Abstract
Free full text

Brain energy rescue: an emerging therapeutic concept for neurodegenerative disorders of ageing
Associated Data
Abstract
The brain requires a continuous supply of energy in the form of ATP, most of which is produced from glucose by oxidative phosphorylation in mitochondria, complemented by aerobic glycolysis in the cytoplasm. When glucose levels are limited, ketone bodies generated in the liver and lactate derived from exercising skeletal muscle can also become important energy substrates for the brain. In neurodegenerative disorders of ageing, brain glucose metabolism deteriorates in a progressive, region-specific and disease-specific manner — a problem that is best characterized in Alzheimer disease, where it begins presymptomatically. This Review discusses the status and prospects of therapeutic strategies for countering neurodegenerative disorders of ageing by improving, preserving or rescuing brain energetics. The approaches described include restoring oxidative phosphorylation and glycolysis, increasing insulin sensitivity, correcting mitochondrial dysfunction, ketone-based interventions, acting via hormones that modulate cerebral energetics, RNA therapeutics and complementary multimodal lifestyle changes.
Increased longevity over the past 50 years has contributed to a rising prevalence of neurodegenerative disorders of ageing (NDAs), particularly Alzheimer disease (AD) and Parkinson disease (PD). These disorders are a major socio-economic and medical challenge with little prospect of a solution so far. Some drugs provide a degree of symptomatic relief, but disease-modifying treatments for NDAs remain elusive despite concerted attempts to counter the pathological processes of neurotoxic protein accumulation, neuroinflammation, axonal or synaptic dysfunction and neuronal death1,2.
Since the concept was first reported 40 years ago, evidence has been accumulating that impaired brain energetics is involved in the cause and progression of NDAs, especially AD3–7. Brain energy metabolism declines subtly during ageing and this decline is frequently present before diagnosis of NDAs; it both drives and is driven by functional impairment and neurodegeneration in a destructive cycle3,6.
Accordingly, a broad range of ‘brain energy rescue’ strategies have recently been explored and are the focus of this Review. These strategies aim to impede the onset and progress of NDAs by improving, preserving and/or restoring brain energy status. We first summarize how fuel is supplied and used across various cell types in the brain, including central, peripheral and endocrine mechanisms that modulate brain energy homeostasis as well as cognitive and neuronal function. The core features of disrupted brain energetics in the five main NDAs — AD, PD, Huntington disease (HD), frontotemporal dementia (FTD) and amyotrophic lateral sclerosis (ALS) — are then outlined. This forms the basis for the brain energy rescue strategies reported in preclinical and clinical studies, including promoting mitochondrial function, alternative fuel sources such as ketone bodies (ketones), hormonal interventions to increase insulin sensitivity and brain glucose metabolism and complementary lifestyle approaches. Finally, we highlight genetic and other emerging approaches to enhance and restore brain energetics and we consider the challenges of translating promising preclinical results towards the dual goals of symptom relief and disease modification in NDAs.
Brain energetics
Despite representing slightly more than 2% of adult body weight, the human brain accounts for 20% of the body’s total energy requirement8. The brain’s main competitors for energy are the liver, kidneys and heart, which have as high or higher rates of energy consumption per gram, but their overall energy consumption is lower that of the brain. The immune system also consumes considerable energy, especially when activated in NDAs9. Brain energy metabolism is influenced by the endocrine modulation of appetite and whole-body energetics — processes that are compromised during healthy ageing and in NDAs10 (Supplementary Table 1). Food intake, glucose-sensing mechanisms and energy homeostasis are themselves regulated by a complex set of neural networks that in turn modulate autonomic function, appetite, reward and executive functioning. These aspects are beyond the scope of this Review, and have been reviewed elsewhere11,12.
ATP
ATP is the main currency of brain energy metabolism. Most ATP is used by Na+/K+-ATPase and Ca2+-ATPase, the cell membrane pumps that reset ion gradients during neuronal signalling8,13,14. Excitatory (glutamatergic) neurons consume 80–85% of the brain’s ATP, with inhibitory neurons and other cells accounting for the remainder15–17. Although non-signalling pathways and cellular processes, including axonal transport, maintenance of cytoskeletal architecture, proton leakage, microglial motility, DNA repair, RNA translation and phospholipid remodelling, consume less energy than neurotransmission, the energetic requirements of these processes remain hard to define13,16. ATP is also a neurotransmitter released from neurons, astrocytes and microglia18.
Glucose
Glucose metabolism fuels 95% or more of ATP production in the brain8. Within the brain, glucose uptake is orchestrated across several cell types collectively known as the neurovascular unit: brain capillary endothelial cells, pericytes, astrocytes, oligodendrocytes, microglia and neurons — the final beneficiary of glucose uptake19,20 (FIG. 1). The normally tight spatial and temporal association of local blood flow, oxygen consumption and glucose consumption in the brain is termed ‘neurovascular coupling’, and is the basis for functional magnetic resonance imaging20.
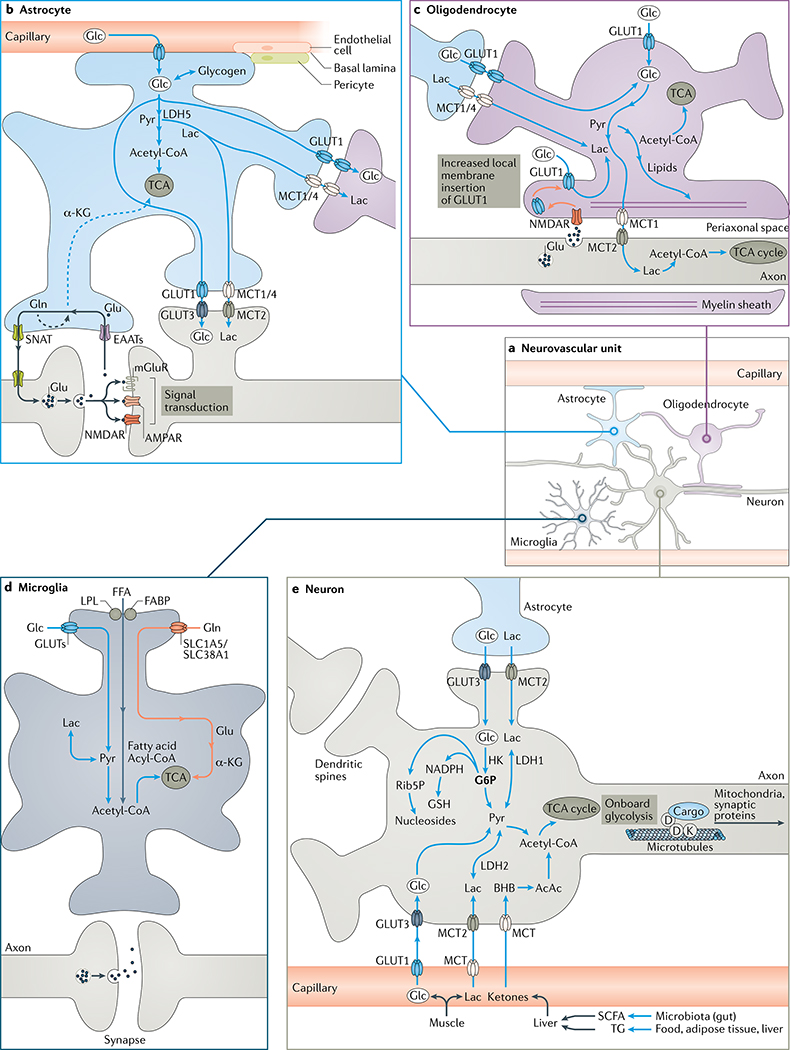
a | Organization of the neurovascular unit, which functions to supply glucose (Glc) to neurons. b | Astrocytes produce ATP mainly via aerobic glycolysis (glucose to pyruvate (Pyr)), yet also exploit oxidative phosphorylation in mitochondria (ovals) to generate ATP using the tricarboxylic acid (TCA) cycle. Astrocytes supply glucose to neurons and oligodendrocytes from capillaries and endogenous stores of glycogen, a reversible transformation. Astrocytes take up glutamate (Glu) released from synapses and convert it into glutamine (Gln), which is sent to neurons: this metabolically costly operation relieves neurons of an energetic burden. Some glutamate and glutamine contributes carbon to the TCA cycle via the intermediate α-ketoglutarate (α-KG). Astrocytic generation of ketones from acetyl coenzyme A (acetyl-CoA) and uptake of lactate (Lac) and medium-chain fatty acids is not shown for clarity. c | Oligodendrocytes insulate axons with myelin and deliver lactate to axons, which is transformed into pyruvate and then ATP by mitochondria. Axons promote their own energy supply by releasing glutamate to stimulate N-methyl-d-aspartate receptors (NMDARs) on oligodendrocytes; this promotes membrane insertion of GLUT1 and increased oligodendrocyte uptake of glucose delivered as lactate to axons. d | Microglia support neurons by clearing pathogens, waste and toxic proteins but do not provide them with energy. They generate ATP mainly from glucose but also from free fatty acids and glutamine. Fatty acid and fructose (prominent in ‘modern diets’) uptake by microglia is linked to neuroinflammation in neurodegenerative disorders of ageing. e | Neurons use transporters to acquire glucose and lactate from astrocytes or the vasculature. Food, adipose tissue and gut microbiota yield short-chain fatty acids (SCFAs) and triglycerides (TGs), which are transformed by the liver into the ketones d-β-hydroxybutyrate (BHB) and acetoacetate (AcAc), which are taken up by neurons. Glucose enters the TCA cycle via pyruvate and acetyl coenzyme A to yield ATP or the pentose phosphate pathway to provide (via glucose 6-phosphate (G6P)) the antioxidant glutathione (GSH) and nucleosides. Neurons also generate some ATP by aerobic glycolysis. Microtubule transport of mitochondria and other cargo is driven by ATP, partially generated by ‘onboard’ glycolytic enzymes. See the main text and Supplementary Fig. 1 for details. AMPAR, α-amino-3-hydroxy-5-methyl-4-isoxazolepropionic acid receptor; EAAT, excitatory amino acid transporter; FABP, fatty acid-binding protein; FFA, free fatty acid; GLUT, glucose transporter; HK, hexokinase; LDH, lactate dehydrogenase; LPL, lipoprotein lipase; MCT, monocarboxylate transporter; mGluR, metabotropic glutamate receptor; Rib5P, ribonucleoside 5-phosphate; SLC1A5, solute carrier family 1 member 5; SLC38A1, solute carrier family 38 member 1; SNAT, sodium-coupled neutral amino acid transporter.
Brain uptake of glucose from the circulation is driven by the energy demand of activated neurons not by the level of circulating glucose. Indeed, under normal conditions, the capacity to transport glucose into the brain exceeds the brain’s energy requirement by twofold to threefold15. Simply put, glucose is actively ‘pulled’ into an area of the brain in response to increased local neuronal activity. Glucose transport is achieved by the coordinated activity of glucose transporters on the capillary endothelium (GLUT1) and plasma membrane of astrocytes (GLUT1, GLUT2, and GLUT7), oligodendrocytes (GLUT1) and neurons (GLUT3 and GLUT4) in the cortex, hippocampus and cerebellum10,17,21. Only GLUT4 is mobilized as a direct response to sustained synaptic activity; its membrane insertion is stimulated by the metabolic sensor, AMP-activated protein kinase (AMPK)17. Membrane translocation of GLUT4 is insulin dependent in muscle and adipose tissue and probably also in neurons, so insulin resistance, as occurs in NDAs, is characterized by reduced neuronal glucose uptake17,22.
To reach neurons from capillaries, blood glucose either diffuses directly through the extracellular space or is channelled through astrocytes via their end-feet, which surround the capillary walls. It is taken up by astrocytic GLUT1 and exits through GLUT1 on perisynaptic processes adjacent to neurons and oligodendrocytes (FIG. 1). Some glucose that enters astrocytes is metabolized to ATP and some is converted to lactate, which can act both as a neurotransmitter (discussed later) and as an alternative energy source18.
Energy use by brain cells
The ATP required by neurons is predominantly generated within mitochondria by oxidative phosphorylation of glucose via the tricarboxylic acid cycle14 (TCA cycle; also known as the citric acid or Krebs cycle; BOX 1). Additional ATP is generated by aerobic glycolysis in the cytoplasm, which is required to support the high energy demands of synaptic transmission17. Glutamate is the neurotransmitter in most excitatory neurons and is recycled by astrocytes and delivered back to neurons as glutamine for reconversion into glutamate or (to a lesser degree) for use in energy generation by the TCA cycle13,23. Compared with astrocytes, neurons have more unphosphorylated (active) pyruvate dehydrogenase, more rapid TCA cycle activity and favour oxidative phosphorylation over aerobic glycolysis8. In contrast, the energy requirements of astrocytes are predominantly met by aerobic glycolysis, so some microdomains in astrocytes contain relatively few mitochondria23.
Neuronal activation transiently triggers aerobic glycolysis in astrocytes, thereby generating lactate. The astrocyte–neuron lactate shuttle hypothesis proposes that neuronal release of glutamate during neural transmission stimulates glucose uptake, glycogen catabolism, aerobic glycolysis and lactate production in neighbouring astrocytes8,24. The lactate produced by astrocytes is posited to support neuroplasticity, although its precise contribution and the conditions of lactate exploitation remain unclear8,10,15,23 (BOX 2).
Oligodendrocytes obtain ATP primarily by aerobic glycolysis. They use lactate for their own energy needs and also supply neighbouring axons with lactate, a process modulated locally by glutamate release from neurons (FIG. 1). This metabolic support of neuronal function by oligodendrocytes is important for effective spatial and temporal information processing in neuronal networks25. Oligodendrocytes are responsible for myelination of axons, which speeds up action potential conduction. However, the insulating myelin sheath restricts access of axons to glucose and other metabolites that would otherwise diffuse across the extracellular space25. The intermittent pattern of axonal myelination in cortical grey matter helps maintain access to extracellular nutrients26. Therefore, the supply of glucose and lactate to myelinated axons by oligodendrocytes requires a highly specialized architecture of the myelin sheath, including a continuum of nanometre-wide cytosolic channels that flank compacted, mature myelin25 (FIG. 1). These channels connect the oligodendrocyte soma with the periaxonal space. Neurons reciprocally deliver N-acetylaspartate to the oligodendrocyte soma via these channels; this N-acetylaspartate (as part of the aspartate–oxaloaspartate–malate shuttle) stimulates the TCA cycle and mitochondrial ATP production and is used to generate lipids and myelin27.
Axons also transport mitochondria, RNA, proteins, vesicles and other cargo to presynaptic terminals. This transport is an ATP-dependent process regulated by calcium and involves motor proteins and microtubules. Retrograde transport of vesicles to the cell body for lysosomal degradation is ATP driven2. Fast-conducting axons release trace amounts of glutamate, which stimulates local N-methyl-d-aspartate (NMDA) receptors in oligodendrocytes, promoting surface expression of GLUT1 on axonal myelin sheaths and thereby increasing glucose uptake and the rate of aerobic glycolysis. This in turn increases local provision of lactate to axons for ATP generation21,28 (FIG. 1). In addition, the molecular motors driving fast axonal transport are equipped with glycolytic enzymes, allowing them to generate their own energy ‘onboard’29 (FIG. 1).
Unlike astrocytes and oligodendrocytes, microglia do not directly provide energy to neurons, but the high amounts of lactate released by activated microglia may well be retrieved by local neurons23. Microglia are predominantly fuelled by oxidative phosphorylation but are metabolically reprogrammed by neuroinflammation in NDAs to an aerobic glycolysis-predominant phenotype associated with upregulation of GLUT1 and GLUT4 (REFS1,2). In parallel with this energetic shift, microglia transition from a protective to a disease-driving role in NDAs. When brain glucose supply is suboptimal for a long time, the high energy demands of activated microglia further limit energy availability to neurons1,30.
Neuronal networks and energy use
The provision of energy substrates from astrocytes to synapses and from oligodendrocytes to axons is critical for communication both within and between brain networks25. Brain regions are connected by tracts of myelinated axons, which are adversely affected by NDAs. For example, corticocortical loops are disrupted in AD and FTD, corticostriatal pathways are disrupted in HD, corticospinal tracts are disrupted in ALS and nigrostriatal projections are disrupted in PD. These tracts consist mainly of long-range excitatory neurons. Inhibitory interneurons, such as fast-spiking interneurons, are mostly present within local networks, such as the CA3 region of the hippocampus and frontal cortex. Local interneuron dysfunction disrupts synchronization between remote neuronal networks and across brain regions31,32.
Gamma oscillations (30–100 Hz) are fast brain rhythms synchronizing the activity of excitatory principal neurons and neuronal networks31. Fast-spiking GABAergic interneurons generating gamma oscillations have a high density of mitochondria in their axons and a specialized kind of myelination that facilitates provision of energy by oligodendrocytes33. The high metabolic needs of fast-spiking interneurons are supported primarily by oxidative phosphorylation. Parvalbumin-positive, GABAergic interneurons are particularly sensitive to deficits in energy and oxygen supply13,34. The decreased ability of oligodendrocytes to provide lactate to axons probably aggravates the decline in fast-spiking interneuron activity observed in NDAs.
Rhythmically firing, highly branched, nigrostriatal dopaminergic neurons in the substantia nigra pars compacta are especially vulnerable to mitochondrial failure and oxidative stress, features characteristic of PD13,35 (Supplementary Table 1).
Neuroendocrine mechanisms
As demonstrated in diverse cellular and animal models, insulin has a globally positive influence on cerebral energy balance and function. It reinforces neuronal energy supply by increasing neuronal glucose uptake by GLUT4 in the hippocampus and cortex17,22 (Supplementary Table 2). Insulin and activation of insulin-like growth factor 1 (IGF1) receptors also promote synaptic plasticity and cognitive processes36. Nevertheless, normal insulin sensitivity is paramount; insulin resistance is a major risk factor for AD because it disrupts both the modulation of energy availability by insulin and insulin signalling pathways in the brain37,38.
Other hormones, including ghrelin, incretins, leptin, amylin and adiponectin, modulate both appetite and energy homeostasis and influence numerous aspects of brain function that are compromised in NDAs. The neurobiology of these hormones and their synthetic agonists in relation to food intake and energy homeostasis, brain energy balance, mitochondrial function, cognition, motor function, neurogenesis, synaptic integrity and neuronal integrity in animal models of NDAs are documented in Supplementary Table 2, with their clinical effects reported in TABLES 1–3 and in the section entitled ‘Therapies based on brain energy rescue’.
Table 1 |
Treatments that improve brain energetics and/or function in preclinical models of NDAs
Study characteristics | Primary end points: results | Ref. |
---|---|---|
Mitochondrial function | ||
AD mice (3xTg) receiving MitoQ (100 μM in drinking water) for 5 months | Mitochondrial function: ↓ cognitive decline, ↓oxidative stress, ↓ Aβ accumulation, ↓ astrogliosis, ↓ synaptic loss, ↓ caspase activation, ↓ neuropathology, ↑ mitochondrial function | 257 |
AD mice (APP/PS1) receiving CP2 at 25 mg kg−1 per day for 14 months | Mitochondrial function:↓ complex I activity, ↑ AMPK level, ↑ mitochondrial bioenergetics | 128 |
AD mice (APP/PS1) receiving 25–250 μM AP39 in neurons in culture; 100 nmol AP39 per kilogram body weight for 6 weeks | Brain energy status and mitochondrial function: ↑ brain ATP level, protected against mitochondrial DNA damage, ↓ ROS concentration, ↓ brain atrophy | 258 |
AD mice (APP/PS1) receiving mdivi-1 at 10 or 40 mg kg−1 by gavage for 1 month | Mitochondrial dynamics: ↓ mitochondrial fragmentation, ↓ loss of mitochondrial membrane potential, ↓ ROS concentration, ↓ synaptic dysfunction,↑ ATP concentration, ↑ learning and memory, ↑ mitochondrial function | 136 |
AD mice (APP/PS1) receiving nicotinamide riboside at 400 mg kg−1 per day for 10 weeks | Mitochondrial function and proteostasis: ↓ Aβ accumulation, ↑ cognitive function, ↑ oxidative phosphorylation activity | 259 |
PD rats (hA53T-α-syn) receiving mdivi-1 at 20 mg kg−1 by intraperitoneal injection for 8 weeks | Mitochondrial dynamics: ↓ mitochondrial fragmentation, ↓ mitochondrial dysfunction, ↓ oxidative stress, ↓ neurodegeneration and α-syn aggregates, ↑ motor function | 135 |
HD mice (HD R6/2 and YAC128) receiving DA1 peptides (1 mg kg−1 per day) via osmotic pump for 2–3 months | Mitochondrial dynamics and function: ↑ mitochondrial biogenesis and bioenergetics, ↓ inflammation and neuropathology | 260 |
ALS mice (Sod1G93A) receiving MitoQ (500 μM in drinking water) for 30–40 days | Mitochondrial function: ↓ nitroxidative stress, ↓ neuropathology, ↑ mitochondrial function and lifespan | 261 |
SCA1 mice (Sca1154Q/2Q) receiving MitoQ (500 μM in drinking water) for 16 weeks | Mitochondrial function: ↓ neuropathology, ↓ oxidative stress, ↓ DNA damage, ↓ neuronal loss, ↑ mitochondrial function | 262 |
Seizure model (risk of AD). In vivo study: CD1 mice; 35% of energy from C10 in regular diet. In vitro study: astrocytes exposed to 200 mM C8 or C10 for 10 days | Seizures — in vivo study: ↓ seizures after C10 but not C8; no change in levels of glycolytic enzymes. In vitro study: C8 and C10: ↑ basal respiration and mitochondrial leak; ↑ ATP synthesis, ↑ antioxidant capacity caused by C10 but not C8 | 150 |
Insulin sensitizers | ||
AD mice (APP/PS1) receiving metformin at 200 mg kg−1 intraperitoneally for 14 days | Cognitive performance, neuropathology: ↑ cognitive performance (Morris water maze), ↓ hippocampal neuron loss, ↓ Aβ accumulation, ↓ neuroinflammation | 176 |
HD mice (Hdh150 knock-in) receiving metformin in drinking water at 5 mg ml−1 for 3 weeks | Early network hyperactivation in visual cortex, behaviour: ↓ hyperactive neurons, ↑ normal network patterns, ↓ green fluorescent protein–huntingtin synthesis, ↓ anxiolytic behaviour | 177 |
Ketogenic molecules | ||
AD mice (APP/PS1) receiving BHB and pyruvate at 26 mg kg−1 per day for 5 weeks | Brain redox status: ↑ brain nicotinamide adenine dinucleotide phosphate (reduced) level; ↓ network hyperactivity (epileptiform discharges) | 146 |
AD mice (3xTgAD) receiving 125 g ketone ester per kg in diet for 8 months | Brain TCA cycle activity, mitochondrial function: BHB level ↑ fivefold; 30–40% ↑ in level of brain TCA cycle and glycolytic intermediates; ↑ mitochondrial redox potential; ↓ level of oxidized lipids/proteins in hippocampus | 263 |
AD mice (Sirt3+/−/AppPs1) receiving ketone ester added at 22% of dietary energy for 20 weeks | Neurodegeneration, neuronal network hyperexcitability: ↑ cortical SIRT3 expression, ↓ loss of GABAergic neurons, ↓ seizures and prevented death of Sirt3+/−/AppPs1 mice | 264 |
PD mice receiving MPTP at 18 mg kg−1 4 times over 2 h and BHB at 40, 80 or 160 mg kg−1 per day for 7 days | Mitochondrial function: ↑ mitochondrial respiration and ATP at complex II, ↓ dopamine neurodegeneration and motor deficit | 265 |
ALS mice (SOD1-G93A) receiving 10% of calories as C8 for 10 weeks (7–17 weeks old) | Physical symptoms: ↑ mitochondrial function, ↓ spinal cord motor neuron loss, ↑ mitochondrial O2 consumption, no change in survival | 266 |
Nutrients and metabolites | ||
AD mice (Tg2576) receiving nicotinamide riboside at 250 mg kg−1 per day for 3 months | Brain redox status, cognitive performance: ↑ cortical redox status; attenuated cognitive decline | 267 |
AD mice (3xTgAD/Polb+/−) receiving nicotinamide riboside at 3 g l−1 (12 mM) in drinking water for 6 months | Brain redox status: normalized cortical NAD+/NADH; nicotinamide riboside ↑ cognitive function and restored hippocampal synaptic plasticity | 142 |
AD mice (treated with streptozotocin) receiving N-acetylcysteine at 50 mg kg−1 for 9 days | Brain glucose uptake, cognitive performance: normalized glucose uptake in hippocampus after streptozotocin treatment; prevented spatial/non-spatial learning and memory impairment | 268 |
ALS mice (SOD1G93A) receiving 35% of calories as triheptanoin for 5 weeks (35–70 days old) | Physical performance, TCA cycle activity, brain glucose uptake, cognitive performance: ↑ hindlimb grip strength by 2.8 weeks, ↑ time to loss of balance on rotarod, ↑ time before weight loss, ↑ TCA cycle | 269 |
Reports shown here exclude those involving the ketogenic diet and lifestyle interventions (see BOX 3); studies involving antioxidants are documented in other reviews60. Triheptanoin269 is a seven-carbon triglyceride. The APP/PS1 Alzheimer disease (AD) mice bear the APP Swedish mutation plus the PS1-L166P mutation. 3xTgAD mice express three mutant proteins (APP Swedish, PS1-M146L and tau-P301L). ↑, increase; ↓, decrease; 3xTg, triple transgenic (amyloid precursor protein, tau and presenilin-1); Aβ, amyloid-β; AMPK, AMP-activated protein kinase; ALS, amyotrophic lateral sclerosis; AP39, proprietary mitochondrial-targeted H2S donor; BHB, d-β-hydroxybutyrate; C8, octanoic acid; C10, decanonic acid; DA1, dynamin-related protein antagonist 1; hA53T, adenosine to threonine missense mutation at position 53 of human synuclein; HD, Huntington disease; mdivi-1, mitochondrial division inhibitor 1; MPTP, 1-methyl-4-phenyl-1,2,3,6-tetrahydropyridine; NDAs, neurodegenerative disorders of ageing; PD, Parkinson disease; Pol, DNA polymerase; ROS, reactive oxygen species; SCA1, spinocerebellar ataxia type 1; α-Syn, α-synuclein; TCA, tricarboxylic acid; YAC, yeast artificial chromosome.
Table 3 |
Trials reporting improved brain function and/or energetics in major risk conditions for NDAs
Disorder | Study details | Results and comments | Ref. |
---|---|---|---|
T2D ± cognitive impairment | Open-label study (n = 205) of DPP4 inhibitor (sitagliptin) at 100 mg per day ± metformin ± insulin (n = 101) vs metformin ± insulin only (n = 104) for 6 months | ↑ MMSE score in DPP4 inhibitor arm; similar glycaemic control in both groups; ↓ insulin level in DPP4 inhibitor arm; RCT needed | 197 |
MCI or mild AD (non-diabetic) | Placebo-controlled, parallel-group crossover study (n = 10 per group) of metformin at 2 g per day for 8 weeks (NCT01965756) | Metformin measurable in CSF; no change in CSF Aβ42 or phosphorylated tau levels; ↑ CBF in two brain regions at 8 weeks; trend to ↑ executive function, memory and attention. Metformin penetrates brain; underpowered for cognitive outcomes | 186 |
MCI | RCT of high-carbohydrate (n = 11) or high-fat (n = 12) KD for 6 weeks (NCT00777010) | Feasibility study; cognitive outcomes (executive function, long-term memory), mood: on KD, ↑ memory (paired associate learning); no change in executive function or depression score. Metabolic improvement for KD: ↓ weight, waist circumference, fasting glucose level and fasting insulin level | 155 |
MCI | RCT of C8C10 at 30 g per day (n = 19) or energy-matched non-ketogenic placebo (n = 20) for 6 months (NCT02551419) | Brain glucose and ketone status: ↑ brain ketone uptake twofold. ↑ executive function, episodic memory, language and processing speed. Several cognitive outcomes improved in direct proportion to ketone concentration and/or brain ketone uptake | 154 |
SMC and MCI | Crossover RCT with 6-week washout in between 6 weeks of AHAD and 6 weeks of MMKD interventions, in patients with SMC (n = 11) or MCI (n = 9) (NCT2984540) | CSF AD markers, neuroimaging markers, peripheral metabolic status, cognition. For MMKD only: ↑ CSF Aβ42 level, ↓ CSF tau level, ↑ brain ketone levels and ↑ brain perfusion. In both groups: ↑ levels of metabolic markers and ↑ memory. Adherence ≥90% in both groups; MMKD feasible, acceptable and has prevention effect on AD CSF biomarkers | 156 |
↑, increase; ↓, decrease; Aβ42, 42 amino acid isoform of amyloid-β; AD, Alzheimer disease; ADAS-Cog, Alzheimer Disease Assessment Scale - Cognitive Subscale; AHAD, American Heart Association diet; C8C10, octanoic acid plus decanoic acid; CSF, cerebrospinal fluid; DPP4, dipeptidyl peptidase 4; KD, ketogenic diet; MCI, mild cognitive impartment; MMKD, modified Mediterranean ketogenic diet; MMSE, Mini Mental State Examination; NDAs, neurodegenerative disorders of ageing; RCT, randomized controlled trial; SMC, subjective memory impairment; T2D, type 2 diabetes.
Brain use of ketones and lactate
Glucose (and glycogen) reserves within the brain can supply its ATP needs for only a few minutes24,39. Much of the brain’s resilience in the face of energetic challenge therefore depends on opportunistic use of alternative fuels sourced from outside the brain. Ketones and lactate are the main alternative fuels to glucose and are delivered to the brain by monocarboxylate transporters on astrocytes and on the capillary endothelium (FIG. 1). The two principal ketones, acetoacetate and d-β-hydroxybutyrate (BHB), are the main alternative brain fuels to glucose in adults under conditions of dietary carbohydrate or energy restriction. In infants, however, ketones are not only an essential brain fuel but are also the main substrate for brain lipid synthesis40.
Acetoacetate and BHB are both in equilibrium in the blood, but only acetoacetate is metabolized to acetyl coenzyme A (acetyl-CoA), which then enters the TCA cycle to generate ATP. After an overnight fast, plasma ketone concentrations are usually 0.1–0.2 mM and they supply 3–5% of brain energy requirements. However, during a 3–4-day fast, plasma ketone concentrations can reach 5–6 mM and they provide 50% or more of brain energy requirements3. Unlike the entry rate of glucose, which enters the brain in response to brain cell activity, the rate of entry of ketones to the brain is directly related to their plasma concentration3, which explains the glucose-sparing effect of increased ketone levels41. Unlike glucose, ketones do not undergo aerobic glycolysis and cannot be metabolized to lactate, so they contribute to ATP production only via oxidative phosphorylation.
The gut–brain axis
Gut microbiota are involved in bidirectional communication between the gastrointestinal tract and the brain, generating nutrients and modulating overall energy homeostasis42. Disruption of the gut microbiota (dysbiosis) is implicated in the pathogenesis of NDAs43. Dietary fibre is an important substrate for generation of short-chain fatty acids by the gut microbiota; dietary fibre also slows down glucose absorption, which increases insulin sensitivity. These effects of dietary fibre are partly mediated by short-chain fatty acids, which are ligands for G-protein-coupled hydroxycarboxylic acid receptors (such as HCAR2) in enterocytes42,44. Short-chain fatty acids produced by gut bacteria, particularly butyrate, are key fuels for intestinal cells. Propionate, butyrate and succinate (a precursor of propionate) generated by the microbiota also improve control of peripheral glucose metabolism, adiposity and body weight44. In preclinical studies, exogenous butyrate promoted the development of dendritic spines, long-term potentiation, myelination and memory formation45. However, butyrate levels produced endogenously are usually low, so butyrate would at best be expected to be a minor energy substrate for the brain.
The beneficial effect of intestinal gluconeogenesis on insulin sensitivity and systemic energy metabolism is mediated in part by glucose sensing in the portal vein. This information is relayed via portal sensory nerves to the brain, which suppresses appetite and reduces hepatic gluconeogenesis44,46. Short-chain fatty acids also modulate the immune system, stimulate the release of hormones such as glucagon-like peptide 1 (GLP1) from the gut (Supplementary Table 2) and inhibit histone deacetylases.
Impaired brain energetics
Impaired brain glucose metabolism compromises transmembrane ion transport, vesicle recycling and synaptic signalling17,31,34. Less effective maintenance of transmembrane ion gradients and transmitter release, especially in fast-spiking interneurons, leads to hyperexcitability, excitatory–inhibitory imbalance and functional impairment of cortical networks, which further compromises the brain’s energy efficiency. These changes are exacerbated by disrupted glutamatergic transmission and abnormal astrocyte and oligodendrocyte function13,34,47,48, as well as impaired autophagy, which, in turn, decreases nutrient recycling2. Furthermore, the neuroinflammation that is common to diverse classes of NDAs is energetically costly2,49.
The regional pattern of brain energetic disruption depends on the NDA and its pathophysiological phenotype (Supplementary Table 1). Indeed, brain glucose hypometabolism in NDAs has no single cause; reduction in neuronal glucose uptake, impairment of aerobic glycolysis and the TCA cycle, failure of axonal transport and the loss of glial energetic support to neurons are all implicated. Supplementary Box 1 outlines how induced pluripotent stem cells and organoids are illuminating the cellular substrates of energy dysregulation, and Supplementary Box 2 summarizes how disruption of the cerebral microvasculature exacerbates the disruption of brain energy supply in NDAs.
Alzheimer disease
AD is the most common NDA. It is associated with weight loss and poor appetite but also type 2 diabetes (T2D), all of which contribute to lower brain energy availability. AD is characterized by lower uptake of glucose, TCA activity, mitochondrial function and astrocyte and oligodendrocyte energetic support of neurons. In addition, microglial consumption of glucose due to neuroinflammation is elevated, siphoning energy away from neurons1,4,50–52 (see Supplementary Table 1).
Even before diagnosis of AD, a characteristic regional disruption of glucose metabolism is linked to neuropathology and reduced cerebral blood flow in the brain. Nevertheless, the brain in AD still has normal or near-normal oxygen, lactate and ketone metabolism3,52–54. Many positron emission tomography (PET) studies confirm that the entorhinal cortex and parietal lobes, including the precuneus, have a 10–12% deficit in glucose uptake in mild cognitive impairment (MCI), a deficit that becomes anatomically more widespread with the onset of AD and worsens during its progression (Supplementary Table 1). The regional pattern of the brain glucose deficit distinguishes AD from FTD, PD, Lewy body disease and other disorders associated with dementia3,4,6.
White matter atrophy in AD impairs neuronal network operation and axonal mitochondrial transport. Especially in women, white matter loss reflects reduced maintenance and synthesis of myelin (energy-intensive processes) and catabolism of myelin to provide energy in the face of glucose scarcity37,55,56. However, as with impaired glucose uptake in grey matter, white matter ketone uptake remains normal in AD57.
Parkinson disease
In idiopathic PD, weight loss and low body mass index are common despite increased visceral fat. A decline in glucose metabolism is seen in the striatum (caudate), the frontal cortex and several other cortical regions but not in the cerebellum: this pattern of hypometabolism correlates with specific patterns of motor and cognitive dysfunction and is predictive of disease progression4,58,59. Although PET cannot resolve the substantia nigra pars compacta (where dopaminergic cell bodies degenerate in PD), mitochondrial fragmentation and dysfunction, including decreased glycolysis and reduced complex I activity, is pronounced in this brain region50,60. Energetic deficits have been reproduced in PD-derived induced pluripotent stem cells overexpressing the gene encoding α-synuclein61. There is evidence that neuroinflammation further compromises neuronal fuel supply in PD1.
Huntington disease
Patients with HD characteristically lose weight, even when increasing their calorie intake. In presymptomatic HD, brain glucose hypometabolism is seen in the striatum, frontal cortex and temporal cortex, and is linked to impaired neurotransmission in corticostriatal tracts62. Glucose uptake, ATP generation by aerobic glycolysis, mitochondrial function and oxidative phosphorylation are all decreased in HD35,63,64. Astrocytes in the striatum may oxidize fatty acids as an alternative source of energy, but reactive oxygen species contribute to further tissue damage65. The cerebellum is less severely affected by impaired glucose metabolism than the basal ganglia, perhaps because it uses amino acids for gluconeogenesis65. HD neurons show disrupted glycolysis66 and impaired axonal transport of vesicles owing to the interference of mutant protein huntingtin with molecular motors35,67.
ALS and FTD
FTD and ALS have overlapping genetic risk factors and clinical and pathophysiological features6,68–70. Both are characterized by increased energy expenditure, yet only in FTD is there a distinctive carbohydrate/sweetness preference and weight gain. In contrast, patients with ALS eventually lose weight due to insufficient nutrient and energy intake68,71 (Supplementary Table 1). Brain energetics also deteriorate differently in ALS and FTD. FTD is associated with declining glucose metabolism and cerebral blood flow, especially in the frontal lobes, striatum and thalamus, where mitochondrial function is disrupted with reduced signalling to the endoplasmic reticulum and aberrant mitophagy6,68,69,72. Conversely, ALS is associated with a regionally complex pattern of lower and higher brain glucose metabolism: of particular note are reductions in mitochondrial function and glycolysis in the cortex, spinal cord and motor neurons, and at neuromuscular junctions in muscle68,70,73. In the superoxidase dismutase 1 (SOD1) mouse model of ALS, the pentose phosphate pathway is also impaired71. Furthermore, a loss of mitochondrial energetics and impaired glycolysis in astrocytes is linked to disruption of C9orf72, a genetic risk factor for ALS associated with failure of energetic support of neurons by astrocytes and oligodendrocytes74,75.
Energy deficits and neurotoxic proteins
Brain glucose hypometabolism contributes to synapse loss and neuronal death in AD, with energetic deficits and neurotoxic protein accumulation mutually aggravating one another in a vicious cycle3,52,76,77 (FIG. 2a). Insufficient neuronal glucose and mitochondrial energy generation compromise the clearance of the 42 amino acid isoform of amyloid-β (Aβ42) and tau proteins from the brain. Conversely, accumulation of Aβ42 and tau triggers mitochondrial damage, impairs energy production and increases oxidative stress77,78. These neurotoxic proteins also inhibit GLUT4 (REF.51) and phosphofructokinase, thereby blocking glucose uptake, aerobic glycolysis and ATP synthesis79. Mitochondria accumulate in axonal swellings and are no longer replaced in presynaptic terminals80. Failure to clear dysfunctional mitochondria by mitophagy further compromises the bioenergetics of vulnerable neuronal circuits in AD, PD and other NDAs2. Excitation–inhibition balance is crucial for network operation at optimal energetic efficiency48 and, at the circuit level, an early, neurotoxic protein-driven feature of AD is the energetically expensive hyperexcitability of glutamatergic neurons34,81, which is associated with an imbalance between excitation and inhibition in local cortical and hippocampal networks32,47.
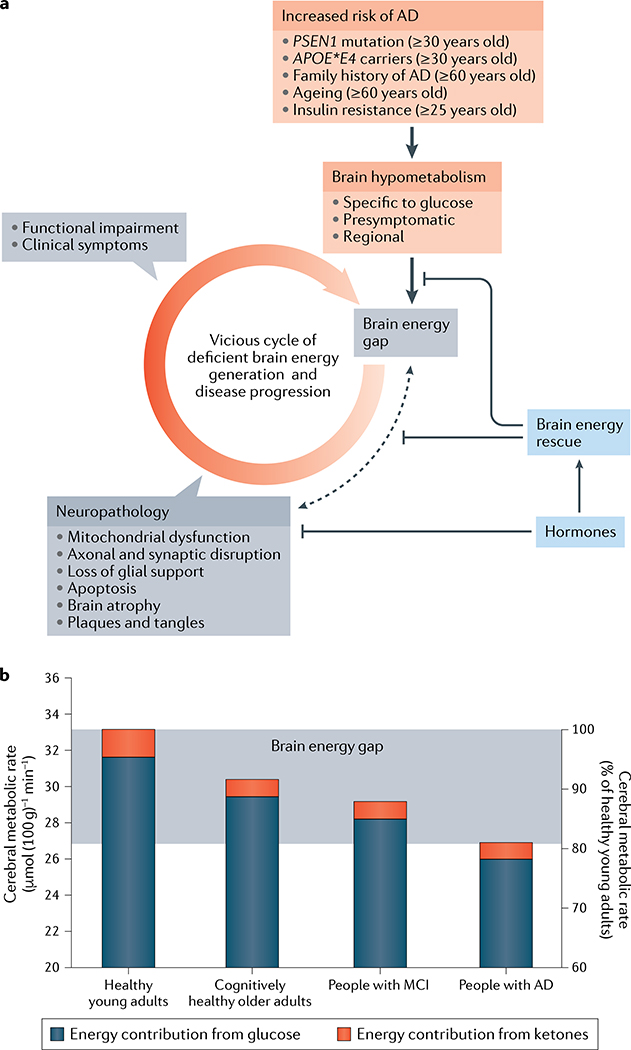
a | Brain glucose hypometabolism occurs in conditions that increase the risk of Alzheimer disease (AD). The persistent brain energy gap and the neuropathological processes both contribute to a vicious cycle leading to brain energy exhaustion and dysfunction. Brain energy rescue strategies (FIG. 3; TABLES 1–3) attempt to inhibit the positive feedback between the brain energy gap and neuropathology involving amyloid-β and phosphorylated tau (dashed black arrow). Hormones (principally insulin, adipokines and incretins), as well as synthetic agonists and insulin sensitizers, can influence brain energy rescue and inhibit the onset of neuropathology. b | Glucose contributes to about 95% of total brain fuel supply in cognitively healthy young adults, and ketones supply the remaining 5%. In cognitively healthy older adults, brain glucose uptake is decreased by about 9%, in people with mild cognitive impairment (MCI) it is decreased by about 12% and in people with mild-to-moderate AD it is decreased by about 18%. The magnitude of the brain energy gap is the difference in total brain fuel uptake (glucose and ketones combined) between healthy young adults and people with mild-to-moderate AD; that is, the therapeutic target for brain energy rescue in MCI and AD. The brain energy gap has not been rigorously quantified in neurodegenerative disorders of ageing other than AD.
Aβ is involved in a healthy neuronal response to damage and/or infection82, but this protective function is lost when Aβ aggregates into plaques. Aβ exacerbates brain glucose hypometabolism, both in foci of Aβ accumulation and in remote regions, possibly due to a pericyte-mediated constriction of capillary blood flow. In turn, this hypometabolism triggers cellular damage and neuroinflammation77,83. Perturbed astrocytic and oligodendrocyte function, together with accumulation of phosphorylated tau, exacerbates ageing32 and Aβ/phosphorylated tau-induced network hyperexcitability, thereby perpetuating a cycle of neurodegeneration and declining brain glucose metabolism13,47 (FIG. 2a). This vicious cycle driven by energy failure in AD has similarities with the neural circuit disruption seen in schizophrenia84 and in epilepsy4, and contributes not only to deterioration of memory and cognition but also to abnormal behaviour in affected patients.
Energetics and endocrine dysregulation
Insulin resistance is a common feature of AD, PD, FTD, ALS and probably also HD, with reduced signalling at central insulin and IGF1 receptors contributing to deficits in neural function, synaptic plasticity and cellular integrity36,37,85 (TABLE 1; Supplementary Table 1). Even though insulin itself does not globally promote brain glucose uptake, insulin resistance reduces glucose uptake by corticohippocampal neurons that express insulin-sensitive GLUT4 (REFS17,22).
NDAs are associated with numerous changes in hormones that modulate brain energetics and neuroplasticity (Supplementary Table 2). The following observations may be highlighted. First, plasma leptin and hippocampal leptin signalling are reduced in AD, resulting in a state of leptin resistance mirroring insulin resistance86. This decline in leptin signalling is superimposed on a background of declining plasma leptin concentration with ageing and is linked to impaired learning, memory and long-term potentiation87. Second, an age-related reduction in ghrelin signalling in the temporal cortex may be related to neuronal damage and cognitive deficits in AD88. Circulating ghrelin concentration is reduced in PD, and the loss of its neuroprotective properties is linked to dopaminergic neuron degeneration and motor dysfunction88,89. In addition to blunted neuroprotective properties, antineuro-inflammatory effects of ghrelin involving astrocytes and microglia may be diminished in AD and PD88,90. Third, amylin oligomers and aggregates are suspected to damage neurons and the microvasculature in AD, although amylin has a Janus-faced role as further discussed later91–93. Fourth, an increase in circulating adiponectin levels has been reported in AD and ALS: if centrally expressed, this increase might counter cognitive deficits and exert neuroprotective properties, but this awaits confirmation86,94–96. In contrast to the above-mentioned hormones, there are very few data on the relationship between GLP1 and glucose-dependent insulinotropic polypeptide (GIP) and NDAs97 (Supplementary Table 1). The levels of brain hormones are challenging to measure and cause–effect relationships are hard to disentangle, but changes in the secretion and central actions of these hormones are implicated in the energy imbalance, pathophysiology and functional deficits in NDAs (Supplementary Table 2).
Energetics and disease risk factors
Age
Ageing is the main risk factor for NDAs, but there is an important distinction between the cognitive, structural and neurometabolic changes associated with healthy ageing and those occurring in NDAs. During healthy ageing, some cognitive domains such as episodic and working memory and processing speed show a modest decline, whereas others (such as semantic memory) change relatively little98. Although the decline in brain volume and cortical thickness forms a continuum between cognitively healthy ageing, MCI and AD, regional changes in brain glucose metabolism seen during healthy ageing are quantitatively and qualitatively different from those in MCI and AD99,100. In healthy ageing, brain glucose metabolism decreases mainly in the frontal cortex, whereas in MCI and AD, the parietal lobe and precuneus are the most markedly affected. Decreased aerobic glycolysis101, loss of myelination, network perturbation and attenuation of neurovascular coupling are integral features of the ageing brain that might provide a template for the onset of the more severe brain energetic deficits in NDAs32,56,102,103. Mitochondrial proteins are expressed at lower levels in brains of older people experiencing accelerated cognitive decline104.
Metabolic dysregulation
The risk of NDAs is substantially higher in conditions of systemic metabolic dysregulation, including insulin resistance, obesity and T2D105 (TABLE 3). Most strikingly, poorly controlled type 1 diabetes (T1D) or T2D is associated with increased risk of cognitive impairment and AD106. Intriguingly, similarities exist between AD and T2D with respect to the deleterious effects of Aβ in the AD brain and the disruptive actions of amylin in the pancreas and brain in T2D: both disorders are associated with neurotoxic protein-induced peripheral metabolic and vascular abnormalities107. In young women with polycystic ovary syndrome, mild insulin resistance is associated with a pattern of glucose-specific brain hypometabolism similar to that seen in older people108, suggesting that the adverse effect of insulin resistance on brain energy metabolism is independent of age.
T2D doubles the risk of developing PD, possibly owing to increased expression of α-synuclein85. The risk of ALS is increased in T1D, but obesity and T2D are associated with decreased risk of ALS2,74. The metabolic syndrome associated with insulin resistance and weight gain is also present in ‘atypical’ major depression, itself often co-morbid with NDAs, especially AD and PD109. Effective treatment of T2D, metabolic syndrome and depression would be expected to reduce the risk of developing AD and other NDAs110.
Despite the persistent deficit in brain glucose uptake and utilization in NDAs, the normal ketogenic response to low plasma glucose levels is not stimulated because the main drivers of endogenous ketone production — low blood glucose and low insulin levels — are absent. Mild hyperglycaemia and mild insulin resistance commonly develop as people age, so plasma insulin levels rarely drop for long enough to release the insulin-mediated inhibition of lipolysis in adipose tissue, the source of the endogenous free fatty acids needed for ketogenesis. This metabolic deterioration continues as AD develops, so the brain experiences a persistent, progressive glucose-specific brain energy gap3 (FIG. 2a) that is not corrected by endogenous ketone production as it would be if insulin sensitivity were normal and plasma glucose concentration were decreased by a period of carbohydrate or caloric restriction.
Oestrogen
Menopause is associated with deteriorating systemic and brain glucose metabolism, weight gain, insulin resistance and loss of mitochondrial efficiency111. Ovariectomized rodent models of menopause show metabolic responses similar to fasting, including increased oxidation of long-chain fatty acids and elevated plasma levels of ketones, as well as white matter and myelin degeneration, changes that in part reflect the use of brain lipids as a source of fatty acids for ATP generation56,112. Oestrogen modulates many facets of brain glucose metabolism, including uptake, aerobic glycolysis and oxidative phosphorylation37. Oestrogen also stimulates the catabolism and clearance of Aβ, in part by upregulating insulin-degrading enzyme, so the loss of oestrogen after menopause could directly favour pathological processes leading to AD56,112. Accordingly, low oestrogen concentration in plasma is associated with an increased incidence of AD in women, although this relationship remains controversial37,112.
Genetic risk factors
Possession of two APOE*E4 alleles (encoding the E4 isoform of apolipoprotein E (ApoE4)) confers the highest genetic risk of sporadic AD. In APOE*E4 carriers the brain is hyperexcitable113, has reduced glucose utilization in regions affected by glucose hypometabolism in AD114 and accumulates more aggregated Aβ. Regardless of age, the following parameters all decline in APOE*E4 carriers in response to a high-fat diet: brain insulin signalling115, expression of glucose-regulating enzymes and glucose transporters114, mitochondrial function in the cortex and cognitive function104,116. These effects of ApoE4 on brain energetics are additive to the adverse effects of Aβ77.
Some of the adverse effects of ApoE4 may result from production of a carboxy-terminal fragment of ApoE4, which inhibits the electron transport chain117,118, increases generation of reactive oxygen species and forces neurons to increase their reliance on aerobic glycolysis or alternative energy substrates118. Whether or not ApoE4 affects ketone metabolism in individuals with MCI or AD is unclear. In one AD study, a ketogenic supplement did not raise plasma levels of ketones or improve cognitive outcomes as much in APOE*E4 carriers as it did in non-carriers119. A clinical trial of medium-chain triglycerides in individuals with AD who were specifically selected non-carriers of APOE*E4 showed beneficial cognitive outcomes after 1 month120. However, in transgenic mice expressing human APOE*E4, the presence of ApoE4 did not significantly affect brain ketone uptake in comparison with wild-type controls114.
Polymorphisms in major risk genes for PD, including PINK1 (encoding PTEN-induced putative kinase protein 1) and PRKN (encoding E3 ubiquitin-protein ligase parkin) are closely linked to impaired brain ATP production50,121. Phosphorylation of the endocytic sorting protein Rab10 by leucine-rich repeat serine/threonine-protein kinase 2 (LRRK2) is essential for GLUT4 translocation to the neuronal plasma membrane and is defective in PD patients possessing the LRRK2G2019S mutation122. In HD, axonal transport of mitochondria and glycolytic proteins to the synapse is hindered by mutant huntingtin29. In ALS and FTD, the proteins encoded by risk genes such as TARDBP (encoding TAR DNA-binding protein 43) interfere with mitochondrial function and quality control, thereby compromising ATP production123. Furthermore, the most prominent risk gene for ALS and FTD, C9orf72, encodes part of a complex with guanine nucleotide exchange factor activity that is linked to decreased autophagic lysosome-driven nutrient recycling, leading to frontal and thalamic glucose hypometabolism and aberrant lipogenesis2,124. Indeed, many products of risk genes associated with NDAs interfere with autophagic lysosomal clearance, which has a doubly disabling effect because the metabolic end products of carbohydrates, fats and proteins are then lost to energy generation pathways2.
Therapies based on brain energy rescue
As outlined already, the prevailing notion that impaired brain glucose metabolism in NDAs is simply a consequence of neuronal dysfunction is now being revised. Most notably in AD, the progressive decline in brain glucose uptake and metabolism creates a persistent brain energy gap that contributes to brain cell dysfunction and accumulation of neurotoxic proteins even before the onset of cognitive and neuropsychiatric deficits3 (FIG. 2b). Once glycolysis is impaired and neuronal function starts to decline, the brain energy deficit cannot be corrected by simply increasing blood glucose concentration; indeed, additional dietary glucose aggravates the insulin resistance already commonly present in older people10. Furthermore, brain glucose uptake is driven by neuronal activity, not by circulating glucose levels3. Conversely, ketones and lactate are an alternative brain energy source41, brain uptake of which is driven by their availability in the circulation.
Because no single common pathway causes brain energy deficits in NDAs, brain energy rescue strategies may need to target different metabolic pathways and processes depending on the disease in question3,4,125 (FIG. 3). Some of these strategies focus on a single enzyme, transporter or metabolite, but others are broader (TABLES 1–3). The following discussion first addresses the energetic dimension of mitochondrial dysfunction in NDAs. Strategies that have broader effects such as modulating redox status and ketone-based approaches are described next, then hormone-based approaches to brain energy rescue, followed by a suite of novel strategies currently under exploration. These strategies should all act synergistically with preventive lifestyle changes, such as increased exercise and dietary improvements that help counter insulin resistance126,127 (BOX 3; TABLE 3). For links between mitochondrial dysfunction, oxidative stress and neurodegeneration, see two recent reviews60,78.
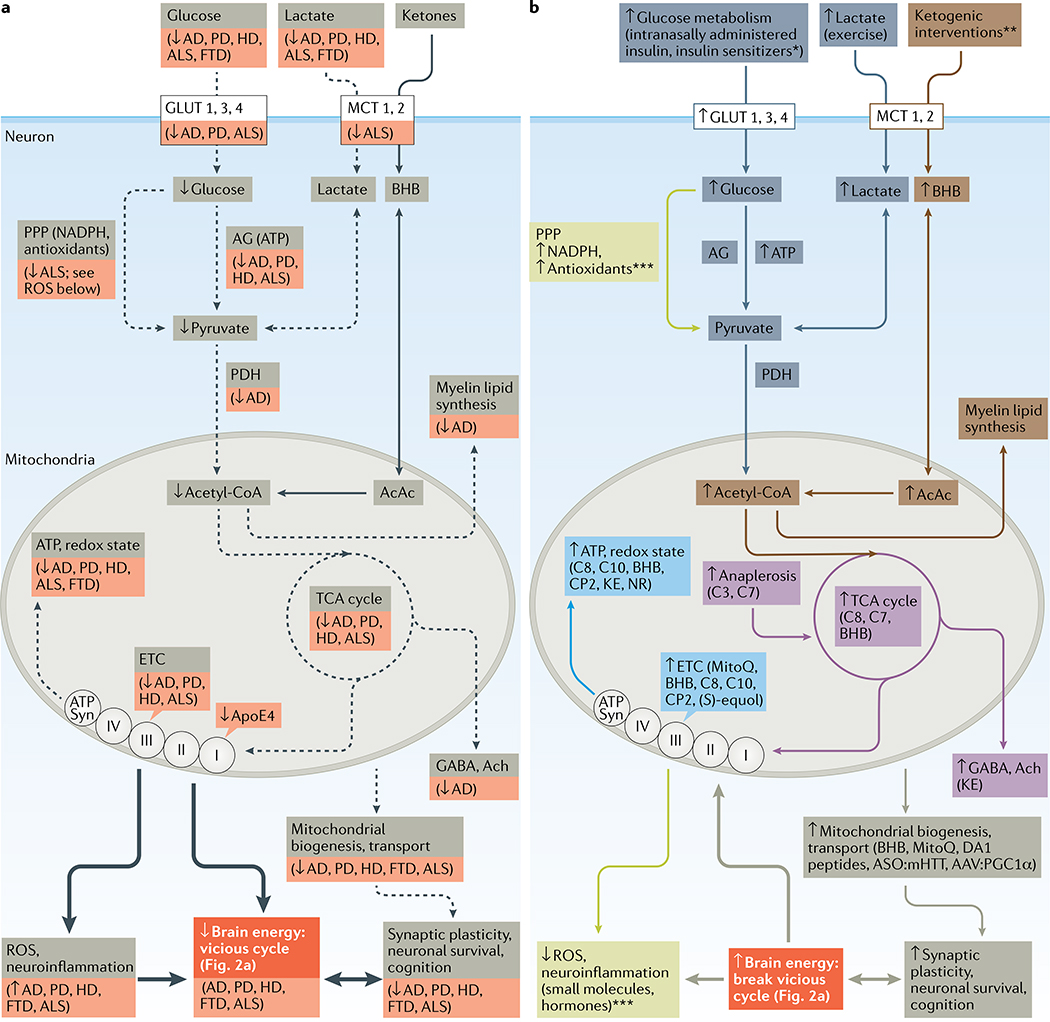
a | Several pathways of brain energy metabolism in neurons are disrupted (shown with dashed black arrows) in neurodegenerative disorders of ageing (specific disorders with declines are shown in the red boxes). Increased production of reactive oxygen species (ROS) and neuroinflammation that negatively affect brain energy levels are shown with a thick black arrow. The combination of impaired ATP production and increased levels of ROS contributes to declining brain function. b | Molecules or potential therapies implicated in brain energy rescue strategies target six broad pathways: ATP and redox state (light blue); brain glucose transport and/or aerobic glycolysis (dark blue; interventions indicated with an asterisk: adiponectin, ghrelin, insulin (GLUT4 only), nicotinamide riboside, dichloroacetate, N-acetylcysteine, oxaloacetate, glucagon-like peptide 1 (GLP1), glucose-dependent insulinotropic polypeptide (GIP), leptin, amylin, metformin, liraglutide and sitagliptin); anaplerosis and the tricarboxylic acid (TCA) cycle (purple; propionic acid (C3), heptanoic acid (C7), octanoic acid (C8), d-β-hydroxybutyrate (BHB), and ketone esters (KE)); mitochondrial transport and biogenesis (olive-grey); ketogenesis (orange; interventions indicated with two asterisks: BHB, C8, decanoic acid (C10) and KE); or protection against ROS and inflammation (light green; interventions indicated with three asterisks: ghrelin, GLP1, GIP, leptin, adiponectin, metformin, AP39, mitochondrial division inhibitor 1 (mdivi-1), MitoQ, BHB, ketogenic diet and KE). Details of the molecules or potential therapies are shown in TABLE 1 (preclinical studies) and TABLES 2,,33 (clinical studies). Complementary interventions such as caloric restriction, ketogenic diet and exercise are not shown. Neurons take up lactate generated by astrocytes and oligodendrocytes (not shown). Medium-chain fatty acids such as decanoic acid and octanoic acid in the circulation can enter astrocytes and produce ketones and acetyl coenzyme A (acetyl-CoA). AAV, adeno-associated virus; AcAc, acetoacetate; acetyl-CoA, acetyl coenzyme A; Ach, acetylcholine; AD, Alzheimer disease; AG, aerobic glycolysis; ALS, amyotrophic lateral sclerosis; ApoE4, E4 isoform of apolipoprotein E; ASO, antisense oligonucleotide; ATP Syn, ATP synthase; DA1, dynamin-related protein antagonist 1; ETC, electron transport chain; FTD, frontotemporal dementia; HD, Huntington disease; MCT, monocarboxylate transporter; mHTT, mutant huntington protein; NR, nicotinamide riboside; PD, Parkinson disease; PGC1α, peroxisome proliferator-activated receptor-γ coactivator 1α; PDH, pyruvate dehydrogenase; PPP, pentose phosphate pathway.
Support of mitochondrial function
Despite continued uncertainty about the extent to which mitochondrial damage is a consequence versus cause of the onset or progression of NDAs65, considerable research focuses on improving mitochondrial function by protecting the electron transport chain, promoting mitochondrial biogenesis and/or reducing oxidative damage to mitochondria125. Assessment of mitochondrial integrity is mostly indirect, but histochemical evidence of decreased cytochrome c activity in post-mortem brain samples from young adult APOE*E4 carriers118 demonstrates that impaired mitochondrial function can be present in presymptomatic individuals at risk of AD.
CP2, a proprietary tricyclic pyrone, improves cognitive and behavioural phenotypes in transgenic AD mice, in part by binding to and partially inhibiting the flavin mononucleotide subunit of complex I. This improves mitochondrial bioenergetics and overall brain energy status, possibly because of increased mitochondrial biogenesis128. CP2 also stimulates AMPK, promotes neuronal resistance to oxidative stress, reduces brain levels of phosphorylated tau and Aβ, improves axonal trafficking and increases levels of brain-derived neurotrophic factor (BDNF) and synaptic proteins in vivo128,129. Controlling the activity of complex I specifically seems to underpin this beneficial effect130 because mutations that inhibit both complex I and complex III or both complex I and complex V are detrimental to brain energetics131.
The mitochondrion-targeted antioxidant MitoQ reduces oxidative stress in mitochondria and is neuroprotective in several NDA models (TABLE 1). Resveratrol stimulates mitochondrial biogenesis through the sirtuin 1 (SIRT1)–AMPK–peroxisome proliferator-activated receptor-γ (PPARγ) coactivator 1α (PGC1α) pathway. Resveratrol also recruits AMPK to enhance autophagy, which removes damaged organelles (including mitochondria) and misfolded proteins and recycles their components, thereby promoting ATP generation2,132. Replacement of old and/or damaged mitochondria starts in the neuronal cell body with new mitochondria being transported along axons to presynaptic terminals15. Both ageing and NDAs increase mitochondrial division in a manner decoupled from the normal fission–fusion cycle, suggesting that inhibiting mitochondrial fragmentation could be beneficial in NDAs133. Quinazolinone and its derivatives such as mitochondrial division inhibitor 1 (mdivi-1) were originally described as selective inhibitors of mitochondrial fission, but their neuroprotective effects in both in vitro and in vivo models of AD, PD and traumatic brain injury are now thought to reflect improved mitochondrial fusion and biogenesis134–136, and possibly better function of complex I.
A pilot clinical study showed that (S)-equol, a selective oestrogen receptor-β agonist, increases cytochrome c oxidase activity in AD137, so treatments that improve mitochondrial function by selective partial inhibition of complex I, target mitochondrial uncoupling proteins or increase mitochondrial biogenesis may result in clinical improvement in NDAs (TABLE 2). Mitochondrial uncoupling proteins could help cells to resist oxidative and metabolic stress98. Low doses of the uncoupling agent dinitrophenol had a neuroprotective effect in preclinical models of AD, PD and HD138. In a mouse model of HD, mitochondrial respiration was improved by bexarotene, a retinoid X receptor agonist and PPARδ activator139.
Table 2 |
Clinical trials reporting improved brain function and/or energetics in NDAs
Disorder | Study details | Results and comments | Ref. |
---|---|---|---|
AD | Single-blind RCT of (S)-equol (n = 15) or placebo (n = 15) for 2 weeks (NCT02142777) | Well tolerated. More participants showed ↑ cytochrome oxidase activity with (S)-equol than with placebo; no cognitive change. First study of a mitochondrial intervention as a direct biomarker of mitochondrial engagement in AD | 137 |
Mild-to-moderate AD and MCI | RCT in individuals with AD (n = 21) or MCI (n = 39) receiving long-acting intranasally administered insulin (20 or 40 IU) or placebo for 4 months (NCT01595646) | Dose-dependent ↑ memory composite score on regular but not long-acting intranasal insulin in ApoE4+ individuals. No change in functional autonomy or executive function | 38 |
AD | RCT of liraglutide (n = 14) or placebo (n = 20) for 26 weeks (NCT01469351) | No change in Aβ load or cognitive scores. ↓ brain glucose uptake over 26 weeks only with placebo. Underpowered for cognitive outcomes. Liraglutide may delay metabolic decline in brain | 194 |
AD or MCI | RCT (n = 20) of metformin (500 mg) or placebo for 8 weeks (NCT01965756) | ↑ Executive function. No change in cerebral blood flow | 182 |
AD | Double-blind RCT of C8 at 20 g per day (n = 77) or placebo (n = 63) for 90 days (NCT00142805) | ADAS-Cog score ↑ by 3.4 points in ApoE4− individuals. Cognitive score varied directly as ketones. ↑ cognition in mild-to-moderate AD | 119 |
Mild-to-moderate AD | Open label study (n = 10) of KD ± C8C10 for 12 weeks (NCT03690193) | ↑ ADAS-Cog score; no cardiovascular safety or other metabolic concerns. First reported clinical use of a KD in AD including medium-chain triglyceride supplementation | 157 |
AD and MCI | RCT of KD (n = 9) or NIA low-fat diet (n = 5) for 12 weeks (NCT02521818) | ↑ composite cognitive score, particularly memory domain, only in adherent participants and only with KD. First reported clinical use of a KD without medium-chain triglyceride supplementation. Feasibility is very challenging but beneficial effects of ketones were clearly present | 162 |
Mild-moderate AD | Open-label study of C8C10 (n = 11) or C8 (n = 6) at 30 g per day for 4 weeks (NCT02709356) | ↑ ketones twofold. Brain ketone uptake ↑ in direct proportion to ↑ ketone and brain glucose utilization. In AD, the brain can utilize additional ketones provided as C8C10 | 270 |
Mild-to-moderate ApoE4− AD | Crossover RCT of medium-chain triglycerides (17.3 g) (n = 24) or placebo (canola oil) (n = 25) for 30 days (ChiCTRIOR6009737) | 2.62-point increase on ADAS-Cog (Chinese version) for medium-chain triglycerides, 2.57-point reduction for placebo. Study restricted to ApoE4− patients. Inverse correlation between cognitive changes and plasma lysophosphatidylcholine species | 120 |
PD | RCT of exenatide at a dosage of 2 mg once per week (n = 31) or placebo (n = 29) for 48 weeks plus a 12-week washout (NCT 01971242) | UPDRS motor subscale at 60 weeks:↑ by 1.0 point for drug and ↓ by 2.1 points for placebo. ↓ motor symptoms | 97 |
PD | RCT of KD (n = 20) or low-fat diet (n = 20) for 8 weeks (ACTRN 12617000027314) | ↑ UPDRS score in both groups, but 41% more for the KD. 86% adherence; tremor ± rigidity intermittently ↑ for KD. First RCT of KD in PD. A KD and low-fat diets are safe in PD | 160 |
HD | Open-label study in individuals with HD (n = 10) and controls (n = 13) receiving triheptanoin at 1 g kg−1 for 1 month (NCT01696708) | MRS: ↑ levels of brain high-energy phosphates including ↑ inorganic phosphate/phosphocreatine during visual stimulation | 271 |
These studies reported statistically significant improvements in primary or secondary end points with novel treatments or drugs approved for other indications and repurposed for treatment of neurodegenerative disorders of ageing (NDAs). The low-fat diet162 was a modified Atkins diet. The three ketogenic diet (KD) trials were all principally feasibility studies not powered for cognitive or metabolic outcomes157,161,163. ↑, increase; ↓, decrease; Aβ, amyloid-β; AD, Alzheimer disease; ADAS-Cog, Alzheimer Disease Assessment Scale — Cognitive Subscale; ApoE4, E4 isoform of apolipoprotein E; C8, octanoic acid; C8C10, octanoic acid plus decanoic acid; HD, Huntington disease; MCI, mild cognitive impairment; MRS, magnetic resonance spectroscopy; NIA, National Institute on Aging; PD, Parkinson disease; RCT, randomized controlled trial; UPDRS; Unified Parkinson Disease Rating Scale.
Redox state, glycolysis and the TCA cycle
The redox state of a cell is typically measured by the ratio of oxidized to reduced nicotinamide adenine dinucleotide (the NAD+ to NADH ratio), which is a non-invasive marker of global brain energy status5,78,140. In general, nutrients and metabolites that raise either blood NAD+ levels or the blood NAD+ to NADH ratio improve the energetic status of the brain141. The NAD+ precursor nicotinamide riboside mitigates cognitive impairment, synaptic degeneration and neuronal death in transgenic mouse models of AD78,98,142. Nicotinamide riboside also improves mitochondrial function in PD neurons and reduces age-related loss of dopaminergic neurons and associated motor deficits in an animal model of PD143. Another potential approach to raising the NAD+ to NADH ratio is dietary supplementation of oxaloacetate144. In several in vitro and animal models of PD, terazosin (a drug approved for treatment of benign prostatic hypertrophy) stimulated phosphoglycerate kinase 1 activity, aerobic glycolysis and ATP production145. Patients taking terazosin to treat other conditions had a decreased risk of developing PD and slower PD progression, so its repurposing to treat PD seems promising.
Supplementation with pyruvate could potentially improve brain energetics by stimulating pyruvate dehydrogenase146,147, a possibility supported by the rescue of defective aerobic glycolysis by pyruvate in HD-derived human induced pluripotent stem cells66. Treatments that improve mitochondrial function have had mixed success in preclinical models of ALS, and these approaches remain largely untested in humans70,74.
Interventions that raise levels of circulating ketones also increase levels of acetyl-CoA, which fuels the TCA cycle independently of aerobic glycolysis. Preclinical studies show that supplementation with BHB, octanoic acid (also known as caprylic acid, an eight-carbon saturated fatty acid), oxaloacetic acid and decanoic acid (also known as capric acid, a ten-carbon saturated fatty acid) as well as a ketogenic diet or caloric restriction (BOX 3) all contribute to increased TCA cycle activity within the brain144,148 (FIG. 3b). In humans, plasma medium-chain fatty acids can be transported into and metabolized by the brain149. TCA cycle intermediates also give rise to bioactive molecules such as the neurotransmitter acetylcholine, whose levels are decreased in AD. These responses are generally reversible and therefore transformation of glutamate into α-ketoglutarate (which enters the TCA cycle) generates ATP in neurons and glia98.
Triheptanoin, a triglyceride of heptanoic acid, delays motor symptoms and is neuroprotective in animal models of ALS74, epilepsy and ischaemic stroke150,151. Triheptanoin also reduces the effort needed to undertake exercise in HD, a beneficial effect associated with increased creatine phosphate concentration in the brain152. Triheptanoin appears to substitute for the branched-chain amino acids that are an endogenous substrate of anaplerosis and levels of which are decreased in HD152,153.
Ketone-based strategies
Several clinical trials show that ketogenic interventions result in cognitive and/or functional improvements in MCI154–156, AD119,120,157–159 and PD160,161. These interventions fall into two categories: ketogenic dietary supplements containing medium-chain triglycerides (either octanoic acid (C8) alone or octanoic acid plus decanoic acid (C8C10)) and the very-low-carbohydrate ketogenic diet (BOX 4; Table 2). In the phase I (ref. 119) and phase II (REF.154) placebo-controlled studies of octanoic acid supplementation and octanoic acid plus decanoic acid supplementation, the interventions lasted from 12 weeks119 to 6 months154, respectively. Two subsequent feasibility studies of ketogenic diets in AD showed increased global cognitive scores in the most adherent patients but did not have control groups157,162.
A recent 6-month study of octanoic acid plus decanoic acid supplementation in MCI showed a direct and statistically significant dose–response relationship between brain uptake of ketones and/or plasma ketone levels and executive function, verbal fluency and language, strongly implying that ketones were directly and mechanistically linked to cognitive improvement via brain energy rescue154. Because of the short half-life of ketones in the body, however, the main challenge with ketogenic interventions is to achieve a sustained therapeutic level of ketosis. In two studies of ketogenic supplements that had a sample size large enough to provide adequate statistical power to assess cognitive outcomes, the 24-h average plasma ketone level was 0.2 mM or less for octanoic acid (REF.119) and 0.4 mM or less for octanoic acid plus decanoic acid (REF.154); these ketone levels would have only partially corrected the brain energy gap in MCI and less so in AD (FIG. 2b; BOX 4). In other clinical trials with a ketogenic diet in AD157,162, MCI155,156 or PD160,161, higher plasma ketone levels were directly related to improved clinical outcomes, but sample size and patient adherence were inadequate to produce definitive evidence of a cognitive benefit.
In individuals with PD, consumption of a ketogenic diet for 8 weeks led to a substantial reduction in urinary problems, pain and fatigue scores versus individuals in a control group consuming a low-fat diet160,161. The ketogenic diet group also showed a trend towards increased motor scores versus the control group. Ketogenic interventions are being explored with some success in animal models of PD163, ALS164 and HD165 (TABLE 1), but randomized, controlled clinical trials of this approach are yet to be reported in these NDAs. A ketogenic diet promotes neurovascular function and metabolic status in mice, along with a healthier intestinal microbiota profile166.
Studies in which a single dose of a ketogenic supplement transiently improved cognition in AD167 and in cognitively normal older people (66 years old)158 suggest that ketones reduce the brain energy gap by bypassing glycolysis and providing acetyl-CoA to enter the TCA cycle directly (FIG. 3b). This interpretation is supported by reports that mild-to-moderate ketosis lasting less than 4 h prevents the autonomic, cognitive and behavioural symptoms of acute insulin-induced hypoglycemia in T1D168. In turn, mild ketosis probably spares some glucose to be used by pathways other than glycolysis and oxidative phosphorylation41, including the pentose phosphate pathway, which generates NADPH, but also anaplerosis for the TCA cycle (FIG. 3). Whether glucose sparing is central to the therapeutic effect of ketone supplementation in NDAs remains to be determined.
Metabolism of ketones in the brain not only generates fuel but also provides an important substrate for the synthesis of brain lipids, including myelin40. Ketones are also substrates for post-translational protein modification and activate cell signalling125. The density and activation of HCAR2 are increased in the substantia nigra in PD169 and lactate is neuroprotective in an animal model of PD170. Reducing neuronal hyperexcitability by raising GABAergic tone may contribute to the efficacy of ketone supplementation in individuals with NDAs as it does in epilepsy13,148,171.
Disease modification — that is, retardation or reversal of neuropathology — is a crucial goal in the treatment of NDAs. Studies in transgenic AD mice show that ketones decrease Aβ deposition in the brain172 and reduce the excitatory effect of Aβ42 on neurons146. These findings from preclinical studies were confirmed in a pilot clinical study in MCI156, suggesting that in addition to providing an alternative brain energy substrate that bypasses the brain glucose deficit, ketogenic interventions could potentially improve cognitive outcomes in MCI and AD by slowing pathological processes that result in Aβ accumulation and damage.
High-fat diets are commonly perceived to increase the risk of cardiovascular disease, so it is important to consider their potential risks. Very-high-fat ketogenic diets have been assessed in five clinical trials of durations ranging from 6 to 12 weeks. Three of these trials were conducted in participants with MCI or AD155,157,162 and the other two were conducted in participants with PD160,161. In none of these trials were the levels of common biomarkers of cardiovascular risk increased, including body weight or plasma LDL cholesterol or triglycerides. However, no standard definition of a ketogenic diet exists and some ‘high-fat’ diets used experimentally (and possibly also clinically) might adversely affect cardiovascular health outcomes (in particular during very long-term use) because they contain an excess of refined carbohydrate. In clinical trials, ketogenic medium-chain triglycerides were not associated with increased cardiovascular or metabolic risk (TABLE 2); indeed, like the very-high-fat ketogenic diet, medium-chain triglycerides are commonly used to treat obesity and T2D, which are themselves risk factors for NDAs.
Increasing insulin sensitivity
Brain energy homeostasis is closely linked to peripheral insulin sensitivity, both of which depend on the balance between global energy intake and energy use. The two main determinants of peripheral insulin sensitivity are exercise and intake of refined carbohydrate173. When lifestyle changes are ineffective or difficult to implement, peripheral injections of insulin are commonly used to treat T2D, but the challenge is to avoid episodes of hypoglycaemia and exacerbation of insulin resistance, both of which increase morbidity168.
Intranasally administered insulin and insulin sensitizers
Intranasally administered insulin and intranasal insulin sensitizers could potentially mitigate the deleterious effects of insulin resistance and a glucose deficit (FIG. 3; TABLES 1–3). Intranasally administered insulin enters the brain directly via olfactory neurons, which enables treatment of central nervous system insulin resistance while minimizing systemic hypoglycaemia. Short-term studies show that intranasally administered insulin enhances cognitive function in healthy young adults, individuals with MCI and individuals with mild-to-moderate AD, in part by stimulating brain glucose metabolism38,174. Little or no intranasally administered insulin enters the peripheral circulation, but intranasal insulin delivery still needs to be optimized to achieve a more consistent increase in brain insulin levels before its efficacy for cognitive improvement can fully be assessed38.
Metformin decreases hepatic glucose production, which increases insulin sensitivity in T2D, so it is being investigated for therapeutic use in NDAs175. Metformin reduces neuropathology and corrects memory deficit in AD mice176 and normalizes cortical network disruption and anxious behaviour in HD mice177. Within the brain, metformin also stimulates autophagy, improves synaptic function and reduces neuroinflammation — effects that mimic those of caloric restriction and exercise2,178. Metformin suppresses coupling of the redox and proton transfer domains of complex I, but its overall mechanism of action remains unclear179,180. Recent data suggest that growth/differentiation factor 15 (GDF15) acting via GDNF family receptor-α-like (GFRAL) may mediate the influence of metformin on energy metabolism, suggesting that they could become novel therapeutic targets for safely combating brain energetic deficits associated with NDAs181. Indeed, metformin is potentially protective against cognitive decline in MCI or AD182,183 and cognitive impairment due to stroke184. However, metformin may exacerbate Aβ accumulation185 and has adverse effects linked to overproduction of AMPK and vitamin B12 deficiency132. In addition, studies are needed to further clarify the putative utility of metformin for restoring energetic status in NDAs175,186.
Impaired brain glucose uptake and insulin receptor desensitization could potentially be corrected by targeting nuclear hormone receptors that activate insulin-regulated and IGF-regulated pathways. For example, thiazolidinediones are PPARγ agonists that potentially reduce brain insulin resistance associated with AD and other NDAs36. However, PPARγ agonists, including pioglitazone and rosiglitazone, have produced no cognitive benefit in clinical trials in AD. Inhibitors of sodium–glucose co-transporter 2 such as dapagliflozin increase glucose excretion, improve cardiovascular outcomes and reduce mortality in T2D187. In addition, these agents induce mild ketonaemia188, suggesting that they should be tested in NDAs, perhaps in combination with ketogenic interventions.
Incretin hormones
GLP1 receptor agonists such as liraglutide are approved to treat insulin resistance, obesity and T2D189,190. On the basis of encouraging findings in animal models of AD, PD, HD and ALS (TABLE 1; Supplementary Table 2), they are also being assessed for treatment of NDAs97,191,192. For example, liraglutide and exenatide reduced neuropathology, neuroinflammation and microvascular pathology and improved cognitive outcomes in a transgenic mouse model of AD192 (TABLE 2). A GLP1 receptor agonist reduced Aβ accumulation and reduced mitochondrial proapoptotic signalling, while increasing antiapoptotic signalling and BDNF levels192. Semaglutide, a long-acting GLP1 analogue, was more neuroprotective than liraglutide in an animal model of PD, a beneficial effect related to improved mitochondrial function and lower oxidative stress, apoptosis and neuroinflammation97. Inhibitors of GLP1 breakdown are now in clinical trials to treat NDAs193.
In mild-to-moderate AD, liraglutide administration for 6 months attenuated the decline in brain glucose uptake but had no effect on brain Aβ load or cognitive outcomes194. Exenatide administration reduced symptoms of PD in a phase II trial195. Interestingly, metformin might exert its actions partly via GLP1 (REF.36). Some dipeptidyl peptidase 4 (DPP4) inhibitors (gliptins) approved to treat T2D are known to prolong the activation of GLP1 (REF.196); one such agent, sitagliptin, improved cognition in older individuals with diabetes with or without AD197.
GIP agonists have been shown to have benefits similar to those of GLP1 agonists in mouse models of PD198,199. GIP agonists closely mimic GLP1 agonists in animal models of AD200,201. Dual agonists of both GLP1 and GIP were more effective in rodent models of PD than GLP1 agonists alone200,201. A triple agonist of GLP1, GIP and glucagon receptors had broad neuroprotective activity in a mouse model of AD202. Clinical data are eagerly awaited for these multitargeted agents.
Ghrelin
Ghrelin is neuroprotective and improves cognition in animal models of AD, PD and HD203–205 (Supplementary Table 2). The beneficial effects of ghrelin involve promotion of neuronal glucose uptake, increased expression of uncoupling protein 2, improved mitochondrial function and enhanced mitophagy88,205. AMPK activation in dopaminergic neurons of the substantia nigra may also contribute to the beneficial effects of ghrelin in PD: AMPK activates PGC1α to induce mitochondrial biogenesis and increase ATP production, as well as stimulating autophagy to eliminate α-synuclein2,205,206. Since ghrelin counters gastrointestinal dysfunction in PD, relamorelin, a centrally penetrant and selective agonist of ghrelin receptor (also known as growth hormone secretagogue receptor type 1), is being assessed to treat constipation in PD and T2D. The effects of relamorelin on motor function, neuronal survival and bioenergetics are also being evaluated in PD207.
Leptin and adiponectin
Leptin promotes mitochondrial function, has neuroprotective properties and mitigates the neurotoxic effects of Aβ accumulation in animal models of AD and PD86,87,208. Synergistic beneficial effects on mitochondrial function have been reported for leptin in combination with PPARα agonists209. The risk of ALS is reduced in individuals with T2D, so it is interesting that knocking out the gene encoding leptin (Lep), which suppresses appetite in a mouse model of ALS, slowed the progression of ALS symptoms while decreasing energy expenditure and increasing body weight210. This suggests that leptin antagonists should be evaluated as a potential treatment in ALS. Data on adiponectin are currently limited to mouse models of AD in which adiponectin agonists had neuroprotective properties associated with reduced loads of Aβ and phosphorylated tau and improved cognitive performance — benefits that were related to increased glucose uptake in the hippocampus and, possibly, to increased insulin sensitivity86,211.
Amylin
The status of amylin as a potential target for treating NDAs is controversial92 because native amylin itself is amyloidogenic and aggregated amylin has proapoptotic and neuroinflammatory effects and may seed Aβ aggregation91,93. Moreover, Aβ binds to amylin receptors, and its neurotoxic actions and interference with cognition were blunted by an amylin antagonist91,212. Nevertheless, amylin itself could have potential beneficial properties as a leptin sensitizer, including leptin resistance in AD213. The satiety-stimulating effects of amylin may help to control weight gain as well as increase insulin sensitivity and brain glucose metabolism. Treatment with human amylin or a non-aggregating amylin analogue, pramlintide, has shown both cognitive benefits and reductions in Aβ pathology in animal models of AD214. Amylin and pramlintide also promote Aβ efflux from the brain, regulate synaptic proteins, reduce oxidative stress and inflammation and improve mitochondrial function214,215. Pramlintide protects against the neurotoxic and memory-disrupting actions of Aβ91. Whether amylin-related mechanisms can be harnessed in the treatment of AD and other NDAs remains to be seen.
Restoration of downstream signalling
An important fate of glucose distinct from its use as an energy substrate is its utilization to generate O-linked β-N-acetylglucosamine (O-GlcNAc), which is post-translationally and reversibly added to serine and threonine residues of numerous proteins. O-GlcNAcylation occurs via O-GlcNAc transferase, whereas O-GlcNAcase removes O-GlcNAc residues: both of these enzymes are therapeutically targetable in NDAs216. O-GlcNAcylation is important for axonal stability and synaptic plasticity and for the local and dynamic coupling of glucose utilization to glycolysis and mitochondrial function at both presynaptic and postsynaptic sites216. In primary cell culture models of PD, O-GlcNAcylation of α-synuclein reduced its aggregation and toxicity217. In addition, in transgenic mouse models of AD, downregulation of O-GlcNAcylation is implicated in the production of Aβ and phosphorylated tau218. Novel therapeutic agents that aim to restore O-GlcNAcylation are currently under investigation in experimental models of NDAs216–218.
Epigenetic interventions
In addition to driving the TCA cycle, acetyl-CoA is a precursor of brain lipids and a substrate for generation of acetylcholine. Acetyl-CoA is also the source of the acetyl moiety used to acetylate several enzymes that modulate glycolysis, gluconeogenesis and the TCA cycle, tau (acetylation of which promotes its aggregation) and histones219. Acetylation is a core component of histone modification, which regulates gene expression, so cellular energetics are affected by the availability of acetyl-CoA for histone acetyltransferases220,221. Accordingly, acetyl-CoA provides a direct link between brain energy balance and the epigenetic control of gene expression — a link reinforced by other components of the TCA cycle, including the intermediates succinate and citrate219. Furthermore, increased activity of the deacetylating enzyme sirtuin 1 promotes mitochondrial function98 and is implicated in the positive influence of the exercise-induced increase in brain lactate levels on cognition222. Specific histone deacetylases in the hippocampus may contribute to the increased resilience to stress mediated by lactate in mice223.
Short-chain fatty acids produced by the intestinal microbiota also influence the activity of histone deacetylases44. For example, BHB modulates the β-hydroxybutyrylation of histones at lysine residues, which couples metabolic status to the control of gene expression224. Post-translational histone modifications and DNA methylation influence bioenergetic processes that are disrupted in NDAs, so pharmacological modulation of these epigenetic mechanisms could potentially improve brain energy status in NDAs220,221.
RNA-based and DNA-based therapies
Diverse techniques are being developed to alter the level of mRNA encoding proteins that are anomalously expressed in NDAs. These strategies could suppress neurotoxic effects or compensate for a loss of physiological function, thereby improving the energetic status of the brain. Targeting specific classes of microRNAs and long non-coding RNAs that control the translation of dysregulated glycolytic and ATP-generating mitochondrial proteins should also be feasible.
Recent clinical success with oligonucleotide-based therapies in central nervous system disorders such as spinal muscular atrophy225,226 and advances in the manipulation of oligonucleotides, such as antagomirs and locked nucleic acids, make this approach increasingly relevant to NDAs, even for hitherto ‘undruggable’ targets227–229. In HD, clinical trials of antisense oligomers directed against mutant HTT mRNA are under way to prevent its interference with mitochondrial transport and function230. In addition, allele-specific strategies that specifically decrease mutant HTT mRNA while preserving normal HTT mRNA are under investigation. Some of these interventions use zinc-finger nucleases (which act as transcription factors), whereas others rely on small molecules that promote clearance specifically of the mutant proteins231. Antisense oligomers and similar approaches could also be used to target genes containing mutations that disrupt mitochondrial energetics in PD and ALS with FTD232,233 (Supplementary Table 1).
Oligonucleotides and small interfering RNAs that modulate pre-mRNA splicing or neutralize mRNA-directed microRNAs preferentially increase the expression of the intact allele of energy-generating genes downregulated in NDAs226,234. A more direct mode of gene therapy that aims to restore abnormally low or absent gene expression is to transfer copies of the intact gene into the brain using an adeno-associated virus vector. For example, the approved gene therapy onasemnogene abeparvovec uses an adeno-associated virus vector to deliver intact SMN1 gene copies to motor neurons to treat spinal muscular atrophy235. An adeno-associated virus vector loaded with the human GLUT1 promoter injected directly into the brains of GLUT1-deficient mice led to robust GLUT1 expression in corticolimbic regions, together with increased cerebrospinal fluid glucose levels and improved motor function236. A similar strategy that targeted dysfunctional PGC1α helped to restore mitochondrial function in dopaminergic pathways in mouse models of PD237.
DNA and RNA editing might also become options for the treatment of NDAs, for example, using zinc-finger nucleases or CRISPR–Cas9 technologies. One specific approach to improve brain energetics in AD is the conversion of the ApoE4 isoform into ApoE3 as shown in a neuronal cell line234,238,239. Gene editing of APOE has not yet been achieved in vivo but progress is rapid in this field and a broad range of options for improving glucose metabolism and other abnormalities associated with AD based on neutralization of ApoE4 are being investigated240.
Mitochondrial dysfunction and other disturbances associated with HD may also be linked to excessive translation of mRNAs and overproduction of proteins resulting from inactivation of the eukaryotic translation initiation factor 4E (EIF4E) translational repressor complex. Rapamycin and other repressors of this complex or its components should therefore be assessed for restoration of mitochondrial energetics and integrity in NDAs241. Another approach could be so-called autophagy-targeting chimaeras to specifically target and eliminate fragmented mitochondria. In a proof-of-concept study, this approach restored the generation of functional mitochondria and ATP levels in fibroblasts from patients with Down syndrome, who invariably develop AD242.
Photobiomodulation therapy
Low wavelengths of light penetrate brain tissue to a considerable depth, and transcranial (intracranial, intra-aural or intranasal) application of near-infrared light is being studied as a treatment for various brain disorders243,244. The mechanisms underlying the positive effects of photobiomodulation therapy await further elucidation, but increased brain perfusion, energy availability and oxygen supply have been proposed, in addition to neuronal actions implicating light-absorbing cytochrome c and increased ATP production243–245. The use of photobiomodulation to improve brain energetics has received initial support from small-scale clinical trials243,244,246, but rigorous controlled studies with larger sample sizes are needed. An alternative strategy to improve the energy status of the brain could be the use of non-invasive light and/or auditory stimulation regimes. In a mouse model of AD, this approach reduced pathology and reduced the levels of neurotoxic proteins, in part by favouring neurovascular coupling and, by inference, brain energetic status247.
Conclusions and outlook
Impaired brain energy metabolism is now recognized in NDAs and, at least in AD, clearly precedes the onset of clinical symptoms. The metabolic defects occur at multiple levels, including reduced neuronal glucose uptake, impaired glycolysis and suboptimal function of the TCA cycle, all of which adversely impact axonal transport, mitochondrial function and ATP production (FIG. 3a). The multiple faces of brain glucose hypometabolism present challenges for drug development in NDAs. Indeed, in view of the multiple brain energetic pathways affected, a fundamental question is whether pharmacological approaches that target a single enzyme, receptor or protein could ever be truly clinically effective. By analogy to other multifactorial disorders associated with an increased risk of NDAs, such as depression and T2D248, where multimodal interventions are the most effective, the same could turn out to be true for brain energetic rescue in NDAs. An example would be use of agents that simultaneously clear aggregated toxic proteins and/or suppress reactive oxygen species and neuroinflammation2,249,250.
The efficacy of a multimodal approach depends on a better understanding of the cause-and-effect relationships between the brain glucose deficit and pathophysiological processes1,2,103. In any event, attempting to promote energetically expensive processes such as microglial clearance, synaptic remodelling, myelin regeneration or axonal transport seems questionable unless the brain has adequate energy resources to fuel the additional work. Therefore, optimization of brain energetics should become a core component of future clinical trials of potential therapies for NDAs, irrespective of their mechanisms of action, because unless the brain energy gap can be reduced (FIG. 3), potential benefits of new medications may well be missed. Furthermore, a multipronged approach embracing both targeted pharmaceutical treatments and broad improvement in diet and lifestyle is emerging as a viable way to improve prognosis and relieve clinical symptoms of NDAs2,5,249,251. Insights could also be obtained by considering brain energetics in other neurological diseases (BOX 5).
Preclinical studies have demonstrated that brain energy rescue can delay the onset and/or progression of NDAs at two levels: first, by improving neuronal integrity, synaptic plasticity and neuronal–glial interactions linked to cognitive and functional deficits; and second, by disease modification, at least for metformin176 and certain ketone-based interventions146,172. The benefit of ketone-based interventions in NDAs resembles that seen in other brain disorders, including schizophrenia84 and epilepsy4,252. It is interesting that ketones and the other traditional ‘black sheep’ of energy metabolism — lactate — which have complementary roles both as signalling molecules and as neuroprotective molecules in brain energetics, have much to offer in developing brain energetic rescue strategies in NDAs3,8,253,254. Hormone-based interventions that modulate appetite and energy expenditure should also have both an early preventive influence by delaying the onset of neuropathology and symptoms and a later benefit by delaying further decline in cognitive function or other functional outcomes. Genetic, epigenetic and other novel strategies are also showing promise for improving brain energetics in NDAs.
Being able to link clinical symptomatic relief to a clinical readout or a measurable biomarker (that is, imaging, metabolite or hormone76,255) would accelerate validation of clinical effectiveness and product development. In addition, an ideal biomarker would be able to predict disease modification by an intervention. Ketone PET links brain energy status to cognitive outcomes in MCI (BOX 4), but it does not demonstrate whether disease modification occurred. Given the probable need for a long-term multimodal strategy including lifestyle intervention, successful adherence and retention in future clinical trials are ultimately likely to depend on the intervention being personalized; for example, exercise or insulin sensitizers only for those who are insulin resistant256.
In conclusion, just as normal neurocognitive development during infancy depends on adequate brain energy supply, the maintenance of cognitive performance and cerebral function during ageing is contingent on the brain continuing to successfully meet its energy needs. Guaranteeing the energy status of the brain should become a cornerstone for trials attempting to delay the onset and progression of NDAs. The observations discussed herein should help us move towards this important goal.
Acknowledgements
This article is based on the proceedings of a small, focused symposium organized by M.J.M. and supported by an unrestricted grant from Advances in Neuroscience for Medical Innovation, which is affiliated with the Institut de Recherche Servier. S.C.C. is supported by the Alzheimer’s Association (USA), the Canadian Institutes of Health Research, the Fonds de Recherche du Québec – Santé, the Natural Sciences and Engineering Research Council of Canada and Nestlé, and thanks V. St-Pierre, M. Fortier, A. Castellano, É. Myette-Côté, E. Croteau, M. Roy, M.-C. Morin and C. Vandenberghe in particular for outstanding help. M.J.M. thanks J.-M. Rivet for help with preparation of the original graphic artwork and M. Gaillot and her team for the ordering and provision of PDF documents consulted in the preparation of the manuscript. R.H.S. is supported by grants P30 AG035982, R01 AG060733 and R01 AG061194 from the US National Institutes of Health (NIH). E.T. is supported by the NIH National Institute on Aging (grant RF1AG55549) and National Institute of Neurological Disorders and Stroke (grants R01NS107265 and RO1AG062135). Z.B.A. is supported by a Senior Research Fellowship from the National Health and Medical Research Council of Australia (APP1154974). P.I.M. is supported by funding from the Alzheimer’s Association (NIRG-13-282387), European Regional Development Fund funds through the operational programme ‘Thematic Factors of Competitiveness’ and by the Portuguese Foundation for Science and Technology (grants PEst-C/SAU/LA0001/2013-2014 and UIDB/04539/2020). G.C. is supported by the NIH (grants 1R15AG050292 and 1R21AG064479). R.D.B. is supported by the National Institute on Aging (grants R37AG053589, R01AG057931 and P01-AG026572). J.H.J. is supported by grants from the Canadian Institutes of Health Research, Alberta Prion Research Institute, the Alzheimer’s Society of Alberta and Northwest Territories and the University Hospital Foundation (Edmonton, AB, Canada). L.H.B. holds grants from Nasjonalforeningen-Demensforbundet, Norway. A.E. is supported by the Swiss National Science Foundation (grant 31003A-179294). O.K. is supported by the Deutsche Forschungsgemeinschaft (grant CRC 1134, B02). M.P.M. is supported by the UK Medical Research Council (MC U105663142) and by a Wellcome Trust Investigator Award (110159/Z/15/Z). F.S. is funded by the European Research Council under the European Union’s Horizon 2020 research and innovation programme (ADG grant agreement no. 834317). W.F.M. is supported by the European Research Council (ADG 666053 and VW 93046). A.P. is supported by the Deutsche Forschungsgemeinschaft (PR1527/5-1) and the German Federal Ministry of Education and Research (AZ.031A318 and 031L0211). K.A.N. is supported by the European Research Council (ADG 671048) and the Adelson Medical Research Foundation. C.M. was supported by the Research Council of Norway: 262647/F20.
Competing interests
S.C.C. declares that he has consulted for and has received honoraria, test products and/or research funding from Abitec, Accera, Bulletproof, Nestlé and Servier, is the founder of Senotec and is co-inventor on a patent for a medium chain triglyceride formulation. M.J.M. declares that he is a full-time employee of Servier and has no other interests to declare. M.P.M. declares that he holds patents related to therapies targeted at decreasing oxidative damage to mitochondria. G.C. declares that she holds a patent related to compositions and methods for treating cognitive deficits using amylin and other hormones. A.E. declares that she has received honoraria, test products and/or research funding from Schwabe and Vifor. F.S. declares that he has consulted for Servier and TEVA. R.D.B. declares that she holds patents for therapeutics targeting Alzheimer disease and neurodegenerative disorders of ageing and is the founder of NeuTherapeutics. E.T. declares that she holds a patent related to compositions and methods for treating cognitive deficit using complex I inhibitors. All other authors declare no competing interests.
Footnotes
Publisher’s note
Springer Nature remains neutral with regard to jurisdictional claims in published maps and institutional affiliations.
Supplementary information
Supplementary information is available for this paper at https://doi.org/10.1038/s41573-020-0072-x.
References
Full text links
Read article at publisher's site: https://doi.org/10.1038/s41573-020-0072-x
Read article for free, from open access legal sources, via Unpaywall:
https://www.duo.uio.no/bitstream/10852/85497/1/Cunnane%2bet%2bal.%2bNRDD%2baccepted.pdf
Citations & impact
Impact metrics
Citations of article over time
Alternative metrics
Smart citations by scite.ai
Explore citation contexts and check if this article has been
supported or disputed.
https://scite.ai/reports/10.1038/s41573-020-0072-x
Article citations
Risk of Alzheimer's disease and genetically predicted levels of 1400 plasma metabolites: a Mendelian randomization study.
Sci Rep, 14(1):26078, 30 Oct 2024
Cited by: 0 articles | PMID: 39478193 | PMCID: PMC11525545
Brain Metabolism in Health and Neurodegeneration: The Interplay Among Neurons and Astrocytes.
Cells, 13(20):1714, 17 Oct 2024
Cited by: 0 articles | PMID: 39451233 | PMCID: PMC11506225
Review Free full text in Europe PMC
Mapping Age Differences in Brain Energy Metabolites and Metabolic Markers of Cellular Membrane Production and Degradation With <sup>31</sup>P Magnetic Resonance Spectroscopy.
Hum Brain Mapp, 45(14):e70039, 01 Oct 2024
Cited by: 0 articles | PMID: 39391993 | PMCID: PMC11467684
Extremely Low-Frequency Electromagnetic Field (ELF-EMF) Increases Mitochondrial Electron Transport Chain Activities and Ameliorates Depressive Behaviors in Mice.
Int J Mol Sci, 25(20):11315, 21 Oct 2024
Cited by: 0 articles | PMID: 39457098 | PMCID: PMC11508854
Therapeutic Effects of Metformin on Central Nervous System Diseases: A Focus on Protection of Neurovascular Unit.
Pharm Res, 41(10):1907-1920, 07 Oct 2024
Cited by: 0 articles | PMID: 39375240
Review
Go to all (327) article citations
Data
Data behind the article
This data has been text mined from the article, or deposited into data resources.
BioStudies: supplemental material and supporting data
Clinical Trials (Showing 11 of 11)
- (2 citations) ClinicalTrials.gov - NCT01965756
- (1 citation) ClinicalTrials.gov - NCT02709356
- (1 citation) ClinicalTrials.gov - NCT03690193
- (1 citation) ClinicalTrials.gov - NCT02551419
- (1 citation) ClinicalTrials.gov - NCT02142777
- (1 citation) ClinicalTrials.gov - NCT01595646
- (1 citation) ClinicalTrials.gov - NCT02521818
- (1 citation) ClinicalTrials.gov - NCT01696708
- (1 citation) ClinicalTrials.gov - NCT01469351
- (1 citation) ClinicalTrials.gov - NCT00142805
- (1 citation) ClinicalTrials.gov - NCT00777010
Show less
Similar Articles
To arrive at the top five similar articles we use a word-weighted algorithm to compare words from the Title and Abstract of each citation.
Mitochondrial biogenesis: pharmacological approaches.
Curr Pharm Des, 20(35):5507-5509, 01 Jan 2014
Cited by: 171 articles | PMID: 24606795
Aberrant Energy Metabolism in Alzheimer's Disease.
J Transl Int Med, 10(3):197-206, 24 Sep 2022
Cited by: 19 articles | PMID: 36776238 | PMCID: PMC9901551
Balancing glycolysis and mitochondrial OXPHOS: lessons from the hematopoietic system and exercising muscles.
Mitochondrion, 19 Pt A:3-7, 28 Sep 2014
Cited by: 13 articles | PMID: 25264322
Review
The cellular and compartmental profile of mouse retinal glycolysis, tricarboxylic acid cycle, oxidative phosphorylation, and ~P transferring kinases.
Mol Vis, 22:847-885, 23 Jul 2016
Cited by: 49 articles | PMID: 27499608 | PMCID: PMC4961465
Funding
Funders who supported this work.
European Research Council (2)
Grant ID: 671048
Grant ID: 666053
Medical Research Council (2)
Mitochondrial oxidative damage and human diseases
Dr Michael Murphy, MRC Centre Cambridge
Grant ID: MC_U105663142
Mitochondrial oxidative damage and human diseases
Dr Michael Murphy, University of Cambridge
Grant ID: MC_UU_00015/3
NIA NIH HHS (11)
Grant ID: R01 AG060733
Grant ID: R01 AG061194
Grant ID: RF1 AG062135
Grant ID: R01 AG057931
Grant ID: R37 AG053589
Grant ID: P01 AG026572
Grant ID: P30 AG035982
Grant ID: R15 AG050292
Grant ID: RF1 AG059093
Grant ID: R21 AG064479
Grant ID: RF1 AG055549
NINDS NIH HHS (2)
Grant ID: R01 NS107265
Grant ID: UH3 NS113776
Swiss National Science Foundation (1)
Grant ID: 179294
Wellcome Trust (1)
Exploring mitochondrial metabolism in health and disease using targeted biological chemistry.
Dr Michael Murphy, MRC Mitochondrial Biology Unit
Grant ID: 110159/Z/15/Z