowledgement tables for global influenza sequences.DOI: http://dx.doi.org/10.7554/eLife.26875.022
Supplementary file 2.
Code and source data files for all analyses.DOI: http://dx.doi.org/10.7554/eLife.26875.023
Major datasets
The following dataset was generated:
Xue KS,Bloom JD,2017,Deep sequencing data,http://www.ncbi.nlm.nih.gov/bioproject/PRJNA364676,Publicly available at the NCBI BioProject database (accession no: PRJNA364676)
References
- Andino R, Domingo E. Viral quasispecies. Virology. 2015;479-480:46–51. 10.1016/j.virol.2015.03.022. [Europe PMC free article] [Abstract] [CrossRef] [Google Scholar]
- Baz M, Abed Y, McDonald J, Boivin G. Characterization of multidrug-resistant influenza A/H3N2 viruses shed during 1 year by an immunocompromised child. Clinical Infectious Diseases. 2006;43:1555–1561. 10.1086/508777. [Abstract] [CrossRef] [Google Scholar]
- Bhatt S, Holmes EC, Pybus OG. The genomic rate of molecular adaptation of the human influenza A virus. Molecular Biology and Evolution. 2011;28:2443–2451. 10.1093/molbev/msr044. [Europe PMC free article] [Abstract] [CrossRef] [Google Scholar]
- Bogner P, Capua I, Cox NJ, Lipman DJ. A global initiative on sharing avian flu data. Nature. 2006;442:981. 10.1038/442981a. [CrossRef] [Google Scholar]
- Boni MF, Zhou Y, Taubenberger JK, Holmes EC. Homologous recombination is very rare or absent in human influenza A virus. Journal of Virology. 2008;82:4807–4811. 10.1128/JVI.02683-07. [Europe PMC free article] [Abstract] [CrossRef] [Google Scholar]
- Campbell AP, Guthrie KA, Englund JA, Farney RM, Minerich EL, Kuypers J, Corey L, Boeckh M. Clinical outcomes associated with respiratory virus detection before allogeneic hematopoietic stem cell transplant. Clinical Infectious Diseases. 2015;61202:192–202. 10.1093/cid/civ272. [Europe PMC free article] [Abstract] [CrossRef] [Google Scholar]
- Debbink K, McCrone JT, Petrie JG, Truscon R, Johnson E, Mantlo EK, Monto AS, Lauring AS. Vaccination has minimal impact on the intrahost diversity of H3N2 influenza viruses. PLOS Pathogens. 2017;13:e1006194. 10.1371/journal.ppat.1006194. [Europe PMC free article] [Abstract] [CrossRef] [Google Scholar]
- Dinis JM, Florek NW, Fatola OO, Moncla LH, Mutschler JP, Charlier OK, Meece JK, Belongia EA, Friedrich TC. Deep sequencing reveals potential antigenic variants at low frequencies in Influenza A Virus-Infected humans. Journal of Virology. 2016;90:3355–3365. 10.1128/JVI.03248-15. [Europe PMC free article] [Abstract] [CrossRef] [Google Scholar]
- Ghedin E, Sengamalay NA, Shumway M, Zaborsky J, Feldblyum T, Subbu V, Spiro DJ, Sitz J, Koo H, Bolotov P, Dernovoy D, Tatusova T, Bao Y, St George K, Taylor J, Lipman DJ, Fraser CM, Taubenberger JK, Salzberg SL. Large-scale sequencing of human influenza reveals the dynamic nature of viral genome evolution. Nature. 2005;437:1162–1166. 10.1038/nature04239. [Abstract] [CrossRef] [Google Scholar]
- Grenfell BT, Pybus OG, Gog JR, Wood JL, Daly JM, Mumford JA, Holmes EC. Unifying the epidemiological and evolutionary dynamics of pathogens. Science. 2004;303:327–332. 10.1126/science.1090727. [Abstract] [CrossRef] [Google Scholar]
- Hegreness M, Shoresh N, Hartl D, Kishony R. An equivalence principle for the incorporation of favorable mutations in asexual populations. Science. 2006;311:1615–1617. 10.1126/science.1122469. [Abstract] [CrossRef] [Google Scholar]
- Herbeck JT, Nickle DC, Learn GH, Gottlieb GS, Curlin ME, Heath L, Mullins JI. Human immunodeficiency virus type 1 env evolves toward ancestral states upon transmission to a new host. Journal of Virology. American Society for Microbiology. 2006;80:1637–1644. 10.1128/JVI.80.4.1637-1644.2006. [Europe PMC free article] [Abstract] [CrossRef] [Google Scholar]
- Hoelzer K, Murcia PR, Baillie GJ, Wood JL, Metzger SM, Osterrieder N, Dubovi EJ, Holmes EC, Parrish CR. Intrahost evolutionary dynamics of canine influenza virus in naive and partially immune dogs. Journal of Virology. 2010;84:5329–5335. 10.1128/JVI.02469-09. [Europe PMC free article] [Abstract] [CrossRef] [Google Scholar]
- Hoffmann E, Stech J, Guan Y, Webster RG, Perez DR. Universal primer set for the full-length amplification of all influenza A viruses. Archives of Virology. 2001;146:2275–2289. 10.1007/s007050170002. [Abstract] [CrossRef] [Google Scholar]
- Kao KC, Sherlock G. Molecular characterization of clonal interference during adaptive evolution in asexual populations of Saccharomyces cerevisiae. Nature Genetics. 2008;40:1499–1504. 10.1038/ng.280. [Europe PMC free article] [Abstract] [CrossRef] [Google Scholar]
- Koel BF, Burke DF, Bestebroer TM, van der Vliet S, Zondag GC, Vervaet G, Skepner E, Lewis NS, Spronken MI, Russell CA, Eropkin MY, Hurt AC, Barr IG, de Jong JC, Rimmelzwaan GF, Osterhaus AD, Fouchier RA, Smith DJ. Substitutions near the receptor binding site determine Major antigenic change during influenza virus evolution. Science. 2013;342:976–979. 10.1126/science.1244730. [Abstract] [CrossRef] [Google Scholar]
- Lang GI, Rice DP, Hickman MJ, Sodergren E, Weinstock GM, Botstein D, Desai MM. Pervasive genetic hitchhiking and clonal interference in forty evolving yeast populations. Nature. 2013;500:571–574. 10.1038/nature12344. [Europe PMC free article] [Abstract] [CrossRef] [Google Scholar]
- Langmead B, Salzberg SL. Fast gapped-read alignment with bowtie 2. Nature Methods. 2012;9:357–359. 10.1038/nmeth.1923. [Europe PMC free article] [Abstract] [CrossRef] [Google Scholar]
- Lemey P, Rambaut A, Pybus OG. HIV evolutionary dynamics within and among hosts. AIDS Reviews. 2006;8:125–140. [Abstract] [Google Scholar]
- Leslie AJ, Pfafferott KJ, Chetty P, Draenert R, Addo MM, Feeney M, Tang Y, Holmes EC, Allen T, Prado JG, Altfeld M, Brander C, Dixon C, Ramduth D, Jeena P, Thomas SA, St John A, Roach TA, Kupfer B, Luzzi G, Edwards A, Taylor G, Lyall H, Tudor-Williams G, Novelli V, Martinez-Picado J, Kiepiela P, Walker BD, Goulder PJ. HIV evolution: ctl escape mutation and reversion after transmission. Nature Medicine. 2004;10:282–289. 10.1038/nm992. [Abstract] [CrossRef] [Google Scholar]
- Li Y, Myers JL, Bostick DL, Sullivan CB, Madara J, Linderman SL, Liu Q, Carter DM, Wrammert J, Esposito S, Principi N, Plotkin JB, Ross TM, Ahmed R, Wilson PC, Hensley SE. Immune history shapes specificity of pandemic H1N1 influenza antibody responses. The Journal of Experimental Medicine. 2013;210:1493–1500. 10.1084/jem.20130212. http://jem.rupress.org/content/210/8/1493 [Europe PMC free article] [Abstract] [CrossRef] [Google Scholar]
- Linderman SL, Chambers BS, Zost SJ, Parkhouse K, Li Y, Herrmann C, Ellebedy AH, Carter DM, Andrews SF, Zheng NY, Huang M, Huang Y, Strauss D, Shaz BH, Hodinka RL, Reyes-Terán G, Ross TM, Wilson PC, Ahmed R, Bloom JD, Hensley SE. Potential antigenic explanation for atypical H1N1 infections among middle-aged adults during the 2013-2014 influenza season. PNAS. 2014;111:15798–15803. 10.1073/pnas.1409171111. [Europe PMC free article] [Abstract] [CrossRef] [Google Scholar]
- Luksza M, Lässig M. A predictive fitness model for influenza. Nature. 2014;507:57–61. 10.1038/nature13087. [Abstract] [CrossRef] [Google Scholar]
- Lässig M, Mustonen V, Walczak AM. Predicting evolution. Nature Ecology & Evolution. 2017;1:0077. 10.1038/s41559-017-0077. [Abstract] [CrossRef] [Google Scholar]
- Martin M. Cutadapt removes adapter sequences from high-throughput sequencing reads. EMBnet.journal. 2011;17:10. 10.14806/ej.17.1.200. [CrossRef] [Google Scholar]
- McCrone JT, Lauring AS. Measurements of Intrahost viral diversity are extremely sensitive to systematic errors in variant calling. Journal of Virology. 2016;90:6884–6895. 10.1128/JVI.00667-16. [Europe PMC free article] [Abstract] [CrossRef] [Google Scholar]
- McMinn P, Carrello A, Cole C, Baker D, Hampson A. Antigenic drift of influenza A (H3N2) virus in a persistently infected immunocompromised host is similar to that occurring in the community. Clinical Infectious Diseases. 1999;29:456–458. 10.1086/520243. [Abstract] [CrossRef] [Google Scholar]
- Memoli MJ, Athota R, Reed S, Czajkowski L, Bristol T, Proudfoot K, Hagey R, Voell J, Fiorentino C, Ademposi A, Shoham S, Taubenberger JK. The natural history of influenza infection in the severely immunocompromised vs nonimmunocompromised hosts. Clinical Infectious Diseases. 2014;58:214–224. 10.1093/cid/cit725. [Europe PMC free article] [Abstract] [CrossRef] [Google Scholar]
- Murcia PR, Baillie GJ, Daly J, Elton D, Jervis C, Mumford JA, Newton R, Parrish CR, Hoelzer K, Dougan G, Parkhill J, Lennard N, Ormond D, Moule S, Whitwham A, McCauley JW, McKinley TJ, Holmes EC, Grenfell BT, Wood JL. Intra- and interhost evolutionary dynamics of equine influenza virus. Journal of Virology. 2010;84:6943–6954. 10.1128/JVI.00112-10. [Europe PMC free article] [Abstract] [CrossRef] [Google Scholar]
- Neher RA. Genetic draft, selective interference, and population genetics of rapid adaptation. Annual Review of Ecology, Evolution, and Systematics. 2013;44:195–215. 10.1146/annurev-ecolsys-110512-135920. [CrossRef] [Google Scholar]
- Neher RA, Russell CA, Shraiman BI. Predicting evolution from the shape of genealogical trees. eLife. 2014;3:e03568. 10.7554/eLife.03568. [Europe PMC free article] [Abstract] [CrossRef] [Google Scholar]
- Neher RA, Bedford T, Daniels RS, Russell CA, Shraiman BI. Prediction, dynamics, and visualization of antigenic phenotypes of seasonal influenza viruses. PNAS. 2016;113:E1701–E1709. 10.1073/pnas.1525578113. [Europe PMC free article] [Abstract] [CrossRef] [Google Scholar]
- Nichols WG, Guthrie KA, Corey L, Boeckh M. Influenza infections after hematopoietic stem cell transplantation: risk factors, mortality, and the effect of antiviral therapy. Clinical Infectious Diseases. 2004;39:1300–1306. 10.1086/425004. [Abstract] [CrossRef] [Google Scholar]
- Poon LL, Song T, Rosenfeld R, Lin X, Rogers MB, Zhou B, Sebra R, Halpin RA, Guan Y, Twaddle A, DePasse JV, Stockwell TB, Wentworth DE, Holmes EC, Greenbaum B, Peiris JS, Cowling BJ, Ghedin E. Quantifying influenza virus diversity and transmission in humans. Nature Genetics. 2016;48200:195. 10.1038/ng.3479. [Europe PMC free article] [Abstract] [CrossRef] [Google Scholar]
- Pybus OG, Rambaut A. Evolutionary analysis of the dynamics of viral infectious disease. Nature Reviews Genetics. 2009;10:540–550. 10.1038/nrg2583. [Europe PMC free article] [Abstract] [CrossRef] [Google Scholar]
- Rambaut A, Pybus OG, Nelson MI, Viboud C, Taubenberger JK, Holmes EC. The genomic and epidemiological dynamics of human influenza A virus. Nature. 2008;453:615–619. 10.1038/nature06945. [Europe PMC free article] [Abstract] [CrossRef] [Google Scholar]
- Renaud C, Kuypers J, Englund JA. Emerging oseltamivir resistance in seasonal and pandemic influenza A/H1N1. Journal of Clinical Virology. 2011;52:70–78. 10.1016/j.jcv.2011.05.019. [Abstract] [CrossRef] [Google Scholar]
- Rice P, Longden I, Bleasby A. EMBOSS: the european molecular Biology Open Software Suite. Trends in Genetics. 2000;16:276–277. 10.1016/S0168-9525(00)02024-2. [Abstract] [CrossRef] [Google Scholar]
- Rocha E, Cox NJ, Black RA, Harmon MW, Harrison CJ, Kendal AP. Antigenic and genetic variation in influenza A (H1N1) virus isolates recovered from a persistently infected immunodeficient child. Journal of Virology. 1991;65:2340–2350. [Europe PMC free article] [Abstract] [Google Scholar]
- Rogers MB, Song T, Sebra R, Greenbaum BD, Hamelin ME, Fitch A, Twaddle A, Cui L, Holmes EC, Boivin G, Ghedin E. Intrahost dynamics of antiviral resistance in influenza A virus reflect complex patterns of segment linkage, reassortment, and natural selection. mBio. 2015;6:e02464. 10.1128/mBio.02464-14. [Europe PMC free article] [Abstract] [CrossRef] [Google Scholar]
- Sobel Leonard A, McClain MT, Smith GJ, Wentworth DE, Halpin RA, Lin X, Ransier A, Stockwell TB, Das SR, Gilbert AS, Lambkin-Williams R, Ginsburg GS, Woods CW, Koelle K. Deep sequencing of Influenza A virus from a human challenge study reveals a selective bottleneck and only limited Intrahost Genetic diversification. Journal of Virology. 2016;90:11247–11258. 10.1128/JVI.01657-16. [Europe PMC free article] [Abstract] [CrossRef] [Google Scholar]
- Stamatakis A. RAxML version 8: a tool for phylogenetic analysis and post-analysis of large phylogenies. Bioinformatics. 2014;30:1312–1313. 10.1093/bioinformatics/btu033. [Europe PMC free article] [Abstract] [CrossRef] [Google Scholar]
- Strelkowa N, Lässig M. Clonal interference in the evolution of influenza. Genetics. 2012;192:671–682. 10.1534/genetics.112.143396. [Europe PMC free article] [Abstract] [CrossRef] [Google Scholar]
- Varble A, Albrecht RA, Backes S, Crumiller M, Bouvier NM, Sachs D, García-Sastre A, tenOever BR. Influenza A virus transmission bottlenecks are defined by infection route and recipient host. Cell Host & Microbe. 2014;16:691–700. 10.1016/j.chom.2014.09.020. [Europe PMC free article] [Abstract] [CrossRef] [Google Scholar]
- Varghese JN, McKimm-Breschkin JL, Caldwell JB, Kortt AA, Colman PM. The structure of the complex between influenza virus neuraminidase and sialic acid, the viral receptor. Proteins: Structure, Function, and Genetics. 1992;14:327–332. 10.1002/prot.340140302. [Abstract] [CrossRef] [Google Scholar]
- Weis WI, Brünger AT, Skehel JJ, Wiley DC. Refinement of the influenza virus hemagglutinin by simulated annealing. Journal of Molecular Biology. 1990;212:737–761. 10.1016/0022-2836(90)90234-D. [Abstract] [CrossRef] [Google Scholar]
- Xue K. parallel-evolution. e0c72033d077036289fdb0bf3e7c083bab851cc8https://github.com/ksxue/parallel-evolution Github. 2017
- Yu G, Smith DK, Zhu H, Guan Y, Lam TT-Y. ggtree: an r package forvisualization and annotation of phylogenetic trees with theircovariates and otherassociated data. Methods in Ecology and Evolution. 2017;8:28–36. 10.1111/2041-210X.12628. [CrossRef] [Google Scholar]
- Zanini F, Brodin J, Thebo L, Lanz C, Bratt G, Albert J, Neher RA. Population genomics of intrapatient HIV-1 evolution. eLife. 2015;4: e11282. 10.7554/eLife.11282. [Europe PMC free article] [Abstract] [CrossRef] [Google Scholar]
- van der Vries E, Schutten M, Fraaij P, Boucher C, Osterhaus A. Influenza virus resistance to antiviral therapy. Advances in Pharmacology. 2013;67:217–246. 10.1016/B978-0-12-405880-4.00006-8. [Abstract] [CrossRef] [Google Scholar]
Decision letter
Thank you for submitting your article "Parallel evolution of influenza across multiple spatiotemporal scales" for consideration by eLife. Your article has been favorably evaluated by Patricia Wittkopp (Senior Editor) and two reviewers, one of whom, Richard A Neher (Reviewer #1), is a member of our Board of Reviewing Editors.
The reviewers have discussed the reviews with one another and the Reviewing Editor has drafted this decision to help you prepare a revised submission.
Summary:
The reviewers agreed that you present a high-quality data set that quantifies and tracks within host evolution of influenza A virus at unprecedented detail. Similar variants are observed in viral populations in different immuno-compromised individuals and the variable sites have unexpected overlap with polymorphic sites in the global epidemic. This raises the possibility that mutation observed within host can inform predictions of influenza strains dominating future seasons. These are important observations.
Essential revisions:
However, before we can recommend your manuscript for publication in eLife, we would like you to address the following points.
1) Be careful and explicit about what can be concluded from the observed parallelism of mutations. The statement "Influenza evolution within single infected hosts acts as a microcosm of viral evolutionary dynamics observed at the global scale" overstates the degree to which global influenza evolution is predicted by within host patterns. In our interpretation, your data suggest that mutations observed within host in HA are enriched for mutations that are polymorphic later in the global epidemic. Beyond that, quite some uncertainty remains. Fixation within host does not imply fixation globally (site 138), and a site observed transiently within host (site 223) might fix globally. The statement that "most" recurrent mutations have the same within host/global dynamics (subsection “Within-host evolution often parallels global evolutionary dynamics”, last paragraph) is not supported by your data and this needs to be toned down. The degree of parallelism is remarkable, but you should be careful not to overstate it.
2) You use sequences from GISAID. Hence you need to provide a table acknowledging the GISAID contributors (or use only public domain sequences).
3) Thank you for providing the analysis code. It would be useful to if you could check this code into a git repo (e.g. on github) that eLife can then clone. A top-level README that explains the content of the different folders and its relation to the paper would also help.
4) Please extend the discussion of the statistical tests for parallelism in the main text (subsection “Within-host evolution often parallels global evolutionary dynamics”, first paragraph) to give the reader a better idea of what is being tested. Please also discuss the fact that significant enrichment is only observed when at least half of all amino acids in HA are assumed mutable.
Author response
Essential revisions:
However, before we can recommend your manuscript for publication in eLife, we would like you to address the following points.
1) Be careful and explicit about what can be concluded from the observed parallelism of mutations. The statement "Influenza evolution within single infected hosts acts as a microcosm of viral evolutionary dynamics observed at the global scale" overstates the degree to which global influenza evolution is predicted by within host patterns. In our interpretation, your data suggest that mutations observed within host in HA are enriched for mutations that are polymorphic later in the global epidemic. Beyond that, quite some uncertainty remains. Fixation within host does not imply fixation globally (site 138), and a site observed transiently within host (site 223) might fix globally. The statement that "most" recurrent mutations have the same within host/global dynamics (subsection “Within-host evolution often parallels global evolutionary dynamics”, last paragraph) is not supported by your data and this needs to be toned down. The degree of parallelism is remarkable, but you should be careful not to overstate it.
We appreciate the suggestions to add greater specificity to our claims, and we have made several textual revisions in response. We have revised our original impact statement from "Influenza evolution within single infected hosts acts as a microcosm of viral evolutionary dynamics observed at the global scale” to “Influenza evolution within infected hosts recapitulates many evolutionary dynamics observed at the global scale,” a more specific statement from our Abstract.
In the Results section, we have changed one of the section titles to state that “Within-host variants frequently arise at sites that are polymorphic in influenza globally.” We have also removed the statement that “within-host evolution can sometimes be a microcosm of global evolution” in favor of more specific language about the fixation (or lack thereof) of the specific mutations that the reviewers point out. We also specifically highlight certain differences at the within- and between-host scales like the mutation Y193, which arises at a globally variable site but is never itself observed on at a global scale.
2) You use sequences from GISAID. Hence you need to provide a table acknowledging the GISAID contributors (or use only public domain sequences).
We have added tables acknowledging the GISAID contributors to the Github repository for this manuscript: https://github.com/ksxue/parallel-evolution/tree/master/acknowledgements We have also included these tables as Supplementary file 1 of the revised manuscript. Because we use tens of thousands of sequences in our analyses, the tables are divided into smaller increments that could be downloaded from the GISAID server.
3) Thank you for providing the analysis code. It would be useful to if you could check this code into a git repo (e.g. on github) that eLife can then clone. A top-level README that explains the content of the different folders and its relation to the paper would also help.
We appreciate the opportunity to make our analysis code more widely available. We have deposited the code within the Github repository here: https://github.com/ksxue/parallel-evolution Per the reviewer suggestions, we have also added a top-level README file that describes the overall organization of the repository. The “analysis” folder, which contains most of the code used to generate the figures, also includes individual README files for each discrete analysis.
4) Please extend the discussion of the statistical tests for parallelism in the main text (subsection “Within-host evolution often parallels global evolutionary dynamics”, first paragraph) to give the reader a better idea of what is being tested. Please also discuss the fact that significant enrichment is only observed when at least half of all amino acids in HA are assumed mutable.
We substantially expanded our description of the statistical tests for parallelism in the main text, which we believe will benefit the readers.
Articles from eLife are provided here courtesy of eLife Sciences Publications, Ltd
Citations & impact
Impact metrics
Citations of article over time
Alternative metrics
Smart citations by scite.ai
Explore citation contexts and check if this article has been
supported or disputed.
https://scite.ai/reports/10.7554/elife.26875
Article citations
Influenza A virus within-host evolution and positive selection in a densely sampled household cohort over three seasons.
Virus Evol, 10(1):veae084, 03 Oct 2024
Cited by: 0 articles | PMID: 39444487 | PMCID: PMC11498174
Within-host influenza viral diversity in the pediatric population as a function of age, vaccine, and health status.
Virus Evol, 10(1):veae034, 25 Apr 2024
Cited by: 0 articles | PMID: 38859985 | PMCID: PMC11163376
Within-Host Rhinovirus Evolution in Upper and Lower Respiratory Tract Highlights Capsid Variability and Mutation-Independent Compartmentalization.
J Infect Dis, 229(2):403-412, 01 Feb 2024
Cited by: 1 article | PMID: 37486790 | PMCID: PMC10873175
Co-evolution of immunity and seasonal influenza viruses.
Nat Rev Microbiol, 21(12):805-817, 02 Aug 2023
Cited by: 11 articles | PMID: 37532870
Review
Genotype-phenotype landscapes for immune-pathogen coevolution.
Trends Immunol, 44(5):384-396, 04 Apr 2023
Cited by: 2 articles | PMID: 37024340 | PMCID: PMC10147585
Review Free full text in Europe PMC
Go to all (69) article citations
Data
Data behind the article
This data has been text mined from the article, or deposited into data resources.
BioStudies: supplemental material and supporting data
BioProject
- (2 citations) BioProject - PRJNA364676
Nucleotide Sequences (2)
- (3 citations) ENA - CY035022
- (1 citation) ENA - CY035029
Protein structures in PDBe (2)
-
(2 citations)
PDBe - 4HMGView structure
-
(1 citation)
PDBe - 2BATView structure
Similar Articles
To arrive at the top five similar articles we use a word-weighted algorithm to compare words from the Title and Abstract of each citation.
Intrahost dynamics of antiviral resistance in influenza A virus reflect complex patterns of segment linkage, reassortment, and natural selection.
mBio, 6(2):e02464-14, 07 Apr 2015
Cited by: 33 articles | PMID: 25852163 | PMCID: PMC4453542
Deep Sequencing Reveals Potential Antigenic Variants at Low Frequencies in Influenza A Virus-Infected Humans.
J Virol, 90(7):3355-3365, 06 Jan 2016
Cited by: 70 articles | PMID: 26739054 | PMCID: PMC4794676
Deep Sequencing of Influenza A Virus from a Human Challenge Study Reveals a Selective Bottleneck and Only Limited Intrahost Genetic Diversification.
J Virol, 90(24):11247-11258, 28 Nov 2016
Cited by: 66 articles | PMID: 27707932 | PMCID: PMC5126380
Implications of segment mismatch for influenza A virus evolution.
J Gen Virol, 99(1):3-16, 15 Dec 2017
Cited by: 53 articles | PMID: 29244017 | PMCID: PMC5882089
Review Free full text in Europe PMC
Funding
Funders who supported this work.
Hertz Foundation (1)
Grant ID: Hertz Graduate Fellowship
Howard Hughes Medical Institute (1)
Grant ID: Faculty Scholar Award
NHLBI NIH HHS (3)
Grant ID: K24 HL093294
Grant ID: K23 HL091059
Grant ID: R01 HL081595
NIAID NIH HHS (1)
Grant ID: R01 AI127893
NIGMS NIH HHS (2)
Grant ID: R01 GM102198
Grant ID: U54 GM111274
National Heart, Lung, and Blood Institute (3)
Grant ID: K23HL091059
Grant ID: K24HL093294
Grant ID: R01HL081595
National Institute of Allergy and Infectious Diseases (1)
Grant ID: R01AI127893
National Institute of General Medical Sciences (1)
Grant ID: R01GM102198
National Science Foundation (1)
Grant ID: DGE-1256082
Simons Foundation (1)
Grant ID: Faculty Scholar Award